DOI:
10.1039/D4NJ01394H
(Perspective)
New J. Chem., 2024,
48, 10341-10346
Bioethanol fermentation in the presence of ionic liquids: mini review
Received
25th March 2024
, Accepted 11th April 2024
First published on 29th May 2024
Abstract
Ionic liquids are known as efficient pretreatment solvents for cellulosic biomass, but typical cellulose-dissolving ionic liquids are toxic to microorganisms, hindering the fermentation process for bioethanol production. Although the ionic liquids can be removed by washing after pretreatment, it negatively impacts the energy balance. Therefore, successive/simultaneous biomass pretreatment, hydrolysis, and fermentation processes with low-toxicity ionic liquids are desired. Herein, especially for the beginners and students, the toxicity of ionic liquids and their mechanisms by which the microorganisms are destroyed are simply discussed. Furthermore, two currently evolving solutions, low-toxicity ionic liquids and ionic liquid-resistant microorganisms for bioethanol production, are discussed. To the best of my knowledge, this is the first review specifically focusing on bioethanol fermentation in ionic liquid solutions, whereas there are many reports on cellulose dissolution.
Lignocellulose as a resource of bioethanol
The global demand for energy is increasing, and concerns about climate change and the instability of oil resources have led to renewed interest in alternative energy sources to replace fossil fuels. As a result, many countries have been focusing on sustainable and renewable biofuels.1,2 Currently, corn starch, sugarcane, and beet monosaccharides are directly converted to ethanol, but these edible sugars compete with food and feed. Therefore, lignocellulose, a major component of plant cell walls, is recognized as one of the most promising resources for ethanol production.3 Specifically, cellulose and hemicellulose in lignocellulose are the resources of bioethanol. Although hemicellulose is relatively easy to convert, cellulose is not; it is one of the most chemically and physically robust and recalcitrant natural polymers.3,4
Significance of bioethanol fermentation in the presence of ionic liquids
Typical ionic liquids, which are liquid salts below 100 °C,5–14 are toxic to microorganisms.15–20 Then, why is it necessary to conduct microbial fermentation in the presence of ionic liquids? To figure this out, we first look at the overall picture of the bioethanol production process (Fig. 1).
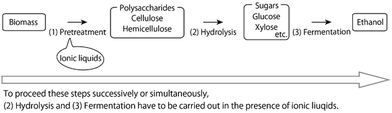 |
| Fig. 1 Overview of the bioethanol production process and the significance of fermentation in the presence of ionic liquids. | |
Polysaccharides can be converted to ethanol by the following three processes: (1) Pretreatment with ionic liquids to improve enzyme accessibility of the polysaccharides; (2) enzymatic hydrolysis of the polysaccharides to sugars, such as glucose and xylose; (3) ethanol fermentation by microorganisms, such as yeasts.
Energy cost is one of the most important factors in the production of ethanol as a fuel. It is essential to reduce energy costs during production and thus maximize the energy obtained from ethanol. Although there are several cellulose solvents such as LiCl/N,N-dimethylacetamide and ethylene diamine/salt,21–23 they do not efficiently dissolve cellulose at room temperature and ambient pressure. The high temperature and/or pressure are very energy intensive, hindering the practical industrialization of bioethanol.
On the other hand, ionic liquids, especially those containing carboxylate, dialkylphosphate, or alkylphosphonate anions, efficiently dissolve cellulose/biomass even at room temperature.24–31 (Chloride-type ionic liquids are less effective in pretreatment.32,33) Therefore, ionic liquids are recognized as the most promising solvents for bioethanol production. Recently, ionic liquid-like onium salts with hydroxide anions were also reported as cellulose/biomass solvents working at room temperature.34–36 Despite these advantages,5–13 using ionic liquids alone is not sufficient for commercialization because ethanol has a low combustion energy density: around 60% of gasoline per volume. Further reduction of the energy cost is necessary and the processes (1)–(3) must be carried out in succession in the same reaction pot (called one-pot ethanol production), indicating that hydrolysis and fermentation must be carried out in the presence of ionic liquids used for pretreatment. However, typical cellulose-dissolving ionic liquids are toxic to microorganisms, which can hinder the fermentation process.15–20 There are several good reviews on biomass pretreatment with ionic liquids6,10,28,37,38 and hydrolysis in the presence of ionic liquids.39,40 Comparatively, there is less focus on fermentation in the presence of ionic liquids, which is reviewed here as a mini-review.
Microbial toxicity of ionic liquids
The toxicity of ionic liquids strongly depends on the alkyl chain length of the cation (Table 1).19,41–44 Long alkyl chains of the cation (e.g., 1-methyl-3-octylimidzaolium acetate: [C8mim]OAc; Fig. 2) enter the cell membrane and eventually destroy it (Fig. 3).41–44 The mechanism of alkyl chain entry is as follows: (i) A positively charged cation comes into close contact with a negatively charged phosphate group of the cell membrane. (ii) The alkyl group of the cation interacts with the acyl group (long-alkyl chain) of the cell membrane by hydrophobic interactions and enters the cell membrane. On the other hand, short alkyl chains of the cation (e.g., [C2mim]OAc, the most well-known pretreatment solvent for biomass) are relatively less toxic than their long counterparts, but are still more toxic than common organic solvents.45,46 For example, a commonly used indicator of toxicity, half maximal effective concentration (EC50), is defined as the concentration of ionic liquids at which the growth of microorganisms is halved compared to that without ionic liquids. The EC50 values of [C2mim]OAc, dimethyl sulfoxide, and ethanol against Escherichia coli (E. coli) are 9, 91, and 17 g L−1, respectively.45 The toxicity is assumed to be derived from the entry of cations into the cell via transporters on the cell membrane.47 The cations alter the mitochondrial membrane potential, leading to apoptosis (cell death).
Table 1 The alkyl chain length of the cations and the minimum growth inhibition concentration of yeast (Saccharomyces cerevisiae). The minimum growth inhibitory concentration is the lowest concentration that prevents the growth; the higher this value, the lower the toxicity
|
Minimum inhibitory concentration (mM) |
[C2mim]Cl |
261.8 |
[C4mim]Cl |
207.5 |
[C6mim]Cl |
7.8 |
[C8mim]Cl |
0.5 |
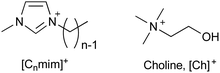 |
| Fig. 2 Ionic liquid cations presented in this review. | |
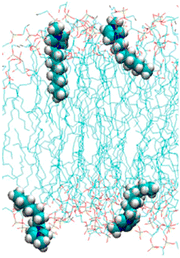 |
| Fig. 3 Cell membrane with inserted [C8mim]+. Adapted with permission.41 Copyright 2014 American Chemical Society. | |
[C2mim]OAc has a lethal effect on the growth and fermentation of Saccharomyces cerevisiae (S. cerevisiae) and E. coli even at concentrations below 1%.20 Comparatively, [C2mim]Cl is slightly less toxic, but still has a significant impact at concentrations of 1–2%.20 Additionally, phosphate ionic liquids exhibit a similar toxicity to chloride ionic liquids.48,49 Therefore, typical imidazolium-based ionic liquids are effective pretreatment solvents,50,51 but are highly toxic and unsuitable for successive/simultaneous ethanol production processes.
Countermeasures against ionic liquid toxicity
Various countermeasures have been proposed to counteract the toxicity of typical ionic liquids, which are broadly classified into three categories.
1. Increase the concentration of microorganisms
The simplest countermeasure is to increase the concentration of microorganisms. Generally, most microorganisms can resist some stresses including ionic liquids at high microbial concentrations. For example, it has been reported that concentrated S. cerevisiae can produce ethanol with little inhibition in a 3% [C2mim]OAc solution.49 A similar trend has been shown with recombinant E. coli (E. coli KO11) that can ferment ethanol.48
Increasing the concentration of microorganisms also contributes to increasing the ethanol yield. When fermentation is initiated with a high microbial concentration, glucose is not used for microbial growth, thereby improving the ethanol yield.
2. Use of ionic liquid-tolerant microorganisms
2-(1) Search for ionic liquid-tolerant microorganisms.
Yarrowia lipolytica (Y. lipolytica), a type of yeast, is highly tolerant to ionic liquids.52 For example, Y. lipolytica is stress tolerant by nature and has the ability to adapt to a wide range of pH (2–11) and high salt concentration such as 12% NaCl. Therefore, it can grow and ferment even in 10% [C2mim]OAc. Although it is not capable of producing ethanol, it can produce useful substances such as α-ketoglutaric acid and eicosapentaenoic acid. Among yeasts capable of ethanol fermentation, Kazachstania telluris and Wickerhamomyces anomalus exhibit high ionic liquid tolerance;53 however, it is not as high as that of Y. lipolytica.
2-(2) Incubation in ionic liquids to confer ionic liquid tolerance.
Walker et al. succeeded in improving the ionic liquid tolerance of Y. lipolytica.54 They started culturing Y. lipolytica in 5% [C2mim]OAc and gradually increased the concentration of [C2mim]OAc to obtain a Y. lipolytica strain that could grow in an 18% [C2mim]OAc solution. This strain showed an increase in the phospholipid content and changes in its composition, as well as an increase in sterol content after incubation. In particular, it has been suggested that the decrease in cell membrane fluidity associated with the increase in sterol content is important.
2-(3) Gene modification to confer ionic liquid tolerance.
To confer ionic liquid tolerance to microorganisms via genetic modification, it is necessary to identify factors that are important for ionic liquid tolerance. Ionic liquids affect the mitochondria in S. cerevisiae because of the cation uptake transporters.55 Therefore, attempts have been made to knock out its related gene, ptk2, to avoid the uptake of ionic liquids. The ptk2 knockout greatly improved the resistance to [C2mim]OAc and [C2mim]Cl.55 Furthermore, E. coli with improved ionic liquid resistance by genetic recombination has been reported.56
Other mechanisms of ionic liquid resistance have been investigated in Enterobacter lignolyticus.55 These include: (1) decreased intracellular permeability of ionic liquids due to changes in the phospholipid composition and reduction of porin (a passive transporter that penetrates through the plasma membrane), (2) extracellular efflux of ionic liquids by multidrug efflux pumps, and (3) accumulation of sugars and amino acids to confer osmotolerance. It can be expected that ionic liquid tolerance will be conferred by using these mechanisms.
3. Use of low toxicity ionic liquids
Although [C2mim]+-based ionic liquids effectively delignify and reduce cellulose crystallinity in biomass,10,57,58 they are basically highly toxic. Therefore, low-toxicity ionic liquids are being developed. One strategy for developing low-toxicity ionic liquids is to use bio-derived ions such as choline acetate ([Ch]OAc). As expected, [Ch]OAc has been reported to be less toxic than [C2mim]OAc.59 Although its pretreatment capacity is somewhat less than that of [C2mim]OAc, it is reported to be adequate.60–62 Pretreatment with [Ch]OAc also occurs through the mechanisms of cellulose crystallinity reduction and delignification. However, despite its low toxicity toward microbial growth, it is highly toxic to fermentation, resulting in low ethanol yields (Table 2).48
Table 2 Half maximal effective concentration (EC50) of zwitterions, an ionic liquid, organic solvents against E. coli KO11 and relative ethanol concentration in 0.5 mol L−1 solutions.45,48
|
|
EC50 (g L−1) |
Relative ethanol concentration (%) |
Pretreatment ability |
Zwitterion |
OE2imC3C |
158 |
96 |
Yes |
|
C1imC3C |
141 |
100 |
Yes |
|
C1imC3S |
>200 |
104 |
No |
Ionic liquid |
[Ch]OAc |
70 |
15 |
Yes |
Organic solvent |
Dimethyl sulfoxide |
91 |
— |
No |
|
Ethanol |
17 |
— |
No |
Control |
— |
— |
100 |
— |
Ionic liquids with choline cations and amino acid anions have also been reported.60,63–66 These ionic liquids are capable of pretreating biomass, with choline lysinate being particularly effective. The primary pretreatment mechanism of choline lysinate is delignification, but it can also slightly reduce cellulose crystallinity.
Artificial ions can also exhibit low toxicity. Zwitterionic liquids (e.g., OE2imC3C; Fig. 4), in which the anion and cation are covalently bonded, have been reported to be much less toxic than the corresponding ionic liquids.45,48,67,68 The greatest advantage of zwitterionization is that zwitterionic liquids exhibit low toxicity without loss of their functionality, because the constituent cations or anions are the same as the original ionic liquids. OE2imC3C, which combines an imidazolium cation with a carboxylic acid anion, can not only perform delignification and reduce cellulose crystallinity, but also dissolution of cellulose.45,48,68–70 In addition, microbial fermentation is possible even in OE2imC3C solutions of more than 50 wt%, showing its extremely low toxicity.48
 |
| Fig. 4 Structures of zwitterions described in this review. | |
Feasibility of continuous ethanol production
One-pot ethanol production from plant biomass is at the edge of realization through the various innovations described above. In particular, the Joint BioEnergy Institute and its affiliated research institutes in the United States are vigorously pursuing research toward practical application, including protic ionic liquids and deep eutectic solvents.66,71–75 They have already been continuously converting tens of kilograms of woody biomass into ethanol with relatively high yields.72 The one-pot ethanol production process also reduces the capital cost and wastewater treatment cost. They then estimate the production cost of ethanol to be approximately $5.3 per gallon, which can be lowered to $1.8 per gallon with further improvements.72 The price is similar or a little bit higher than the case produced by the other pretreatment methods,76 although precise comparison of the values from different literature is difficult. Reducing the price of ethanol to match that of gasoline will facilitate the practical application of bioethanol.
Conclusions
The practical application of cellulosic bioethanol is an extremely difficult task. It is evidenced by the fact that it has not yet been economically industrialized on a large scale, despite knowing that “cell walls are made of glucose units” for 100 years. However, as mentioned in the previous section, the possibility of practical application is gradually emerging. In Europe, a venture company, Lixea, has been established, and its practical application is expected in the future.
On the other hand, bioethanol production includes chemical, enzymatic, and microbial processes, requiring interdisciplinary insights and techniques such as those from biochemistry, genetics, organic chemistry, and chemical engineering. Agriculture is also important considering the cultivation of biomass. Thus, new ideas and innovation are urgently required from areas other than of ionic liquids and biomass pretreatment.
While this paper mainly focuses on ethanol production by yeast, a wide variety of fermentation products can be obtained from glucose and other sugars. For example, limonene56 and isopentenol66 were produced by E. coli, which can be easily genetically modified. The technologies described in this review are expected to expand into many other areas, not limited to bioethanol production.
Conflicts of interest
There are no conflicts to declare.
Acknowledgements
I thank Dr K. Ninomiya and Dr Y. Tsuge (Kanazawa Univ.) for their instruction of philosophy on microbial biology. This study was also partly supported by KAKENHI (18K14281, 23H03824, 23KF0226 from the Japan Society for the Promotion of Science), Leading Initiative for Excellent Young Researchers (for K. K., from Ministry of Education, Culture, Sports, Science and Technology-Japan), ACT-X (for K. K., JPMJAX1915 from Japan Science and Technology Agency), A-STEP (from Japan Science and Technology Agency), Kanazawa University SAKIGAKE project 2020, 2022, and NIBB Collaborative Research Program (21-608), Research Center for Thermotolerant Microbial Resources of Yamaguchi University (2023-01).
Notes and references
- A. P. C. Faaij, Energy Policy, 2006, 34, 322–342 CrossRef.
- A. J. Ragauskas, C. K. Williams, B. H. Davison, G. Britovsek, J. Cairney, C. A. Eckert, W. J. Frederick, J. P. Hallett, D. J. Leak, C. L. Liotta, J. R. Mielenz, R. Murphy, R. Templer and T. Tschaplinski, Science, 2006, 311, 484–489 CrossRef CAS PubMed.
- M. E. Himmel, S.-Y. Ding, D. K. Johnson, W. S. Adney, M. R. Nimlos, J. W. Brady and T. D. Foust, Science, 2007, 315, 804–807 CrossRef CAS PubMed.
- S. Y. Ding, Y. S. Liu, Y. N. Zeng, M. E. Himmel, J. O. Baker and E. A. Bayer, Science, 2012, 338, 1055–1060 CrossRef CAS PubMed.
- T. Welton, Chem. Rev., 1999, 99, 2071–2083 CrossRef CAS PubMed.
- M. Armand, F. Endres, D. R. MacFarlane, H. Ohno and B. Scrosati, Nat. Mater., 2009, 8, 621–629 CrossRef CAS PubMed.
- R. D. Rogers and K. R. Seddon, Science, 2003, 302, 792–793 CrossRef PubMed.
- Z. Lei, B. Chen, Y. M. Koo and D. R. MacFarlane, Chem. Rev., 2017, 117, 6633–6635 CrossRef PubMed.
- B. D. Rabideau, K. N. West and J. H. Davis, Chem. Commun., 2018, 54, 5019–5031 RSC.
- A. Brandt, J. Gräsvik, J. P. Hallett and T. Welton, Green Chem., 2013, 15, 550–583 RSC.
- P. Walden, Bull. Acad. Imp. Sci. St.-Petersbourg, 1914, 8, 405–422 Search PubMed.
- J. S. Wilkes and M. J. Zaworotko, J. Chem. Soc., Chem. Commun., 1992, 965–967 RSC.
- J. S. Wilkes, Green Chem., 2002, 4, 73–80 RSC.
- A. M. Lopes and R. Bogel-Lukasik, ChemSusChem, 2015, 8, 947–965 CrossRef PubMed.
- S. Stolte, M. Matzke, J. Arning, A. Böschen, W.-R. Pitner, U. Welz-Biermann, B. Jastorff and J. Ranke, Green Chem., 2007, 9, 1170–1179 RSC.
- T. P. Pham, C. W. Cho and Y. S. Yun, Water Res., 2010, 44, 352–372 CrossRef CAS PubMed.
- A. Romero, A. Santos, J. Tojo and A. Rodriguez, J. Hazard. Mater., 2008, 151, 268–273 CrossRef CAS PubMed.
- D. Zhao, Y. Liao and Z. Zhang, Clean: Soil, Air, Water, 2007, 35, 42–48 CAS.
- J. Łuczak, C. Jungnickel, I. Łacka, S. Stolle and J. Hupka, Green Chem., 2010, 12, 593–601 RSC.
- M. Ouellet, S. Datta, D. C. Dibble, P. R. Tamrakar, P. I. Benke, C. L. Li, S. Singh, K. L. Sale, P. D. Adams, J. D. Keasling, B. A. Simmons, B. M. Holmes and A. Mukhopadhyay, Green Chem., 2011, 13, 2743–2749 RSC.
- N. Tamai, H. Aono, D. Tatsumi and T. Matsumoto, Nihon Reoroji Gakkaishi, 2003, 31, 119–130 CrossRef CAS.
- D. Klemm, B. Heublein, H.-P. Fink and A. Bohn, Angew. Chem., Int. Ed., 2005, 44, 3358–3393 CrossRef CAS PubMed.
- M. W. Frey, L. Li, M. Xiao and T. Gould, Cellulose, 2006, 13, 147–155 CrossRef CAS.
- Y. Fukaya, A. Sugimoto and H. Ohno, Biomacromolecules, 2006, 7, 3295–3297 CrossRef CAS PubMed.
- I. Kilpelainen, H. Xie, A. King, M. Granstrom, S. Heikkinen and D. S. Argyropoulos, J. Agric. Food Chem., 2007, 55, 9142–9148 CrossRef PubMed.
- N. Sun, H. Rodriguez, M. Rahman and R. D. Rogers, Chem. Commun., 2011, 47, 1405–1421 RSC.
- Y. Fukaya, K. Hayashi, M. Wada and H. Ohno, Green Chem., 2008, 10, 44–46 RSC.
- H. Ohno and Y. Fukaya, Chem. Lett., 2009, 38, 2–7 CrossRef CAS.
- B. Zhao, L. Greiner and W. Leitner, RSC Adv., 2012, 2, 2476–2479 RSC.
- M. Abe, Y. Fukaya and H. Ohno, Green Chem., 2010, 12, 1274 RSC.
- A. M. da Costa Lopes, K. G. Joao, D. F. Rubik, E. Bogel-Lukasik, L. C. Duarte, J. Andreaus and R. Bogel-Lukasik, Bioresour. Technol., 2013, 142, 198–208 CrossRef CAS PubMed.
- R. P. Swatloski, S. K. Spear, J. D. Holbrey and R. D. Rogers, J. Am. Chem. Soc., 2002, 124, 4974–4975 CrossRef CAS PubMed.
- H. Zhang, J. Wu, J. Zhang and J. S. He, Macromolecules, 2005, 38, 8272–8277 CrossRef CAS.
- M. Abe, T. Yamada and H. Ohno, RSC Adv., 2014, 4, 17136–17140 RSC.
- M. Abe, S. Yamanaka, H. Yamada, T. Yamada and H. Ohno, Green Chem., 2015, 17, 4432–4438 RSC.
- M. Abe, K. Kuroda and H. Ohno, ACS Sustainable Chem. Eng., 2015, 3, 1771–1776 CrossRef CAS.
- H. Wang, G. Gurau and R. D. Rogers, Chem. Soc. Rev., 2012, 41, 1519–1537 RSC.
- Z. Usmani, M. Sharma, P. Gupta, Y. Karpichev, N. Gathergood, R. Bhat and V. K. Gupta, Bioresour. Technol., 2020, 304, 123003 CrossRef CAS PubMed.
- R. M. Wahlström and A. Suurnäkki, Green Chem., 2015, 17, 694–714 RSC.
- A. A. Elgharbawy, M. Z. Alam, M. Moniruzzaman and M. Goto, Biochem. Eng. J., 2016, 109, 252–267 CrossRef CAS.
- G. S. Lim, J. Zidar, D. W. Cheong, S. Jaenicke and M. Klähn, J. Phys. Chem. B, 2014, 118, 10444–10459 CrossRef CAS PubMed.
- R. J. Bingham and P. Ballone, J. Phys. Chem. B, 2012, 116, 11205–11216 CrossRef CAS PubMed.
- B. Jing, N. Lan, J. Qiu and Y. Zhu, J. Phys. Chem. B, 2016, 120, 2781–2789 CrossRef CAS PubMed.
- C. M. N. Mendonça, D. T. Balogh, S. C. Barbosa, T. E. Sintra, S. P. M. Ventura, L. F. G. Martins, P. Morgado, E. J. M. Filipe, J. A. P. Coutinho, O. N. Oliveira Jr and A. Barros-Timmons, Phys. Chem. Chem. Phys., 2018, 20, 29764–29777 RSC.
- T. Komori, H. Satria, K. Miyamura, A. Ito, M. Kamiya, A. Sumino, T. Onishi, K. Ninomiya, K. Takahashi, J. L. Anderson, T. Uto and K. Kuroda, ACS Sustainable Chem. Eng., 2021, 9, 11825–11836 CrossRef CAS.
- S. M. Lee, W. J. Chang, A. R. Choi and Y. M. Koo, Korean J. Chem. Eng., 2005, 22, 687–690 CrossRef CAS.
- Q. Dickinson, S. Bottoms, L. Hinchman, S. McIlwain, S. Li, C. L. Myers, C. Boone, J. J. Coon, A. Hebert, T. K. Sato, R. Landick and J. S. Piotrowski, Microb. Cell Fact., 2016, 15, 17 CrossRef PubMed.
- K. Kuroda, H. Satria, K. Miyamura, Y. Tsuge, K. Ninomiya and K. Takahashi, J. Am. Chem. Soc., 2017, 139, 16052–16055 CrossRef CAS PubMed.
- K. Nakashima, K. Yamaguchi, N. Taniguchi, S. Arai, R. Yamada, S. Katahira, N. Ishida, H. Takahashi, C. Ogino and A. Kondo, Green Chem., 2011, 13, 2948–2953 RSC.
- B. D. Rabideau, A. Agarwal and A. E. Ismail, J. Phys. Chem. B, 2013, 117, 3469–3479 CrossRef CAS PubMed.
- T. Uto, K. Yamamoto and J. I. Kadokawa, J. Phys. Chem. B, 2018, 122, 258–266 CrossRef CAS PubMed.
- S. Ryu, N. Labbe and C. T. Trinh, Appl. Microbiol. Biotechnol., 2015, 99, 4237–4244 CrossRef CAS PubMed.
- I. R. Sitepu, S. Shi, B. A. Simmons, S. W. Singer, K. Boundy-Mills and C. W. Simmons, FEMS Yeast Res., 2014, 14, 1286–1294 CrossRef CAS PubMed.
- C. Walker, S. Ryu and C. T. Trinh, Metab. Eng., 2019, 54, 83–95 CrossRef CAS PubMed.
- J. I. Khudyakov, P. D'haeseleer, S. E. Borglin, K. M. Deangelis, H. Woo, E. A. Lindquist, T. C. Hazen, B. A. Simmons and M. P. Thelen, Proc. Natl. Acad. Sci. U. S. A., 2012, 109, E2173–E2182 CrossRef CAS PubMed.
- M. Frederix, F. Mingardon, M. Hu, N. Sun, T. Pray, S. Singh, B. A. Simmons, J. D. Keasling and A. Mukhopadhyay, Green Chem., 2016, 18, 4189–4197 RSC.
- N. Sun, M. Rahman, Y. Qin, M. L. Maxim, H. Rodríguez and R. D. Rogers, Green Chem., 2009, 11, 646 RSC.
- W. Y. Li, N. Sun, B. Stoner, X. Y. Jiang, X. M. Lu and R. D. Rogers, Green Chem., 2011, 13, 2038–2047 RSC.
- K. Ninomiya, C. Ogino, M. Ishizaki, M. Yasuda, N. Shimizu and K. Takahashi, Biochem. Eng. J., 2015, 103, 198–204 CrossRef CAS.
- N. Sun, R. Parthasarathi, A. M. Socha, J. Shi, S. Zhang, V. Stavila, K. L. Sale, B. A. Simmons and S. Singh, Green Chem., 2014, 16, 2546–2557 RSC.
- K. Ninomiya, T. Yamauchi, M. Kobayashi, C. Ogino, N. Shimizu and K. Takahashi, Biochem. Eng. J., 2013, 71, 25–29 CrossRef CAS.
- K. Ninomiya, H. Soda, C. Ogino, K. Takahashi and N. Shimizu, Bioresour. Technol., 2013, 128, 188–192 CrossRef CAS PubMed.
- X. D. Hou, T. J. Smith, N. Li and M. H. Zong, Biotechnol. Bioeng., 2012, 109, 2484–2493 CrossRef CAS PubMed.
- Q. P. Liu, X. D. Hou, N. Li and M. H. Zong, Green Chem., 2012, 14, 304–307 RSC.
- F. Xu, J. Sun, N. V. S. N. M. Konda, J. Shi, T. Dutta, C. D. Scown, B. A. Simmons and S. Singh, Energy Environ. Sci., 2016, 9, 1042–1049 RSC.
- M. J. Liszka, A. Kang, N. V. S. N. M. Konda, K. Tran, J. M. Gladden, S. Singh, J. D. Keasling, C. D. Scown, T. S. Lee, B. A. Simmons and K. L. Sale, Green Chem., 2016, 18, 4012–4021 RSC.
- K. Kuroda, T. Komori, K. Ishibashi, T. Uto, I. Kobayashi, R. Kadokawa, Y. Kato, K. Ninomiya, K. Takahashi and E. Hirata, Commun. Chem., 2020, 3, 163 CrossRef CAS PubMed.
- H. Satria, K. Kuroda, Y. Tsuge, K. Ninomiya and K. Takahashi, New J. Chem., 2018, 42, 13225–13228 RSC.
- R. Kadokawa, T. Endo, Y. Yasaka, K. Ninomiya, K. Takahashi and K. Kuroda, ACS Sustainable Chem. Eng., 2021, 9, 8686–8691 CrossRef CAS.
- A. Hachisu, H. Tobe, K. Ninomiya, K. Takahashi and K. Kuroda, ACS Sustainable Chem. Eng., 2022, 10, 6919–6924 CrossRef CAS.
- L. Das, E. C. Achinivu, C. A. Barcelos, E. Sundstrom, B. Amer, E. E. K. Baidoo, B. A. Simmons, N. Sun and J. M. Gladden, ACS Sustainable Chem. Eng., 2021, 9, 4422–4432 CrossRef CAS.
- C. A. Barcelos, A. M. Oka, J. Yan, L. Das, E. C. Achinivu, H. Magurudeniya, J. Dong, S. Akdemir, N. R. Baral, C. Yan, C. D. Scown, D. Tanjore, N. Sun, B. A. Simmons, J. Gladden and E. Sundstrom, ACS Sustainable Chem. Eng., 2021, 9, 4042–4053 CrossRef CAS.
- J. Sun, N. V. S. N. M. Konda, R. Parthasarathi, T. Dutta, M. Valiev, F. Xu, B. A. Simmons and S. Singh, Green Chem., 2017, 19, 3152–3163 RSC.
- J. A. Pérez Pimienta, G. Papa, J. Sun, V. Stavila, A. Sanchez, J. M. Gladden and B. A. Simmons, Green Chem., 2022, 24, 207–217 RSC.
- F. Xu, J. Sun, M. Wehrs, K. H. Kim, S. S. Rau, A. M. Chan, B. A. Simmons, A. Mukhopadhyay and S. Singh, ACS Sustainable Chem. Eng., 2018, 6, 8914–8919 CrossRef CAS.
- A. R. C. Morais, J. Zhang, H. Dong, W. G. Otto, T. Mokomele, D. Hodge, V. Balan, B. E. Dale, R. M. Lukasik and L. da Costa Sousa, Green Chem., 2022, 24, 4443–4462 RSC.
|
This journal is © The Royal Society of Chemistry and the Centre National de la Recherche Scientifique 2024 |
Click here to see how this site uses Cookies. View our privacy policy here.