DOI:
10.1039/D3NR06015B
(Communication)
Nanoscale, 2024,
16, 4563-4570
A homologous series of macrocyclic Ni clusters: synthesis, structures, and catalytic properties†
Received
26th November 2023
, Accepted 16th January 2024
First published on 19th January 2024
Abstract
Due to their intriguing ring structures and promising applications, nickel–thiolate clusters, such as [Nin(SR)2n] (n = 4–6), have attracted tremendous interest. However, investigation of the synthesis, structures, and properties of macrocyclic Nin clusters (n > 8) has been seriously impeded. In this work, a homologous series of macrocyclic nickel clusters, Nin(4MPT)2n (n = 9–12), was fabricated by using 4-methylphenthiophenol (4MPT) as the ligand. The structures and compositions of the clusters were determined by single-crystal X-ray diffraction (SXRD) in combination with electrospray ionization mass spectrometry (ESI-MS). Experimental results and theoretical calculations show that the electronic structures of the clusters do not change significantly with the increase of Ni atoms. The coordination interactions between Ni and S atoms in [NiS4] subunits are proved to play a crucial rule in the remarkable stability of Ni clusters. Finally, these clusters display excellent catalytic activity towards the reduction of p-nitrophenol, and a linear correlation between catalytic activity and ring size was revealed. The study provides a facile approach to macrocyclic homoleptic nickel clusters, and contributes to an in-depth understanding of the structure–property correlations of nickel clusters at the atomic level.
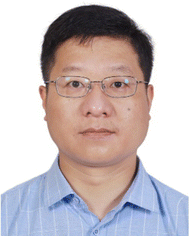 Chuanhao Yao | Chuanhao Yao received his Ph.D. degree from the University of Chinese Academy of Sciences in 2016. Subsequently, he carried out postdoctoral research at the Department of Chemistry, National University of Singapore (NUS). He attained his professor position at Northwestern Polytechnical University (NPU) in 2019, and in early 2023, he moved to Fujian Normal University as a full professor. His research interests are focused on the design, synthesis and characterization of atomically precise metal clusters and their applications in catalysis, biomedicine, and light-emitting and flexible electronic devices. |
1. Introduction
Metal clusters have attracted extensive interest due to their precise compositions as well as their well-defined structures, which provide excellent platforms to elucidate the relationships between their structures and properties.1–5 The last two decades have witnessed breakthroughs in the synthesis, characterization, and property exploration of group 11 transition metal (Au, Ag, Cu) clusters and their alloys;6–17 however, the study of the metal clusters composed of group 10 transition metals (Ni, Pd, Pt) is still limited. In particular, the synthesis of core–shell structured clusters is extremely challenging; instead, cyclic polynuclear, tiara-like Ni clusters are readily available.18–31 The development of the cyclic polynuclear Ni6(SR)12 clusters dates back to the 1960s.18,19 Since then, nickel clusters have received increasing attention, probably because of their low price and availability of large reserves of nickel compared to platinum and palladium.21,25,28,32 As a medium-sized cyclic nickel cluster, Ni6 clusters stabilized by diverse ligands have been intensively studied.18–26,33,34 For example, Woodward and co-workers reported the first representative of a Ni6 cluster, which was obtained from the reaction of diethyl disulfide with nickel carbonyl in benzene.18 Subsequently, cyclic Ni6 clusters stabilized by other thiol ligands have also been reported, and in-depth investigations into their structure and properties conducted.19–26,33,34 In addition, smaller size nickel clusters such as Ni4 and Ni5 have also been prepared, and structure characterization results demonstrated that both have crown-like structures built up from approximately planar [NiS4] units linked by bridged thiolate ligands.27,29–32,35 Such series of small-ring Nin clusters (n = 4, 5, 6) are easy to prepare and display highly symmetric polygonal structures, probably because of their aromaticity.27,36,37 The delocalization of the d-orbital electrons across the Nin rings leads to bond length equalization and thereby aromaticity.27 The structures of the small Nin (n = 4, 5, 6) rings are not sensitive to the type of ligands utilized; however, this is not always the case when the size of clusters becomes larger. For example, ring-structured Ni8(SCH2COOEt)16 was produced in 1985;38 however, by using NaSC4H9 instead of the HSCH2COOEt ligand, a non-ring structured nickel sulfide thiolate cluster, [Ni8S(SC4H9)9], was reported by Henkel and coworkers.39 In another contribution, the intriguing cyclic structures of Ni9(SPh)18 and Ni11(SPh)22 were determined by Dahl and coworkers, but Ni10(SPh)20 was not reported in their work.40 Subsequently, the cyclic Ni10 and Ni12 were revealed, in which the nickel atoms were stabilized by two different types of thiolate ligands, but Ni11 was missing.41 As a dodecanuclear cluster, Ni12(tBu3SiS)12Br12 was produced by Sydora and coworkers, and in this cluster, the nickel atoms are linked by a thiolate ligand and bromine atom to form a tetrahedral geometry, distinctly different from the planar [NiS4] subunits previously reported.42 These works make us wonder whether the homoleptic Ni9(SR)18, Ni10(SR)20, Ni11(SR)22, and Ni12(SR)24 do exist or not. Based on the discussion above, a consensus that ligands play an important role in dictating the structure of clusters, can be reached.32,43–46 In other words, the structures of larger nickel clusters are sensitive to the ligands utilized. To date, the synthesis of larger sized nickel clusters is still challenging, and the in-depth structure–property understanding of the macrocyclic nickel clusters has been impeded.
Herein, we report the synthesis of a sequence of macrocyclic nickel clusters, Nin(4MPT)2n (n = 9–12), protected by the same ligand (4MPT = 4-methylphenthiophenol), where their compositions and structures are determined by electrospray ionization mass spectrometry (ESI-MS) in combination with single-crystal X-ray diffraction (SXRD). The results show that all these nickel clusters possess ring structures. The primary structures of Ni9(4MPT)18 and Ni11(4MPT)22 are similar to those of the reported Ni9(SPh)18 and Ni11(SPh)22.40 However, the structures of the Ni10(4MPT)20, and Ni12(4MPT)24 are novel and distinct from the structures of Ni10 and Ni12 clusters protected by two kinds of thiolate ligands.41 Most interestingly, although the clusters become larger with increased numbers of Ni atoms, their electronic structures and optical properties do not change dramatically. Finally, the reduction of p-nitrophenol catalyzed by these homologous series of nickel clusters was investigated. The results indicate that these clusters show excellent catalytic activity towards the reduction of p-nitrophenol, and a linear correlation between catalytic activity and nickel ring size was revealed.
2. Results and discussion
The clusters were synthesized by a one-pot method. Typically, in a 50 mL round-bottomed flask, 24 mg of NiCl2·6H2O was dissolved in 20 mL of absolute ethanol containing 15 mg of 4MPT as the ligand, under vigorous stirring. Subsequently, 20 mg of NaBH4 (which had been dissolved in 3 mL of absolute ethanol in advance) was added to the reaction solution, and the color of the solution changed from green to dark. After reacting for 12 h, the crude products were collected by centrifugation and washed with ethanol three times. 2 mL of dichloromethane (DCM) was used as the extraction agent, and the nickel cluster homologues were separated by thin layer chromatography (TLC), as shown in Fig. S1.†
To determine their precise structures, we attempted the crystallization of the as-synthesized clusters using a typical recipe involving the diffusion of methanol into a toluene-based cluster solution.47,48 After a week, black sheets or ribbons were obtained, as shown in Fig. S1.† Single-crystal X-ray diffraction (SXRD) analysis reveals that the clusters obtained herein are members of a homologous series of macrocyclic Ni clusters, that is, Nin(4MPT)2n, (n = 9–12). All the Ni clusters feature macrocyclic structures, and they may be ideally viewed as a convex n-polygon of coplanar nickel atoms with two sulfur atoms bridging each pair of adjacent nickel atoms, as shown in Fig. 1 and Fig. S2.† The NinS2n frameworks of Ni9(4MPT)18 and Ni11(4MPT)22 (Fig. 1a and c) are similar to those of Ni9(SPh)18 and Ni11(SPh)22;40 however, for those of homoleptic Ni10(4MPT)20 and Ni12(4MPT)24, both frameworks are unique. The nickel–sulfur frameworks of Ni10(4MPT)20 and Ni12(4MPT)24 can be viewed as a truncated rectangle and truncated square, respectively, as shown in Fig. 1b and d, totally differing from those of [Ni(StBu)(pyet)]10 and [Ni(StBu)(etet)]12, in which a circular or elliptical geometry was observed.41 Subsequently, the molecular parameters of the Ni–S framework of the Nin(4MPT)2n clusters were studied. The Ni–Ni distances, the Ni–S–Ni angles, and the Ni–S bond length of the Nin(4MPT)2n (n = 9–12) clusters are shown in Fig. S3, S4, and S5.† It is clear that the NinS2n frameworks of Ni9(4MPT)18, Ni10(4MPT)20 and Ni12(4MPT)24 are more regular than that of Ni11(4MPT)22, indicated by the more regular Ni–Ni distances, Ni–S–Ni angles, and Ni–S bond length of the Nin(4MPT)2n (n = 9, 10, 12) clusters. The top views of the NinS2n frameworks (n = 9, 10, 12) in Fig. 1 reveal D3h, D2h, and D4h symmetries, respectively. Nevertheless, the Ni11S22 architecture discloses a highly deformed toroidal geometry of pseudo-C2v symmetry. A detailed crystal structure analysis shows that to minimize steric interactions of the ligands, the –PhCH3 substituents of the ligands in Nin(4MPT)2n (n = 9, 10, 12) were found to be sterically disposed in alternating axial and equatorial positions about the nickel rings; however, in Ni11(4MPT)22, two of the axial substituents are bent inward, resulting in distortion of the ring, as shown in Fig. S2c.† To confirm the validity of the composition and the charge state of the clusters, ESI-MS measurements were conducted, as shown in Fig. 2. The ESI-MS spectra show peaks at m/z ∼2745.88, 3051.86, 3357.88, and 1963.83 Da, respectively, corresponding to the molecular ion peaks of [Ni9(4MPT)18 + H]+, [Ni10(4MPT)20 + H]+, [Ni11(4MPT)22 + H]+, and [Ni12(4MPT)24 + 2Cs]2+ adducts, as evidenced by the well-matched high-resolution experimental MS spectra compared with the calculated values of each cluster. In addition, the data were acquired in a positive model, and the results indicate that all the Nin(4MPT)2n (n = 9–12) clusters bear one or two positive charges by combining one or two H+/Cs+ ions. The charge states of the clusters are equal to the number of H+ or Cs+ ions combined, implying that these clusters are neutral.
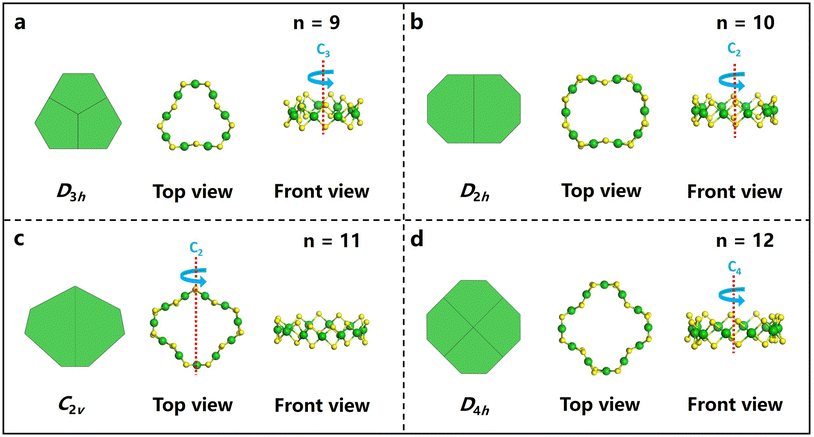 |
| Fig. 1 Structure analysis of the Nin(4MPT)2n clusters. (a) Ni9(4MPT)18. (b) Ni10(4MPT)20. (c) Ni11(4MPT)22. (d) Ni12(4MPT)24. Color codes: yellow sphere, S; green sphere, Ni. All C and H atoms are omitted for clarity. | |
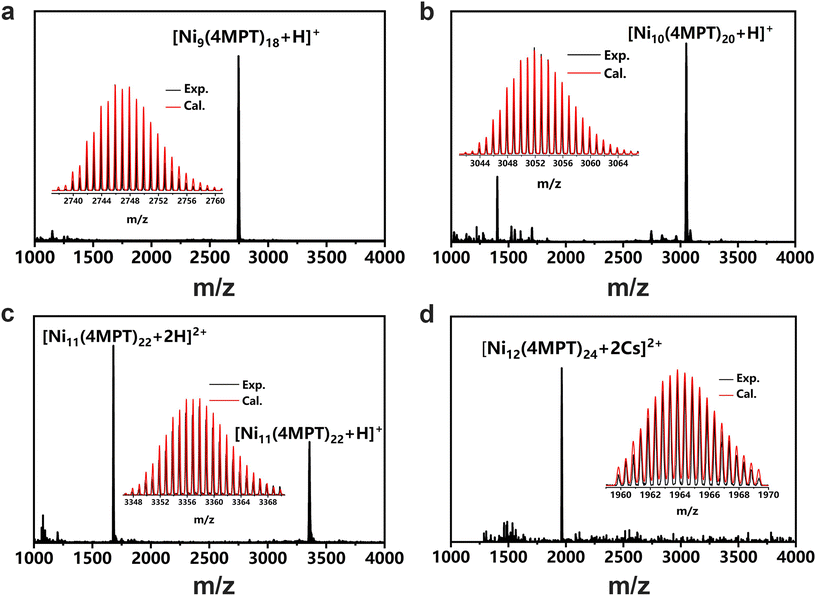 |
| Fig. 2 ESI-MS of the Nin(4MPT)2n clusters. (a) Ni9(4MPT)18. (b) Ni10(4MPT)20. (c) Ni11(4MPT)22. (d) Ni12(4MPT)24. Inset: the measured (black) and simulated (red) isotopic distribution patterns of the corresponding molecular ion peaks. | |
The UV-vis-NIR spectra of the clusters are shown in Fig. 3. For each cluster, there are three featured absorption peaks located at ∼345 and ∼470 nm, as well as a shoulder at ∼600 nm. The similar absorption profiles of the clusters imply that the electronic structures of the clusters may have something in common. Subsequently, quantum chemical calculations were carried out to reveal the electronic structures and optical behaviors of the Nin(4MPT)2n (n = 9–12) clusters. The toroidal framework of each cluster is constructed from n continuously linked planar [NiS4] subunits. The total structures of Ni clusters are distorted to D3-Ni9(4MPT)18, D2-Ni10(4MPT)20, C1-Ni11(4MPT)22, and D4-Ni12(4MPT)24 symmetries, respectively, through coordination with bridging ligands (4MPT). The Ni–Ni and Ni–S bond lengths of the optimized structures listed in Table S1† show an identical trend and agree well with those of the experimental results (see Fig. S3 and S4†). We find that the Ni clusters suggest similar electronic configurations and atomic orbital distributions, with all Ni-3d orbitals located around the Fermi level, as can be seen from the projected density of states in Fig. S6† and the schematic Kohn–Sham molecular orbital energy level diagram in Fig. 4a. Additionally, the number of vacant Ni-3d-dominated MOs is given by n and the number of occupied MOs equals 4n, indicating the formal oxidation state of the Ni atom to be +2. There are weak bonding interactions between Ni-3dxy, dx2–y2, dz2, and S-2p orbitals, contributing to the nearly non-bonding HOMO and the adjacent occupied MOs, as depicted in Fig. 4b. However, the LUMO and the adjacent vacant MOs mainly arise from strong anti-bonding σ interactions between Ni-3dxz,yz and S-2p orbitals (see Fig. S7†), which illustrates the large HOMO–LUMO gaps and robust stabilities of Ni clusters. The computed absorption spectra and peak features from the TDDFT calculations fit well with the experimental spectra (see Fig. S8†), with the largest peak position error being 46 nm. Taking Ni12(4MPT)24 as an example, the cluster reveals three peaks (Fig. 4c), where electron excitations of strong peaks I and II correspond to transitions from the ligand-dominated orbitals in the lower and higher energy regions to vacant Ni-3d-dominated orbitals, respectively. The lower-energy peak III is weaker as it primarily comes from electron transitions between Ni-3d-dominated orbitals.
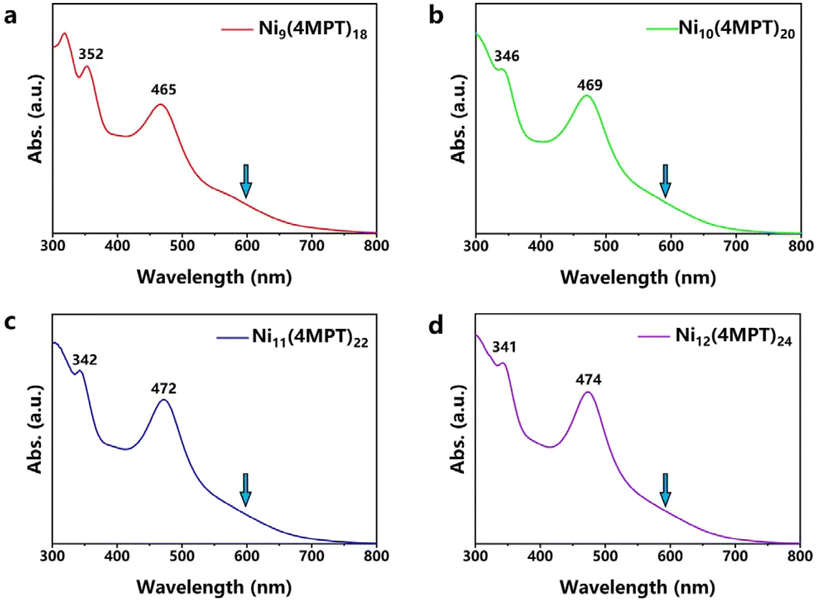 |
| Fig. 3 UV-vis-NIR spectra of the Nin(4MPT)2n clusters. (a) Ni9(4MPT)18. (b) Ni10(4MPT)20. (c) Ni11(4MPT)22. (d) Ni12(4MPT)24. | |
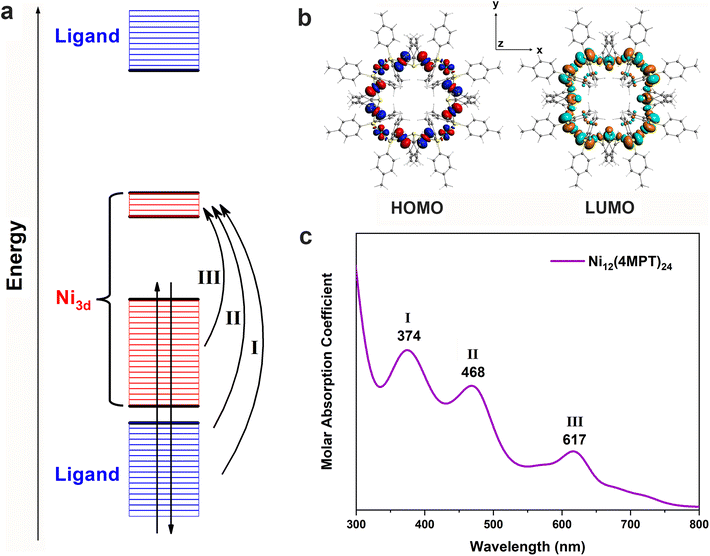 |
| Fig. 4 Theoretical results. (a) Schematic Kohn–Sham molecular orbital energy level diagram of nickel clusters. (b) The highest occupied molecular orbital (HOMO) and lowest unoccupied molecular orbital (LUMO) of the Ni12(4MPT)24 cluster using the PBE functional (isovalue = 0.015 a.u.). (c) The simulated UV-vis spectrum of the Ni12(4MPT)24 cluster at the TDDFT/LB94 SR-ZORA level of theory with the Gaussian half-width of 45 nm, where the electron transition channels of peaks I, II, and III are shown in (a). | |
Previous studies have shown that the electronic structures of gold or silver clusters are very sensitive to their composition. In some cases, even replacing, adding or removing only one metal atom or ligand will result in an electronic structure variation of the clusters, thereby their properties.49–51 Interestingly, for this sequence of macrocyclic nickel clusters, Nin(4MPT)2n (n = 9–12), their electronic structures did not exhibit a significant change with the increasing Ni atom number of rings, which is evidenced by similar experimental absorption spectra and the calculated HOMO–LUMO orbitals as discussed above. We believe that the similar geometries and the same Ni–S coordination of the clusters can satisfactorily explain the similarity of their electronic structures.
Due to the similarity in their geometric and electronic structures, the clusters probably exhibit similar physicochemical properties. First, the stability of the clusters was studied. As shown in Fig. S9–Fig. S12,† the clusters are very stable under diverse conditions including high temperature as well as acidic/alkaline/reductive environments, as evidenced by the negligible changes in the UV-vis spectra under each condition. Subsequently, the excellent stability of the clusters under alkaline and reductive conditions prompted us to investigate their catalytic properties in the reduction reaction of p-nitrophenol. As shown in Fig. 5 and Fig. S13,† the reduction of p-nitrophenol occurs very fast, and p-nitrophenol is converted into p-aminophenol within 2 min. Previous studies suggest that the reaction follows a quasi-first-order kinetics.52 According to the equation, ln(c0/ct) = kappt, the slope of the line resulting from the ln(c0/ct) − t(s) plot gives the rate constant (kapp), a fundamental parameter in chemical kinetics to compare the rate of an individual reaction.53 As shown in Fig. 5b and c, the kapp values for Nin(4MPT)2n (n = 9–12) are 0.025, 0.032, 0.036, and 0.047 s−1, respectively. The clusters demonstrate excellent catalytic activity, and their kapp values exhibit an increasing trend from Ni9(4MPT)18 to Ni12(4MPT)24. In other words, the reaction becomes faster with increasing ring size. One possible explanation is that for the homologous series of macrocyclic Ni cluster catalysts, the number of active sites increases with the number of [NiS4] subunits in the clusters, and therefore a faster reaction results.
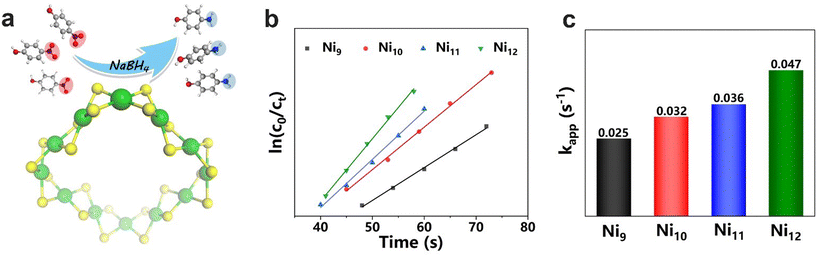 |
| Fig. 5 Catalytic properties of the Nin(4MPT)2n (n = 9–12) clusters in the reduction reaction of p-nitrophenol. (a) A schematic diagram for p-nitrophenol reduction. (b) The corresponding reaction kinetics of the reactions. (c) Reaction rate constant (kapp) of the reduction reactions catalyzed by the clusters. Color codes: yellow sphere, S; green sphere, Ni. red sphere, O; blue sphere, N; gray sphere, C; white sphere, H. | |
3. Conclusions
In summary, we, for the first time, have reported a series of macrocyclic homoleptic nickel clusters Nin(4MPT)2n (n = 9–12), and their compositions and structures were determined by using SXRD and ESI-MS. Although the clusters possess ring-like structures, the Ni10(4MPT)20 and Ni12(4MPT)24 clusters are unique and distinct from those of their reported Ni10 and Ni12 counterparts. More interestingly, the electronic structures and optical properties do not show significant changes with the increase of the Ni atoms. Finally, the reduction of p-nitrophenol catalyzed by these clusters was studied, and the clusters display excellent catalytic activity towards the reduction of p-nitrophenol. The rate constant exhibits an increasing trend from Ni9(4MPT)18 to Ni12(4MPT)24, which implies that the catalytic activity is positively correlated with the size of the Ni–S ring. The study provides a facile approach to macrocyclic homoleptic nickel clusters, and we anticipate that the work reported herein will benefit the fabrication of other metal macrocyclic homoleptic clusters consisting of group 10 transition elements, and contributes to an in-depth understanding of their structure–property correlations at the atomic level.
Author contributions
C. Y. conceived and designed the experiments. C. X. and J. L. performed quantum chemical calculations and analyzed the data. H. X. and R. C. synthesized the samples and performed the measurements. C. R., C. M., Y. G., and W. C. analyzed parts of the data and plotted the figures. Y. Z. collected and refined the crystal data of the clusters. C. Y., H. X., and C. X. wrote the paper.
Conflicts of interest
There are no conflicts to declare.
Acknowledgements
This work was financially supported by start-up research funding of Fujian Normal University, the Natural Science Foundation of Ningbo (202003N4005), the National Natural Science Foundation of China (No. 22033005 and 22103035) and National Key Research and Development Project (2022YFA1503900). Computational resources were provided by the CHEM high-performance supercomputer cluster (CHEM-HPC) and Center for Computational Science and Engineering at Southern University of Science and Technology.
References
- X. Zou, X. Kang and M. Zhu, Chem. Soc. Rev., 2023, 52, 5892–5967 RSC.
- Y. Jin, C. Zhang, X.-Y. Dong, S.-Q. Zang and T. C. W. Mak, Chem. Soc. Rev., 2021, 50, 2297–2319 RSC.
- Z. J. Guan, J. J. Li, F. Hu and Q. M. Wang, Angew. Chem., Int. Ed., 2022, 61, e202209725 CrossRef CAS PubMed.
- X. J. Wang, B. Yin, L. R. Jiang, C. Yang, Y. Liu, G. Zou, S. Chen and M. Z. Zhu, Science, 2023, 381, 784–790 CrossRef CAS PubMed.
- Y. Li, Y. Song, X. Zhang, T. Liu, T. Xu, H. Wang, D.-e. Jiang and R. Jin, J. Am. Chem. Soc., 2022, 144, 12381–12389 CrossRef CAS PubMed.
- L. Tang, B. Wang, R. Wang and S. Wang, Nanoscale, 2023, 15, 1602–1608 RSC.
- T. Jia, Z.-J. Guan, C. Zhang, X.-Z. Zhu, Y.-X. Chen, Q. Zhang, Y. Yang and D. Sun, J. Am. Chem. Soc., 2023, 145, 10355–10363 CrossRef CAS PubMed.
- Z.-M. Zhu, Y. Zhao, H. Zhao, C. Liu, Y. Zhang, W. Fei, H. Bi and M.-B. Li, Nano Lett., 2023, 23, 7508–7515 CrossRef CAS PubMed.
- Z. Zuo, K.-J. Hu, S. Lu, S. Hu, S. Tang, Y. Zhang, Z. Zhao, D. Zheng and F. Song, Nanoscale, 2023, 15, 15043–15049 RSC.
- P. D. Jadzinsky, G. Calero, C. J. Ackerson, D. A. Bushnell and R. D. Kornberg, Science, 2007, 318, 430–433 CrossRef CAS PubMed.
- F. Hu, R. L. He, Z. J. Guan, C. Y. Liu and Q. M. Wang, Angew. Chem., Int. Ed., 2023, 62, e202304134 CrossRef CAS PubMed.
- Y. Cao, Y. Xu, H. Shen, P. Pan, X. Zou, X. Kang and M. Zhu, Nanoscale, 2023, 15, 13784–13789 RSC.
- L. Fang, W. Fan, G. Bian, R. Wang, Q. You, W. Gu, N. Xia, L. Liao, J. Li, H. Deng, N. Yan and Z. Wu, Angew. Chem., Int. Ed., 2023, 62, e202305604 CrossRef CAS PubMed.
- J. Tang, C. Liu, C. Zhu, K. Sun, H. Wang, W. Yin, C. Xu, Y. Li, W. Wang, L. Wang, R. Wu, C. Liu and J. Huang, Nanoscale, 2023, 15, 2843–2848 RSC.
- C. Xu, Y. Jin, H. Fang, H. Zheng, J. C. Carozza, Y. Pan, P.-J. Wei, Z. Zhang, Z. Wei, Z. Zhou and H. Han, J. Am. Chem. Soc., 2023, 145, 25673–25685 CrossRef CAS PubMed.
- L. Li, P. Wang and Y. Pei, Nanoscale, 2022, 14, 5694–5700 RSC.
- G. Yang, X. Pan, W. Feng, Q. Yao, F. Jiang, F. Du, X. Zhou, J. Xie and X. Yuan, ACS Nano, 2023, 17, 15605–15614 CrossRef CAS PubMed.
- P. Woodward, L. F. Dahl, E. W. Abel and B. C. Crosse, J. Am. Chem. Soc., 1965, 87, 5251–5253 CrossRef CAS.
- E. W. Abel and B. C. Crosse, J. Chem. Soc. A, 1966, 1377–1378 RSC.
- R. Angamuthu, H. Kooijman, M. Lutz, A. L. Spek and E. Bouwman, Dalton Trans., 2007, 4641–4643 RSC.
- M. Zhu, S. Zhou, C. Yao, L. Liao and Z. Wu, Nanoscale, 2014, 6, 14195–14199 RSC.
- C. Tan, M. Jin, H. Zhang, S. Hu, T. Sheng and X. Wu, CrystEngComm, 2015, 17, 5110–5115 RSC.
- D. R. Kauffman, D. Alfonso, D. N. Tafen, J. Lekse, C. Wang, X. Deng, J. Lee, H. Jang, J.-s. Lee, S. Kumar and C. Matranga, ACS Catal., 2016, 6, 1225–1234 CrossRef CAS.
- X. Chai, T. Li, M. Chen, R. Jin, W. Ding and Y. Zhu, Nanoscale, 2018, 10, 19375–19382 RSC.
- M. P. Maman, T. Gurusamy, A. K. Pal, R. Jana, K. Ramanujam, A. Datta and S. Mandal, Angew. Chem., Int. Ed., 2023, 62, e202305462 CrossRef CAS PubMed.
- H. Seong, J. Kim, K. Chang, H.-w. Kim, W. Choi and D. Lee, J. Electrochem. Sci. Technol., 2023, 14, 243–251 CrossRef CAS.
- A. Datta, N. S. John, G. U. Kulkarni and S. K. Pati, J. Phys. Chem. A, 2005, 109, 11647–11649 CrossRef CAS PubMed.
- H. N. Kagalwala, E. Gottlieb, G. Li, T. Li, R. Jin and S. Bernhard, Inorg. Chem., 2013, 52, 9094–9101 CrossRef CAS PubMed.
- K. S. Joya, L. Sinatra, L. G. AbdulHalim, C. P. Joshi, M. N. Hedhili, O. M. Bakr and I. Hussain, Nanoscale, 2016, 8, 9695–9703 RSC.
- S. Srinivasan, Z. Liu, S. House and R. Jin, Inorg. Chem., 2022, 62, 1875–1884 CrossRef PubMed.
- S. Funaki, T. Kawawaki, T. Okada, K. Takemae, S. Hossain, Y. Niihori, T. Naito, M. Takagi, T. Shimazaki, S. Kikkawa, S. Yamazoe, M. Tachikawa and Y. Negishi, Nanoscale, 2023, 15, 5201–5208 RSC.
- A. J. Touchton, G. Wu and T. W. Hayton, Small, 2021, 17, 2003133 CrossRef CAS PubMed.
- A. H. Mahmoudkhani and V. Langer, Polyhedron, 1999, 18, 3407–3410 CrossRef CAS.
- C. Tan, M. Jin, X. Ma, Q. Zhu, Y. Huang, Y. Wang, S. Hu, T. Sheng and X. Wu, Dalton Trans., 2012, 41, 8472–8476 RSC.
- T. Krüger, B. Krebs and G. Henkel, Angew. Chem., Int. Ed., 2003, 28, 61–62 CrossRef.
- Q. You, X.-L. Jiang, W. Fan, Y.-S. Cui, Y. Zhao, S. Zhuang, W. Gu, L. Liao, C.-Q. Xu, J. Li and Z. Wu, Angew. Chem., Int. Ed., 2023, e202313491 Search PubMed.
- S. Havenridge and C. M. Aikens, J. Phys. Chem. A, 2023, 127, 9932–9943 CrossRef CAS PubMed.
- I. G. Dance, M. L. Scudder and R. Secomb, Inorg. Chem., 1985, 24, 1201–1208 CrossRef CAS.
- T. Kruger, B. Krebs and G. Henkel, Angew. Chem., Int. Ed. Engl., 1989, 28, 61–62 CrossRef.
- S. A. Ivanov, M. A. Kozee, W. A. Merrill, S. Agarwal and L. F. Dahl, J. Chem. Soc., Dalton Trans., 2002, 4105–4115 RSC.
- C. Zhang, T. Matsumoto, M. Samoc, S. Petrie, S. Meng, T. C. Corkery, R. Stranger, J. Zhang, M. G. Humphrey and K. Tatsumi, Angew. Chem., Int. Ed., 2010, 49, 4209–4212 CrossRef CAS PubMed.
- O. L. Sydora, P. T. Wolczanski, E. B. Lobkovsky, E. Rumberger and D. N. Hendrickson, Chem. Commun., 2004, 650–651 RSC.
- H. Xiang, H. Yan, J. Liu, R. Cheng, C. Q. Xu, J. Li and C. Yao, J. Am. Chem. Soc., 2022, 144, 14248–14257 CrossRef CAS PubMed.
- C. Yao, C. Q. Xu, I. H. Park, M. Zhao, Z. Zhu, J. Li, X. Hai, H. Fang, Y. Zhang, G. Macam, J. Teng, L. Li, Q. H. Xu, F. C. Chuang, J. Lu, C. Su, J. Li and J. Lu, Angew. Chem., Int. Ed., 2020, 59, 8270–8276 CrossRef CAS PubMed.
- C. J. Zeng, C. Y. Liu, Y. Pei and R. C. Jin, ACS Nano, 2013, 7, 6138–6145 CrossRef CAS PubMed.
- H. Yan, H. Xiang, J. Liu, R. Cheng, Y. Ye, Y. Han and C. Yao, Small, 2022, 18, e2200812 CrossRef PubMed.
- C. Yao, N. Guo, S. Xi, C.-Q. Xu, W. Liu, X. Zhao, J. Li, H. Fang, J. Su, Z. Chen, H. Yan, Z. Qiu, P. Lyu, C. Chen, H. Xu, X. Peng, X. Li, B. Liu, C. Su, S. J. Pennycook, C.-J. Sun, J. Li, C. Zhang, Y. Du and J. Lu, Nat. Commun., 2020, 11, 4389 CrossRef CAS PubMed.
- C. Ruan, H. Xiang, H. Yan, Y. Deng, Y. Zhao, C. Q. Xu, J. Li and C. Yao, Small, 2023, 19, 2305056 CrossRef CAS PubMed.
- C. Dong, R. W. Huang, A. Sagadevan, P. Yuan, L. Gutiérrez-Arzaluz, A. Ghosh, S. Nematulloev, B. Alamer, O. F. Mohammed, I. Hussain, M. Rueping and O. M. Bakr, Angew. Chem., Int. Ed., 2023, 62, e202307140 CrossRef CAS PubMed.
- Y. Li, H. K. Kim, R. D. McGillicuddy, S.-L. Zheng, K. J. Anderton, G. J. Stec, J. Lee, D. Cui and J. A. Mason, J. Am. Chem. Soc., 2023, 145, 9304–9312 CrossRef CAS PubMed.
- C. Yao, Y.-j. Lin, J. Yuan, L. Liao, M. Zhu, L.-h. Weng, J. Yang and Z. Wu, J. Am. Chem. Soc., 2015, 137, 15350–15353 CrossRef CAS PubMed.
- Z. J. Guan, R. L. He, S. F. Yuan, J. J. Li, F. Hu, C. Y. Liu and Q. M. Wang, Angew. Chem., Int. Ed., 2022, 61, e202116965 CrossRef CAS PubMed.
- S. Song, Y. Wang, H. Shen, J. Zhang, H. Mo, J. Xie, N. Zhou and J. Shen, ACS Appl. Nano Mater., 2019, 2, 7074–7084 CrossRef CAS.
Footnotes |
† Electronic supplementary information (ESI) available. CCDC 2307845 (for Ni9(4MPT)18), 2307689 (for Ni10(4MPT)20), 2307690 (for Ni11(4MPT)22), and 2308123 (for Ni12(4MPT)24). For ESI and crystallographic data in CIF or other electronic format see DOI: https://doi.org/10.1039/d3nr06015b |
‡ These authors contributed equally to this work. |
|
This journal is © The Royal Society of Chemistry 2024 |
Click here to see how this site uses Cookies. View our privacy policy here.