DOI:
10.1039/D4NR01011F
(Paper)
Nanoscale, 2024,
16, 13050-13060
A hydrogel based on Fe(II)-GMP demonstrates tunable emission, self-healing mechanical strength and Fenton chemistry-mediated notable antibacterial properties†
Received
10th March 2024
, Accepted 6th June 2024
First published on 6th June 2024
Abstract
Supramolecular hydrogels serve as an excellent platform to enable in situ reactive oxygen species (ROS) generation while maintaining controlled localized conditions, thereby mitigating cytotoxicity. Herein, we demonstrate hydrogel formation using guanosine-5′-monophosphate (GMP) with tetra(4-carboxylphenyl) ethylene (1) to exhibit aggregation-induced emission (AIE) and tunable mechanical strength in the presence of divalent metal ions such as Ca2+, Mg2+, and Fe2+. The addition of divalent metal ions leads to structural transformation in the metallogels (M-1GMP). Furthermore, the incorporation of Fe2+ ions into the hydrogel (Fe-1GMP) promotes the Fenton reaction that could be upregulated upon adding ascorbic acid (AA), demonstrating antibacterial efficacy via ROS generation. In vitro studies on AA-loaded Fe-1GMP demonstrate excellent bacterial killing efficacy against E. coli, S. aureus and vancomycin-resistant enterococci (VRE) strains. Finally, in vivo studies involving topical administration of Fe-1GMP to Balb/c mice with skin infections further suggest the potential antibacterial efficacy of the hydrogel. Taken together, the hydrogel with its unique combination of mechanical tunability, ROS generation capability and antibacterial efficacy can be used for biomedical applications, particularly in wound healing and infection control.
Introduction
Bacterial infections have far-reaching consequences for global health, constituting a significant burden on healthcare systems and necessitating continuous efforts in research and treatment.1 The predominant approach in the therapeutic management of bacterial infections has so far been through antibiotic treatment. Yet, the irrational and rampant use of antibiotics is leading to the threat of antimicrobial resistance (AMR) due to the bacteria developing self-mechanisms to resist the action of these pharmacological agents.2,3 Of late, there has been an upsurge in the development of alternative and effective treatment strategies to mitigate such threats.4 In this regard, hydrogels have evolved as promising candidates to mitigate bacterial infections, especially in wound healing, tissue engineering and other disease conditions.5–9 These nanostructured materials can possess inherent antibacterial activity or can be utilized toward the targeted and controlled release of antimicrobial drugs, enhancing both specificity and potency in combating bacterial infections.10–15 Moreover, hydrogels due to their cargo loading efficacy, mechanical strength, response to different stimuli and wettability have evolved as a primary tool to be employed in various strategies of microbial management.15 These strategies include loading antibacterial drugs into hydrogels for controlled release at infected sites, utilizing active antibacterial agents as gelators that can form gels through interactions with other molecules and developing hydrogels capable of exhibiting photothermal therapy (PTT) or photodynamic therapy (PDT).16–18
Recently, such biomaterials and hydrogels have been developed for the prevention of bacterial infections in wound healing while addressing challenges of AMR.19–21 Such hydrogels primarily act as carrier systems for delivering antimicrobial agents or as templates for ROS production to control bacterial infections. Through the localized delivery of antibiotics or ROS, hydrogels minimize the exposure of bacteria to the sublethal concentrations of these agents in a bid to mitigate the development of bacterial resistance. Therefore, hydrogels offer a promising approach for combating bacterial infections while minimizing the risk of resistance development. Haldar et al. developed a polymeric hydrogel-based wound dressing that shows a broad spectrum of bactericidal activities and potent wound healing capability.22 In another approach, Bajaj et al. developed a cholic acid-derived non-immunogenic supramolecular hydrogel for the treatment of Staphylococcus aureus infections in topical wounds.23 Recently, peptide-based hydrogels showed great promise as potential antimicrobial and antibiofilm agents to address chronic wound healing owing to the presence of charged amino acids, e.g. arginine and lysine, and conjugation of anti-inflammatory drugs.24–27 In another strategy, Chatterjee et al. have reported the effect of the hydrophobic side chain length of peptides on antibacterial activity and cytotoxicity.28 Stupp et al. reported self-assembled peptide nanofiber-anchored silver nanoparticles in organic–inorganic hybrids to exhibit robust antimicrobial properties.29
Recently, we also reported a strategy to mitigate bacterial multidrug resistance by forming a charge transfer complex between the donor–acceptor pair of peptide-tethered pyrene and tetracyanoquinodimethane to inhibit bacterial growth owing to its high charge mobility to perturb the electrochemical potential of bacterial cell membranes.30 In this regard, PDT has emerged as a targeted and minimally invasive approach with fewer side effects and high cure rates in the treatment of certain types of cancer, skin diseases and microbial infections.31,32 This involves a photosensitizing agent that absorbs light and generates ROS to stimulate oxidative stress and ultimately trigger a cascade of events leading to cell death. Reactive oxygen species include the superoxide anion (O2˙−), singlet oxygen (1O2) and the hydroxyl radical (˙OH). Srivastava et al. reported temporal ROS generation in phenylalanine self-assembled hydrogel composites for anticancer applications.33 Recently, Zheng et al. showcased antibacterial PDT by generating 1O2 using a boron dipyrromethene photosensitizer.34 Such ROS generation can also be mitigated by multiple chemical reactions such as oxidative phosphorylation, NADPH oxidases, the heme oxygenase reaction and the Fenton reaction.35 The Fenton reaction is a chemical reaction involving the generation of ROS, specifically hydroxyl radicals, through the interaction of hydrogen peroxide (H2O2) with Fe2+ ions.36 The amount of H2O2 available for oxidation may also account for the production of ˙OH.37 Harnessing the Fenton reaction to address microbial contamination holds promise for diverse applications, including water decontamination and the formulation of antimicrobial coatings.38,39
However, challenges, such as the need for controlled ROS generation with minimal H2O2 usage and considerations of cytotoxicity, must be addressed to mitigate microbial infections.
Thus, achieving precise spatiotemporal control over ROS generation is crucial for achieving their biomedical application.40 Supramolecular hydrogels,41–43 especially metallogels, offer an ideal platform for this purpose, providing a biologically relevant and adjustable nanostructure capable of accommodating the Fenton reaction. Shen et al. reported photothermally enhanced ˙OH radical production towards crosslinking hydrogels and subsequent bacteria-infected diabetic wound management.44 However, a rational control over the crosslinking of hydrogels for hosting such a Fenton reaction is rather limited. This prompted us to develop biocompatible supramolecular hydrogels with tuneable architectures and properties for ROS generation.
Herein, we envisaged guanosine monophosphate (GMP)-based hydrogels as driven by the extensive Hoogsteen-type hydrogen bonding among the guanosine nucleobases to facilitate metal ion stabilized G-quadruplex self-assembled structures.45,46 Herein, we demonstrate additional hydrogen bonding interactions of a GMP-based G-quadruplex with tetravalent tetra(4-carboxylphenyl) ethylene (1) to generate a 1GMP hydrogel (Scheme 1). Such additional cross-linking presumably reinforces the G-quadruplex secondary structures to form hydrogels and exhibit AIE behavior. Interestingly, upon binding with divalent metal ions, the change in secondary structures modulates the AIE and increases the mechanical strength of the resulting metallogels (M-1GMP). Furthermore, we exploited the Fe-1GMP hydrogel for in situ ROS generation using the Fenton reaction in the presence of H2O2, which was upregulated upon addition of ascorbic acid (AA). To our knowledge, hosting the Fenton reaction in metallogels for controlled evolution of ROS has so far not been attempted. We showed efficient antibacterial efficacy against E. coli, S. aureus and vancomycin-resistant enterococci (VRE) mediated by ROS generation in the Fe-1GMP hydrogel. Finally, we validated the antibacterial efficacy of the hydrogel hosting Fenton reaction against E. coli in an in vivo Balb/C mouse model.
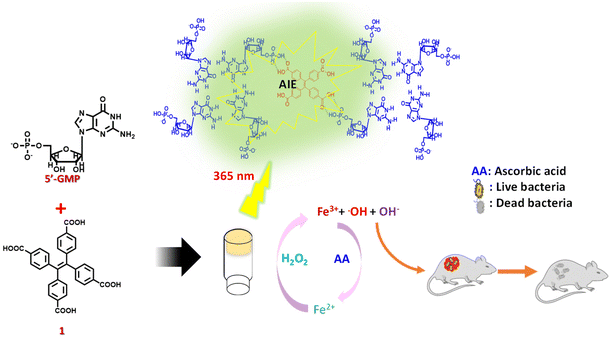 |
| Scheme 1 Supramolecular hydrogels mediated by the crosslinking of GMP with 1 and divalent metal ions (M) as in M-1GMP to exhibit aggregation-induced emission. The Fe-1GMP hydrogel exhibits upregulation of the Fenton reaction in the presence ascorbic acid to generate ROS for eventual application in controlling bacterial growth in topical wounds. | |
Experimental section
Preparation of the hydrogel (1GMP)
A powdered form of GMP (12 mg) was taken in 500 μL of 60 mM acetate buffer at pH 4 and heated to complete dissolution to obtain a GMP (60 mM) solution of pH 4. Subsequently, various concentrations (1 mM to 9 mm) of tetra(4-carboxylphenyl) ethylene (1) in ethanol was added to the GMP solution, followed by heating at 80 °C for 10 min and then gradual cooling which ensued gelation. We noticed that only an optimum concentration range of 1 (4.5 to 6 mM) furnished strong hydrogels of 1GMP.
For the preparation of metallogels (M-1GMP), divalent metal salt solutions of Ca2+, Mg2+, and Fe2+ (1 mM) were added to the hot solution of 1GMP at 70 °C, followed by subsequent gradual cooling to room temperature. The metallogel formation was optimized by varying the ratio of metal ions w.r.t. 1GMP.
Spectral studies of the hydrogels
The samples were prepared by drop-coating 100 μL of the hydrogel on a quartz slide for circular dichroism and fluorescence spectral studies. Fluorescence spectra were recorded with an Edinburgh Instruments spectrofluorometer FS5. Circular Dichroism (CD) spectra were recorded using a JASCO J-1500 circular dichroism spectrometer from Easton, MD, USA. Samples for 1H-NMR analysis were prepared from the lyophilized hydrogel powder after suspending it in D2O and 1H-NMR spectra were recorded using an FT-NMR Bruker 400 MHz NMR spectrometer.
Rheology of the hydrogels
Rheological assessments were carried out using an Anton Paar rheometer MCR302 device equipped with Rheocompass version 1.31 software and a cone plate (CP-25) configuration featuring a controllable Peltier system. The measurements were performed with a 0.2 mm gap distance.
ROS generation
The Fe-1GMP hydrogel (0.6 mM) was taken with and without AA in 1000 μL of acetate buffer at pH 4. Methylene blue (MB) solution (0.1 mM) in water was added to record the UV-visible absorption spectra. Furthermore, H2O2 was gradually added (ranging from 0.05 mM to 50 mM) to monitor the reduction in the intensity of absorbance due to MB. The percentage degradation of dye was calculated by taking the absorbance of the hydrogel with MB before adding H2O2 as 100%.
In vitro antimicrobial studies
Antimicrobial properties were evaluated against the strains of bacteria, E. coli and S. aureus that were grown overnight in Luria–Bertani (LB) at 37 °C to a final bacterial concentration of 3 × 106 CFU mL−1. For drug-resistant bacterial strain, Enterococcus faecium was grown in brain heart infusion broth (BHIB) media at 37 °C to a final concentration of 5 × 106 CFU mL−1. Then, 100 μL of the bacterial stock was suspended in 2 mL of the corresponding medium. The hydrogels were sterilized under UV light. In the test samples, H2O2 was injected just before adding the hydrogels to the media containing bacteria. A control with only H2O2 was also taken for comparison. The treated samples were kept in a BOD incubator at 37 °C and thoroughly shaken for 16 h. Then, 100 μL of suspension was taken as an aliquot from each sample and diluted accordingly using autoclave water. Furthermore, 100 μL of this diluted suspension was spread on the LB agar, BHI agar plates were incubated at 37 °C for another 14 h before taking the agar plates for visualizing bacterial colonies. For comparison, the microbial assay was conducted taking silver sulfadiazine as a standard. Furthermore, for quantification, colony forming units per mL (c.f.u per mL) of bacterial cells was calculated.
Cellular compatibility of the hydrogels
The biocompatibility and cellular proliferation ability of the hydrogels (Fe-1GMP and Fe-1GMP+AA) were assessed using mouse fibroblast L929 cell lines in a cytotoxicity assay. The cells were individually cultured in Dulbecco's Modified Eagle's Medium (DMEM, Cytiva) supplemented with 10% fetal bovine serum (FBS) (Gibco, Thermo Fisher Scientific) at 37 °C and 5% CO2. Culturing was performed in 25 cm cell culture flasks from Cole-Parmer and the cells were passaged upon reaching 60–70% confluency. Biocompatibility tests with different concentrations of Fe-1GMP and Fe-1GMP+AA were performed using 3-(4,5-dimethylthiazol-2-yl)-2,5-diphenyltetrazolium bromide in the MTT assay. 5000 L929 cells per well were seeded with DMEM (HIMEDIA) supplemented with 10% FBS in a 96-well plate. After overnight incubation, different concentrations of the media containing Fe-1GMP and Fe-1GMP+AA were treated for 48 h at 37 °C, followed by the addition of MTT at a concentration of 0.5 mg mL−1 and incubated for 2 h. The absorbance of DMSO-solubilized formazan crystals was measured at 570 nm using a BIOTEK multiplate reader. The assay was performed in triplicate for each sample.
In vivo antimicrobial study on rat skin
Animal experiments, approved by the Institutional Animal Ethics Committee, were conducted following the ethical guidelines (no. IACS/IAEC/2018/04). For the in vivo study, male Balb/c mice aged around 6–8 weeks and weighing 20–25 g were chosen. These mice were procured from the National Institute of Nutrition (NIN) animal facility in Hyderabad. Neutropenia was induced in all mice by dual administration of cyclophosphamide monohydrate (TCI chemicals) via intraperitoneal injection with both doses given at a concentration of 100 mg per kg body weight (the second dose was administered 3 days after the first). After 24 hours from the second dose, the mice were taken out and the hair around the dorsal midline was trimmed and removed using hair removal cream. The region was then made aseptic by rubbing with 70% ethanol. For the study, a pathogenic strain of E. coli was prepared following the LB broth method. The bacterial solution was incubated overnight. After centrifugation, the bacterial pellet was dissolved in sterile 1× PBS buffer (100 μL).
Furthermore, dorsal surfaces of the mice were mildly wounded to create a skin abrasion. The bacterial solution (100 μL) was added to this surface and secured with a sterile gauge and band-aids on all sides to allow aeration for the inoculated bacterial biomass to colonize the skin and form biofilms. After 24 hours of undisturbed incubation, the hydrogel formulations (four different sets: 1GMP without AA, only H2O2, 1GMP without AA and H2O2, and 1GMP with AA and H2O2) were applied on mouse skin. After 24 hours, the mice were sacrificed and the dorsal skin portions were isolated and washed with 0.9% NaCl to remove residual bacteria and gel particles.
A portion of the skin was homogenized and the bacterial biomass was dislodged. The resulting solution was centrifuged and the dislodged bacteria were obtained. The supernatant was used to determine the number of colony-forming units (CFU) per 50 mg of skin tissue. Equal volumes from each group were diluted, plated on nutrient agar and incubated at 37 °C in a BOD incubator overnight and the colonies were observed.
Another fraction of the mouse skin was homogenized and the displaced bacterial population was subjected to centrifugation using a REMI C24 plus centrifugation machine at 5000 rpm. The bacteria were stained to quantify live and dead cells employing Syto-9 (Invitrogen™ SYTO™ 9 green fluorescent nucleic acid stain) and propidium iodide (PI) (Sigma-Aldrich) in a 1
:
1 staining mixture. The concentrations of the two dyes were set at 5 μM for Syto-9 and 30 μM for propidium iodide, both prepared in 1× PBS buffer. The bacterial pellet obtained from centrifugation was dissolved in 50 μL of 1× PBS buffer containing the dyes and left to incubate in the dark for 20 min. Then a solution inoculum was placed on a clean sterile glass slide, covered with a coverslip, and kept at 4 °C for 30 minutes. Fluorescence images of the bacterial biomass were captured using an EVOS M5000 imaging system.
Results and discussion
G-quartet hydrogel formation
Guanosine 5′-monophosphate disodium salt self-assembled in acetate buffer of pH 4 into a viscous solution, presumably due to the formation G4-quartet structures as mediated by the hydrogen bonding donor–acceptor pair in guanosine bases that was further stabilized by sodium ions for G-quadruplex structures.47 However, even after repeated annealing, it did not form hydrogels, indicating the weak nature of crosslinking. Interestingly, upon addition of an ethanolic solution of tetra(4-carboxylphenyl) ethylene (1) with four carboxylic acid motifs, we observed the formation of hydrogels at an optimal concentration.48 Introduction of 1 promotes the cross-linking among the G4-quartet stacks via hydrogen bonding interactions. Each G4-quartet unit contains four phosphoric groups, while 1 possesses four carboxylic acid groups available for hydrogen bonding. This suggests the significance of the stoichiometry between the two components in the gelation process. To investigate this, gelation experiments were conducted by varying the concentration of 1, ranging from 1 mM to 9 mM (Fig. 1A). Gelation was specifically observed at 4.5 mM and 6 mM concentrations of 1. However, precipitation of 1 occurred at higher concentrations, presumably due to its limited solubility in the aqueous medium. Notably, gelation did not occur at the lower concentration of 1, indicating insufficient cross-linking to underscore the critical role of the 1 and GMP ratio in gelation. The self-assembly mechanism was further investigated through comparative 1H-NMR studies (Fig. 1B). The addition of 1 to the GMP solution in D2O resulted in a downfield shift in the proton peak (a) adjacent to the phosphate group from δ = 3.99 to 4.10 ppm which suggested the active involvement of the phosphate group in hydrogel formation through hydrogen bonding. Additionally, a downfield shift was observed in the aromatic proton peak (b′) of 1 (Fig. S1†) to provide additional confirmation for the involvement of the COOH group in hydrogen bonding interactions. Similarly, we observed a shift in the 1H-NMR peaks of the protons (a, b, c, and d) from the pentose sugar upon binding to 1. This suggested the presence of an extensive H-bonding network among the carboxylic moieties of 1 and the phosphoric groups of G4-quadruplex structures to provide additional crosslinking, leading to the formation of the 1GMP hydrogel. Furthermore, the hydrogel exhibited green emission under UV light at a wavelength of 365 nm attributable to the aggregation-induced emission (AIE) properties of 1 (Fig. 1C). The CD spectra of 1GMP exhibited negative Cotton bands at 216 and 279 nm and a positive Cotton band at 315 nm (Fig. 1D), indicating the presence of G4-quadruplex secondary structures mediated by H-bonding as corroborated from 1H-NMR. Next, the comparative mechanical properties of the hydrogels formed at different concentrations of 1 were investigated by rheological studies. The oscillatory frequency sweep experiment for hydrogel networks exhibited a frequency-independent behaviour over three orders of angular frequency with a G′ value of ∼1000 Pa and a G′/G′′ ratio of ∼4 (Fig. 1E) to indicate mechanically stiff and viscoelastic solid-like hydrogels for 1GMP.
 |
| Fig. 1 (A) Digital images of hydrogel formation at different concentrations of 1 and 60 mM GMP. (B) Comparative 1H-NMR spectra of 1 in DMSO-d6 and GMP and 1GMP in D2O, showing shifts in the peak position of the pentose sugar ring protons upon hydrogel formation. (C) Digital image of the 1GMP hydrogel showing AIE under UV light (365 nm). (D) CD spectra of 1GMP showing positive and negative CD signals, confirming the presence of the G4-quadruplex stacks of GMP. (E) Frequency sweep rheological measurements of 1GMP at different concentrations of 1. | |
Effect of metal ions in the metallogel
Next, we investigated the impact of divalent metal ions on the self-assembling properties of 1GMP. Thus, 1 mM calcium (Ca2+), iron (Fe2+) and magnesium (Mg2+) were added to 1GMP (60 mM). Interestingly, the hydrogel structures remained intact even after the addition of these divalent metal ions. Upon adding Ca2+, Mg2+ and Fe2+ to 1GMP, we observed an increase in absorbance in the ∼350 nm wavelength range in the UV-Vis spectra (Fig. S2A†). Notably, all the samples in the vial exhibited strong emission behavior under UVA (λmax = 365 nm) light (Fig. 2A inset). Furthermore, we employed fluorescence spectroscopy to investigate the role of 1 in the emission behavior of the hydrogels as compared to its monomeric state (Fig. S2B†). Thus, we observed a significant increase in the emission intensity of 1GMP hydrogels at 510 nm that could be attributed to the aggregation-induced emission (AIE) properties of 1. Moreover, increased crosslinking with metals for M-1GMP metallogels led to a further increase in the emission intensity, possibly due to the stabilization of higher order aggregated states by different metal ions (Fig. 2A). The trend of emission intensity with Ca-1GMP < Mg-1GMP < Fe-1GMP hydrogels is worth noting. Next, we subjected the Fe-1GMP hydrogel for heat–cool cycles to achieve reversible gel-sol-gel transition. Interestingly, the hydrogel exhibited reversible AIE behaviour as monitored at 510 nm with a noticeable increase in the emission intensity upon gel formation that was subsequently diminished upon heating (Fig. 2B). Furthermore, we recorded CD spectra to assess the alterations in the secondary structure of 1GMP induced by the addition of metal ions (Fig. 2C). 1GMP exhibited the characteristic CD signature of G-quadruplex; however, upon the addition of Mg2+ and Ca2+, we observed negative Cotton bands at 240 and 325 nm and a positive Cotton band at 265 nm. This clearly indicated the transformation of the G-quadruplex structures to G-triplex stacks as reported in the literature.48,49 In contrast, in the presence of Fe2+, no characteristic CD signals were observed, implying the absence of G-quartet or G-triplex stacks in the formed nanostructures.
 |
| Fig. 2 (A) Fluorescence spectroscopy exhibiting the change in the emission intensity of 1 in the hydrogels formed with different metal ions. Inset: a digital image of the hydrogels with various metal ions under UV light (365 nm). (B) Fluorescence emission of Fe-1GMP with the heating–cooling cycle showing reversible gel to sol conversion. (C) Comparison of the CD spectra of metallogels showing structural transitions from G-quadruplex structures. (D and E) Comparative 1H-NMR spectra of the M-1GMP hydrogels with different metal ions in D2O in different chemical shift ranges characteristic of the protons of GMP and 1. | |
This was further corroborated by PXRD where the peak at 2θ = 27.5° corresponding to G4-quadruplex stacking among the two adjacent vertical G–G stacks was absent for the case of the Fe2+ ion, hence indicating the absence of prominent G4-quadruplex stacks (Fig. S2C†).50 To further investigate the intricacies of the self-assembly mechanism, we performed 1H-NMR spectral analysis that highlighted the distinct characteristics of the protons in Fe-1GMP compared to other metallogels (M-1GMP) (Fig. 2D and E). Thus, the protons from the nucleobase (f) and the pentose sugar (e) peaks display a downfield shift and a broadening for Fe-1GMP in the case of Fe-1GMP, suggesting the coordination of Fe2+ ions with the nucleobase group (Scheme 2). Additionally, the aromatic proton peak (b′) of 1 showed a slight upfield shift in Fe-1GMP, indicating the potential involvement of molecule 1 in facilitating the self-assembly process. Furthermore, the pentose ring protons exhibited a broader nature in Fe-1GMP (Fig. S2D†), suggesting the absence of G4-quadruplex stacks within the Fe-1GMP assembly as corroborated from the observations of the CD spectral and PXRD data. FE-SEM showed the presence of a nano-fibrillar network of the Fe-1GMP hydrogel network (Fig. S2E†).
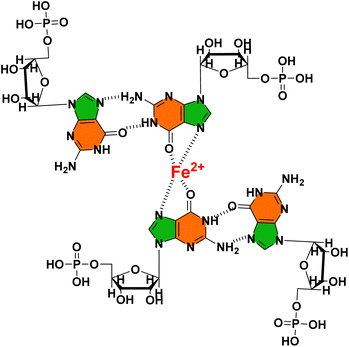 |
| Scheme 2 Schematic illustration of the possible interaction of Fe2+ with 5′-GMP in the Fe-1GMP hydrogel. | |
Tunable mechanical strength in metallogels (M-1GMP)
Next, we compared the bulk mechanical properties of the metallogels (M-1GMP) using frequency sweep oscillatory rheological studies (Fig. 3A). As compared to the native 1GMP hydrogel, all the metallogels showed higher G′ values and frequency-independent behaviour, indicating the reinforced mechanical strength upon adding divalent ions. However, no gel formation was observed when GMP and 1 were individually mixed with the metal ions (Fig. S3A†). We noted the following trend with the increase of storage moduli (G′): 1GMP < Ca-1GMP < Fe-1GMP < Mg-1GMP (Fig. 3B). We further varied the concentration of Fe2+ to optimize the metal ion : 1GMP ratio to improve the strength of the Fe-1GMP hydrogel. We performed frequency sweep oscillatory measurement on the hydrogels for varied concentrations of Fe2+ (Fig. 3C) and found a maximum mechanical strength attained at a concentration of 2 mM Fe2+ with GMP (60 mM) and 1 (4.5 mM). We investigated the reversibility of the Fe-1GMP hydrogel by subjecting the hydrogel to five consecutive heat–cool cycles. Thus, the gel was heated to 70 °C to convert it into a sol state, followed by sonication for 2 min and gradual cooling at room temperature. We noticed consistent hydrogel formation within 3–5 min after each cycle, confirming the reversible nature of Fe-1GMP (Fig. S3B†). Next, we subjected the Fe-1GMP hydrogel to dynamic thixotropic studies involving alternating cycles of high deforming shear (strain 200% at 5 rad s−1), followed by recovery (strain 1% at 5 rad s−1). The Fe-1GMP hydrogel exhibited 94% recovery, confirming its self-healing behavior (Fig. 3D).51–53 Thus, combining such a mechanical response of the Fe-1GMP hydrogel with the multiple oxidation states of Fe, this hydrogel emerged as a promising candidate to host the Fenton chemistry in a controllable manner.
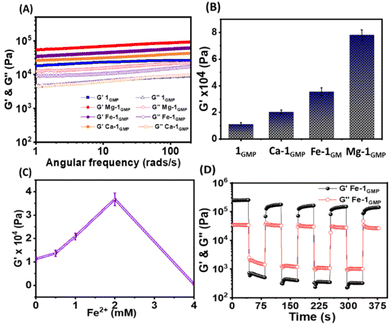 |
| Fig. 3 (A) Frequency sweep oscillatory rheology for the M-1GMP metallogels and the native 1GMP hydrogel and (B) the corresponding bar diagram showing storage moduli, G′, upon addition of different metal ions. (C) Storage moduli of Fe-1GMP with varying concentrations of the Fe2+ ion. (D) Dynamic thixotropy study of the Fe-1GMP hydrogel performed at a constant angular frequency of 5 rad s−1 and a temperature of 25 °C with alternating low and high strains of 0.1 and 100%, respectively. | |
ROS generation via the Fenton reaction
It occurred to us that the metallohydrogels (M-1GMP) with M in multiple oxidation states could participate in the Fenton reaction, albeit in a diffusion-controlled manner in the hydrogel. Thus, we explored the Fe-1GMP hydrogel's ability to trigger the Fenton reaction to generate reactive oxygen species, e.g. hydroxyl radicals (˙OH) that might have potential antibacterial properties (Fig. 4A). In the Fenton reaction, Fe2+ in the hydrogel might get oxidized to Fe3+ by H2O2 to generate a hydroxyl radical and an anion. We probed such ˙OH generation in a dye degradation experiment by monitoring the characteristic absorbance of methylene blue (MB) dye at 667 nm in the UV-visible spectra. MB dye was added to the Fe-1GMP hydrogel; however, gradual addition of H2O2 solution in the concentration range of 0.05 mM–50 mM in Fe-1GMP exhibited a decrease in the dye absorbance (Fig. 4B and S4A†). Such reduction in the absorbance indicated the degradation of MB dye, signifying in situ generation of ROS mediated by the Fenton reaction within Fe-1GMP (Fig. 4C). A control study performed to show the stability of the MB dye in the presence of only H2O2 without the Fe-1GMP hydrogel clearly confirmed the specificity of ROS generation (Fig. S4B†). Moreover, we did not observe such ROS generation in other metallogels, M-1GMP with Ca2+ and Mg2+ that indicated the significant of Fe2+ in the network (Fig. S4C and D†). Furthermore, in a bid to ramp up the ROS generation, we incorporated 10 mM ascorbic acid (AA, C6H8O6) in the Fe-1GMP hydrogel network. Addition of AA did not affect the hydrogel formation and mechanical properties of the hydrogels as shown by the rheological studies for both Fe-1GMP and Fe-1GMP + AA with G′ values of ∼20 kPa (Fig. S5A and B†). Ascorbic acid played a crucial role in the reduction of Fe3+ (ferric iron) to Fe2+ (ferrous iron), resulting in the conversion and promoting the production of ROS. The oxidized form of ascorbic acid, dehydroascorbic acid (DHA), was produced as a result of the redox reaction after the addition of H2O2 (Fig. 4D). We monitored the ROS generation and MB degradation using UV-vis spectra with a significant decrease in the absorbance upon addition of a low concentration (0.05 mM) of H2O2 (Fig. 4E).
 |
| Fig. 4 (A) Schematic representation of the Fenton reaction illustrating the conversion of ferrous ions (Fe2+) and hydrogen peroxide (H2O2) into ferric ions (Fe3+), hydroxyl radicals (˙OH), and hydroxide ions (OH−). (B) UV-vis spectra depicting changes in the absorption of the MB dye following successive additions of H2O2 solution to Fe-1GMP. (C) Comparison with respect to dye degradation in different hydrogels. (D) Schematic illustration of the role of ascorbic acid in increasing the production of reactive oxygen species (ROS). (E) UV-vis spectra showing enhanced degradation of MB after the addition of AA to Fe-1GMP. (F) Comparison of the percentage dye degradation of Fe-1GMP and Fe-1GMP + AA by addition of 0.05 mM H2O2. | |
Thus, Fe-1GMP alone exhibited only 10% dye degradation while Fe-1GMP with ascorbic acid showed 45% of dye degradation with the same H2O2 concentration (Fig. 4F). The control MB dye with H2O2 showed only 1% degradation of the dye that suggested the significantly enhanced efficacy of ROS generation in the presence of AA within the Fe-1GMP system. Interestingly, the formation of Fe3+ resulted in the weakening of the gel, as observed from the rheological studies (Fig. S5C†). A substantial decrease in the storage modulus (G′) value was observed, decreasing from 35 kPa to 7.7 kPa that was even lower than the G′ value of the gel without the addition of ions (11.1 kPa). This suggested that the mechanical strength of the hydrogel decreased significantly upon the formation of Fe3+ ions.
In vitro antibacterial efficacy
The successful demonstration of the efficient in situ ROS generation and its upregulation prompted us to assess the antimicrobial properties in vitro. To comprehensively evaluate the broad spectrum of activity against both Gram-positive and Gram-negative strains, we employed Staphylococcus aureus (S. aureus) and Escherichia coli (E. coli) strains. Furthermore, to evaluate the efficacy of our hydrogel system against drug resistant bacteria, we performed an antibacterial assay against vancomycin-resistant enterococci (Fig. 5C). We cultured the bacterial strains in the presence of H2O2 as a control and H2O2 with 1GMP. The efficiency of the Fenton reaction was evaluated by culturing Fe-1GMP with H2O2 and Fe-1GMP with H2O2 + AA for 16 hours on agar plates. Fig. 5A–C show substantial growth of bacterial colonies for the control sample with H2O2 and the 1GMP hydrogel with H2O2, indicating no significant bacterial killing in these samples. Remarkably, we observed a significant decrease in bacterial colony growth for Fe-1GMP with H2O2 for all the three bacterial strains. It is noteworthy that the clinical standard for disinfecting wounds typically involves the use of 3% H2O2.54 In our study, we opted for a lower concentration (0.5%) of H2O2 for bacterial killing. Furthermore, we quantified the antimicrobial efficacy by calculating the c.f.u per mL (Fig. 5D).55 Interestingly, we found 100% killing of the bacterial strains with Fe-1GMP with H2O2 + AA as compared to the other controls: only H2O2, 1GMP with H2O2 and Fe-1GMP with H2O2 (Fig. 5D). These observations underscore the efficient antibacterial efficacy of the Fe-1GMP hydrogel via ROS generation and its upregulation by addition of ascorbic acid, thereby highlighting its potential for antimicrobial applications. Furthermore, we compared our hydrogel system with a broad spectrum topical antibiotic drug, silver sulfadiazine, at its minimum inhibitory concentration (MIC), which showed ∼50% bacterial killing with both E. coli and S. aureus strains (Fig S6A and B†).56 In this regard, the Fe-1GMP hydrogel with H2O2 + AA showed better antibacterial effect as compared to the topical antibiotics. Recently, Gao et al. reported enzymatic cascade micro reactors for the production of hydroxyl radicals to test their activity against multi-drug resistant S. aureus.57 Therefore, our iron-complexed hydrogel system exhibited potent antibacterial activity not only against the Gram-positive and Gram-negative bacteria, but also remained effective against the drug-resistant strain of vancomycin-resistant enterococci. Finally, we evaluated the cytotoxicity of the Fe-1GMP and Fe-1GMP + AA hydrogels in the L929 mouse fibroblast cell line. The quantitative evaluation conducted through the MTT assay revealed more than 80% biocompatibility for both Fe-1GMP and AA-loaded Fe-1GMP after a 48 h incubation period (Fig. S6E†).For the control, L929 cells were cultured on a tissue culture plate. The higher survival rate of the cells indicates the biocompatibility of the hydrogel. These findings indicate minimal cytotoxic effects on the mammalian cells, thereby enhancing the potential applicability of the Fe-1GMP hydrogel in biomedical settings.
 |
| Fig. 5 Images showing the growth of bacterial colonies of (A) E. coli (upper panel), (B) S. aureus (middle panel) and (C) vancomycin-resistant enterococci (VRE) bacterial strains (lower panel) for the control samples (only H2O2) and treated samples of the 1GMP hydrogel with H2O2, the Fe-1GMP hydrogel with H2O2 and the AA-loaded Fe-1GMP hydrogel with H2O2. (D) The corresponding quantitative colony forming unit (c.f.u) per mL of S. aureus, E. coli and the resistant strain of VRE. | |
In vivo antibacterial efficacy
Furthermore, we conducted an in vivo study on male Balb/c mice with dorsal skin infections caused by E. coli to successfully demonstrate the applicability of the hydrogel as a potential antibacterial agent. The mice were induced with neutropenia by dual administration of a cyclophosphamide drug, followed by creation of skin abrasion at the dorsal midline. The wound site was infected with the pathogenic strain of E. coli using a band-aid and incubated for 1 day. The mice were divided in four sets and treatment was given using control (only H2O2), 1GMP with H2O2, Fe-1GMP with H2O2 and Fe-1GMP with H2O2 + AA (Fig. 6A). The next day, the mice were sacrificed and the dorsal skin portions were investigated for bacterial growth. Fig. 6B shows the maximum bacterial colonization for the control mice treated with only H2O2 and 1GMP with H2O2. Interestingly, mice treated with Fe-1GMP with H2O2 exhibited a significant decrease in bacterial colonization while mice treated with Fe-1GMP + AA and H2O2 showed the highest killing efficiency, thereby successfully demonstrating the antibacterial efficacy of the hydrogel via ROS generation.
 |
| Fig. 6 (A) Schematic illustration showing the detailed day wise protocol for in vivo studies on Balb/c mice. (B) Antibacterial activity of Fe-1GMP and AA-loaded Fe-1GMP showing the growth of bacterial colonization in different samples. (C) Fluorescence microscopy images showing the active bacterial cells emitting green when stained with SYTO-9 dye and the dead cells emitting red when stained with PI. | |
Furthermore, the bacteria were stained to quantify live and dead cells, employing Syto-9 (green fluorescent nucleic acid stain) and propidium iodide (PI) in a 1
:
1 staining mixture. Fluorescence microscopy images provided insights into the growth of bacterial cells with live cells stained with SYTO-9 and dead cells stained with propidium iodide (PI). The results highlighted the maximum green emission for the control samples (Fig. 6C). However, in the case of AA-loaded Fe-1GMP with H2O2 treatment, the green fluorescence signal of SYTO-9 was significantly less with a bright red emission from PI signifying the dead bacterial cells. Such a decrease in green fluorescence and an increase in red fluorescence suggest a substantial decrease in the live bacterial cells and enhanced antibacterial properties of the hydrogels via ROS generation. Thus, these findings not only supported, but also visually illustrate the potential of the hydrogel for use as an effective antibacterial agent through topical administration to the infected sites.
Conclusions
In conclusion, we have successfully designed an interesting 1GMP hydrogel system based on the extensive hydrogen bonding and stacking interaction between guanosine 5′-monophosphate (GMP) and tetra(4-carboxylphenyl) ethylene (1). Extensive characterization through various techniques like 1H-NMR, CD and rheology provided insights into the self-assembly mechanism and mechanical properties of the hydrogels. The hydrogel exhibited tunable aggregation-induced emission properties and mechanical strength upon incorporation of various divalent metal ions such as Ca2+, Mg2+ and Fe2+ to form mechanically stiffer hydrogels of M-1GMP. Furthermore, the Fe-1GMP metallogel was investigated for the in situ generation of reactive oxygen species via the Fenton reaction that was upregulated upon addition of ascorbic acid. Taken together, the hydrogel's capacity to generate hydroxyl radicals was demonstrated along with its reformability and thixotropic nature to enhance its versatility. Furthermore, owing to such ROS generation, Fe-1GMP exhibited efficient in vitro antibacterial properties against E. coli, S. aureus and vancomycin-resistant enterococci. Notably, the Fe-1GMP hydrogel loaded with ascorbic acid exhibited the highest killing efficiency. Finally, in vivo experiments on mice with dorsal skin infections validated further Fe-1GMP hydrogel's antibacterial efficacy, underscoring its potential for topical applications. Such supramolecular metallogels hosting the Fenton chemistry with precise control over the spatiotemporal generation of ROS and adjustable mechanical properties make them suitable for exploitation in bacterial control in wound management in biomedical fields, and work is currently underway in our laboratory toward these applications.
Data availability
The data supporting this article have been included as part of the ESI.†
Conflicts of interest
There are no conflicts to declare.
Acknowledgements
The authors are thankful to the Institute of Nano Science and Technology (INST), Mohali, for providing infrastructure and characterization facilities. The authors are grateful to the animal facilities of IACS, Kolkata. The authors gratefully acknowledge the generous help of Prof. Avinash Bajaj and Varsha Saini from the Regional Centre for Biotechnology (RCB), Faridabad, for providing access to the BSL-II facility to conduct in vitro antimicrobial assays of drug-resistant bacteria. SB acknowledges DST for the award of the J C Bose Fellowship. A. Pal expresses gratitude for financial support from SERB grants (CRG/2020/004251) and the Department of Biotechnology (BIRAC-PACE, BT/AIR01159/PACE-21/20). VC and Umesh thank INST for the fellowship.
References
- H. C. Neu, Science, 1992, 257, 1064–1073 CrossRef CAS PubMed.
- H. Liu, T. Xu, Z. Xue, M. Huang, T. Wang, M. Zhang, R. Yang and Y. Guo, ACS Infect. Dis., 2024, 2, 350–370 CrossRef PubMed.
- X. Li, S. M. Robinson, A. Gupta, K. Saha, Z. Jiang, D. F. Moyano, A. Sahar, M. A. Riley and V. M. Rotello, ACS Nano, 2014, 8, 10682–10686 CrossRef CAS PubMed.
- K. M. G. O'Connell, J. T. Hodgkinson, H. F. Sore, M. Welch, G. P. C. Salmond and D. R. Spring, Angew. Chem., Int. Ed., 2013, 52, 10706–10733 CrossRef PubMed.
- Q. Zeng, X. Qi, G. Shi, M. Zhang and H. Haick, ACS Nano, 2022, 16, 1708–1733 CrossRef CAS PubMed.
- Y. Yang, B. Chu, J. Cheng, J. Tang, B. Song, H. Wang and Y. Yao, Nat. Commun., 2022, 13, 1255 CrossRef CAS PubMed.
- M. K. Pradhan, D. Gupta, K. R. Namdev, C. Miglani, A. Pal and A. Srivastava, Nanoscale, 2022, 14, 15079–15090 RSC.
- L. Schnaider, S. Brahmachari, N. W. Schmidt, B. Mensa, S. Shaham-Niv, D. Bychenko, L. Adler-Abramovich, L. J. W. Shimon, S. Kolusheva, W. F. DeGrado and E. Gazit, Nat. Commun., 2017, 8, 1365 CrossRef PubMed.
- D. Nath, J. Ralhan, J. P. Joseph, C. Miglani and A. Pal, Biomacromolecules, 2024, 25, 853–863 CrossRef CAS PubMed.
- E. O. Ogunsona, R. Muthuraj, E. Ojogbo, O. Valerio and T. H. Mekonnen, Appl. Mater. Today, 2020, 18, 100473 CrossRef.
- V. B. Kumar, M. Lahav and E. Gazit, J. Mater. Chem. B, 2024, 12, 2855–2868 RSC.
- M. Makvandi, C. Y. Wang, E. N. Zare, A. Borzacchiello, L. N. Niu and F. R. Tay, Adv. Funct. Mater., 2020, 30, 1910021 CrossRef.
- I. J. Budiarso, N. D. W. Rini, A. Tsalsabila, M. D. Birowosuto and A. Wibowo, ACS Biomater. Sci. Eng., 2023, 9, 3084–3115 CrossRef CAS PubMed.
- P. Prasad and S. Gupta, ACS Appl. Bio Mater., 2020, 3, 8271–8285 CrossRef CAS PubMed.
- S. Li, S. Dong, W. Xu, S. Tu, L. Yan, C. Zhao, J. Ding and X. Chen, Adv. Sci., 2018, 5, 1700527 CrossRef PubMed.
- B. O. Okesola and D. K. Smith, Chem. Soc. Rev., 2016, 45, 4226–4251 RSC.
- M. C. Sardaru, S. Morariu, O. E. Carp, E. L. Ursu, A. Rotaru and M. Barboiu, Chem. Commun., 2023, 59, 3134–3137 RSC.
- A. Maleki, J. He, S. Bochani, V. Nosrati, M. A. Shahbazi and B. Guo, ACS Nano, 2021, 15, 18895–18930 CrossRef CAS PubMed.
- S. Ghosh, S. Mukherjee, D. Patra and J. Haldar, Biomacromolecules, 2022, 23, 592–608 CrossRef CAS PubMed.
- K. Ghosal, D. Chakraborty, V. Roychowdhury, S. Ghosh and S. Dutta, ACS Omega, 2022, 7, 43364–43380 CrossRef CAS PubMed.
- Z. Ahmadian, A. Correia, M. Hasany, P. Figueiredo, F. Dobakhti, M. R. Eskandari, S. H. Hosseini, R. Abiri, S. Khorshid, J. Hirvonen, H. A. Santos and M.-A. Shahbazi, Adv. Healthcare Mater., 2021, 10, 2001122 CrossRef CAS PubMed.
- B. Bhattacharjee, S. Ghosh and J. Haldar, ACS Appl. Bio Mater., 2023, 6, 4867–4876 CrossRef CAS PubMed.
- S. Kumar, S. Pal, J. Thakur, P. Rani, K. Rana, A. Kar, R. Kar, D. Mehta, S. K. Jha, M. K. Pradhan, D. Jain, K. Rajput, S. Mishra, M. Ganguli, A. Srivastava, U. Dasgupta, V. S. Patil and A. Bajaj, ACS Appl. Mater. Interfaces, 2021, 13, 44041–44045 CrossRef CAS PubMed.
- S. Paul, S. Verma and Y.-C. Chen, ACS Infect. Dis., 2024, 10, 1034–1055 CrossRef CAS PubMed.
- M. Halder, M. Narula and Y. Singh, Bioconjugate Chem., 2023, 34, 645–663 CAS.
- I. Mukherjee, A. Ghosh, P. Bhadury and P. De, ACS Omega, 2017, 2, 1633–1644 CrossRef CAS PubMed.
- V. Castelletto, R. H. Barnes, K. A. Karatzas, C. J. Edwards-Gayle, F. Greco, I. W. Hamley, R. Rambo, J. Seitsonen and J. Ruokolainen, Biomacromolecules, 2018, 19, 2782–2794 CrossRef CAS PubMed.
- G. Pandit, N. Chowdhury, Sk. A. Mohid, A. P. Bidkar, A. Bhunia and S. Chatterjee, ChemMedChem, 2021, 16, 355–367 CrossRef CAS PubMed.
- E. Pazos, E. Sleep, C. M. Rubert Pérez, S. S. Lee, F. Tantakitti and S. I. Stupp, J. Am. Chem. Soc., 2016, 138, 5507–5510 CrossRef CAS PubMed.
- J. Ralhan, V. Kumar, H. Bhatt, D. Nath, N. A. Mavlankar, H. N. Ghosh and A. Pal, Langmuir, 2024, 5, 2754–2763 Search PubMed.
- A. M. Itoo, M. Paul, S. G. Padaga, B. Ghosh and S. Biswas, ACS Omega, 2022, 7, 45882–458909 CrossRef CAS PubMed.
- S. Liao, M. Cai, R. Zhu, T. Fu, Y. Du, J. Kong, Y. Zhang, C. Qu, X. Dong, J. Ni and X. Yin, Mol. Pharmaceutics, 2023, 20, 875–885 CrossRef CAS PubMed.
- S. D. Bhagat and A. Srivastava, Biomater. Sci., 2020, 8, 4750–4756 RSC.
- X. Wang and M. Zheng, ACS Appl. Nano Mater., 2023, 6, 13689–13697 CrossRef.
- B. Yang, Y. Chen and J. Shi, Chem. Rev., 2019, 119, 4881–4985 CrossRef CAS PubMed.
- H. Ranji-Burachaloo, P. A. Gurr, D. E. Dunstan and G. G. Qiao, ACS Nano, 2018, 12, 11819–11837 CrossRef CAS PubMed.
- Z. Tang, P. Zhao, H. Wang, Y. Liu and W. Bu, Chem. Rev., 2021, 121, 1981–2019 CrossRef CAS PubMed.
- S. Ghosh, A. Maity and N. R. Jana, ACS Appl. Nano Mater., 2023, 6, 21595–21602 Search PubMed.
- I. Banerjee, R. C. Pangule and R. S. Kane, Adv. Mater., 2011, 23, 690–718 CrossRef CAS PubMed.
- M. Godoy-Gallardo, M. Merino-Gómez, L. C. Matiz, M. A. Mateos-Timoneda, F. J. Gil and R. A. Perez, ACS Biomater. Sci. Eng., 2022, 9, 40–61 CrossRef PubMed.
- V. D. Nguyen, A. Pal, F. Snijkers, M. Colob-Delsuc, G. Leonetti, S. Otto and J. van der Gucht, Soft Matter, 2016, 12, 432–440 RSC.
- A. Singh, J. P. Joseph, D. Gupta, C. Miglani, N. A. Mavlankar and A. Pal, Nanoscale, 2021, 13, 13401–13409 RSC.
- E. Mattia, A. Pal, G. Leonetti and S. Otto, Synlett, 2017, 28, 103–107 CAS.
- C. Zheng, X. Wu, M. Liu, Y. Lan, Q. Liu, E. Cai, Z. Liao and J. Shen, Smart Med., 2024, e20230047 CrossRef CAS.
- G. M. Peters, L. P. Skala, T. N. Plank, H. Oh, G. N. M. Reddy, A. Marsh, S. P. Brown, S. R. Raghavan and J. T. Davis, J. Am. Chem. Soc., 2015, 137, 5819–5827 CrossRef CAS PubMed.
- S. Sarkar, S. Bera, P. Moitra and S. Bhattacharya, Mater. Today Chem., 2023, 30, 101554 CrossRef.
- A. Bhunia, V. Vasylyeva and C. Janiak, Chem. Commun., 2013, 49, 3961–3963 RSC.
- D. Bhattacharyya, G. M. Arachchilage and S. Basu, Front. Chem., 2016, 4, 38 Search PubMed.
- H. X. Jiang, Y. Cui, T. Zhao, H. W. Fu, D. Koirala, J. A. Punnoose, D. M. Kong and H. Mao, Sci. Rep., 2015, 5, 9255 CrossRef CAS PubMed.
- A. Ghosh, B. Parasar, T. Bhattacharyya and J. Dash, Chem. Commun., 2016, 52, 11159–11162 RSC.
- J. P. Joseph, N. Gupta, C. Miglani, D. Nath, A. Singh, D. Gupta and A. Pal, Chem. Mater., 2022, 34, 4364–4374 CrossRef CAS.
- J. Thomas, N. Gupta, J. P. Joseph, V. Chopra, A. Pal and D. Ghosh, ACS Biomater. Sci. Eng., 2021, 7, 5798–5809 CrossRef CAS PubMed.
- K. Sharma, J. P. Joseph, A. Sahu, N. Yadav, M. Tyagi, A. Singh, A. Pal and K. P. R. Kartha, RSC. Adv., 2019, 9, 19819–19827 RSC.
- G. Zhu, Q. Wang, S. Lu and Y. Niu, Med. Princ. Pract., 2017, 26, 301–308 CrossRef PubMed.
- F. A. Pinho-Ribeiro, L. Deng, D. V. Neel, O. Erdogan, H. Basu, D. Yang, S. Choi, A. J. Walker, S. Carneiro-Nascimento, K. He and G. Wu, Nature, 2023, 615, 472–481 CrossRef CAS PubMed.
- G. Sandri, M. C. Bonferoni, F. D'Autilia, S. Rossi, F. Ferrari, P. Grisoli, M. Sorrenti, L. Catenacci, C. Del Fante, C. Perotti and C. Caramella, Eur. J. Pharm. Biopharm., 2013, 84, 84–90 CrossRef CAS PubMed.
- T. Li, J. Li, Q. Pang, L. Ma, W. Tong and C. Gao, ACS Appl. Mater. Interfaces, 2019, 11, 6789–6795 CrossRef CAS PubMed.
Footnotes |
† Electronic supplementary information (ESI) available: Synthesis and additional figures. See DOI: https://doi.org/10.1039/d4nr01011f |
‡ These authors contributed equally to this work. |
|
This journal is © The Royal Society of Chemistry 2024 |
Click here to see how this site uses Cookies. View our privacy policy here.