DOI:
10.1039/D4NR01769B
(Paper)
Nanoscale, 2024,
16, 14310-14318
Embedding AIE-type Ag28Au1 nanoclusters within ZIF-8 for improved photodynamic wound healing through bacterial eradication†
Received
23rd April 2024
, Accepted 20th June 2024
First published on 3rd July 2024
Abstract
Designing antibacterial agents with rapid bacterial eradication performance is paramount for the treatment of bacteria-infected wounds. Metal nanoclusters (NCs) with aggregation-induced emission (AIE) have been considered as novel photodynamic antibacterial agents without drug resistance, but they suffer from poor photostability and low charge carrier separation efficiency. Herein, we report the design of a photodynamic antibacterial agent by encapsulating AIE-type AgAu NCs (Ag28Au1 NCs) into a zeolitic Zn(2-methylimidazole)2 framework (ZIF-8). The encapsulation of AIE-type Ag28Au1 NCs into porous ZIF-8 could not only enhance the photostability of Ag28Au1 NCs by inhibiting their aggregation but also promote the separation of photoinduced charge carriers, resulting in the rapid generation of destructive reactive oxygen species (ROS) for bacterial killing under visible-light irradiation. Consequently, the as-designed photodynamic Ag28Au1 NCs@ZIF-8 antibacterial agent could rapidly eliminate 97.7% of Escherichia coli (E. coli) and 91.6% of Staphylococcus aureus (S. aureus) within 5 min in vitro under visible light irradiation. Furthermore, in vivo experimental results have highlighted the synergistic effect created by AIE-type Ag28Au1 NCs and ZIF-8, enabling Ag28Au1 NCs@ZIF-8 to effectively eradicate bacteria in infected areas, reduce inflammation, and promote the generation of blood vessels, epithelial tissue, and collagen. This synergistic effect promoted the healing of S. aureus-infected wound, with nearly 100% of wound recovery within 11 days. This work may be interesting because it sheds light on the design of metal NC-based photodynamic nanomedicine for bacteria-infected disease treatment.
1. Introduction
Wound infections, afflicting over several million people each year, are a growing public health threat.1–5 Open wounds provide a rapid breeding ground for pathogenic bacteria, such as Escherichia coli (E. coli) and Staphylococcus aureus (S. aureus), thus impeding wound healing and increasing the risk of severe infection-related syndromes such as localized septic infection, sepsis, acute renal failure, and even death.6–8 Therefore, rapid bacterial eradication in infected areas is crucial for effective treatment. While antibiotic therapy is a common clinical strategy,9,10 the low antibacterial efficiency of antibiotics could cause the formation of biofilms, leading to a high failure rate of treatment.11 Furthermore, antibiotic abuse can accelerate bacterial resistance, resulting in the emergence of superbacteria.12 Hence, there is an urgent need to develop a fast antimicrobial strategy for wound infection treatment.
Recently, antimicrobial photodynamic therapy has emerged as a promising strategy for treating bacteria-infected wounds.13–15 This therapy harnesses reactive oxygen species (ROS) generated from photocatalysts under light irradiation to rapidly kill bacteria without inducing antimicrobial resistance.13,16 Consequently, extensive research has been conducted to develop efficient photocatalysts for wound infection treatment.17 Metal nanoclusters (NCs) with aggregation-induced emission (AIE), such as gold NCs (Au NCs),18–22 silver NCs (Ag NCs),23–25 or alloy NCs (AuAg NCs),26–28 are considered as good candidates due to their photochemical properties (e.g., a large Stokes shift, a high content of metal(I) components, strong photoluminescence (PL),29–31 and a long-lived PL lifetime32–34). Metal NC-based antibacterial agents traditionally function by sacrificing themselves, either through metal ion release or cell adhesion/internalization, in order to kill bacteria. This self-consumed antibacterial action, however, renders them ineffective for long-lasting bacterial killing. In contrast, AIE-type metal NCs have the unique ability to generate ROS under light illumination, which effectively deactivates bacteria. This characteristic offers an innovative approach to achieving reliable and durable photodynamic bacterial inactivation, serving as an alternative to traditional methods based on advanced oxidation techniques.35 However, the ultrasmall size of AIE-type metal NCs usually leads to structural decomposition and fast recombination of the photogenerated carriers, hindering their utility in photodynamic therapy.36 To address this limitation, encapsulating metal NCs into a rigid porous material to strengthen stability and promote separation of photogenerated carriers might be a feasible strategy.37 In this regard, metal–organic frameworks (MOFs) are good porous candidates. MOFs, with their highly ordered structures and ultrahigh porosity, provide a platform to immobilize metal NCs.21 Additionally, most MOFs are semiconductors with a large energy-band gap, enabling them to serve as electron acceptors to promote the separation of photogenerated charge carriers for other photocatalysts.38 MOFs can also promote wound healing by facilitating cell proliferation or differentiation.39,40 Therefore, we hypothesize that if we could encapsulate metal NCs into the porous structure of MOFs, we may overcome the challenges of poor photostability and fast recombination of photogenerated carriers in AIE-type metal NCs, thereby achieving rapid and sustained sterilization for wound healing.
Herein, we report the design of a photodynamic antibacterial agent for rapid photodynamic bacterial eradication facilitating wound healing by encapsulating AIE-type bimetallic AgAu NCs (namely, Ag28Au1 NCs, protected by dihydrolipoic acid (DHLA)) into a zeolitic Zn(2-methylimidazole)2 framework (ZIF-8) (Scheme 1). The key design feature is the employment of ZIF-8 as a porous matrix, which serves to enhance the photostability of the Ag28Au1 NCs by preventing their migration and subsequent aggregation. Furthermore, ZIF-8 acts as an electron acceptor, facilitating the efficient separation of photogenerated charge carriers in the Ag28Au1 NCs, thereby ensuring the rapid generation of ROS essential for fast bacterial eradication. This novel design of the photodynamic antibacterial agent (namely, Ag28Au1 NCs@ZIF-8) demonstrated a notable capability for rapid bacterial inactivation under visible light irradiation in vitro, along with a favorable wound healing effect in vivo. The outcomes of this study highlight the promising photodynamic therapeutic potential of the proposed agent in addressing wound infections, potentially opening up new avenues for future research endeavors on developing other NC-based photodynamic antibacterial agents for the treatment of bacteria-infected wounds.
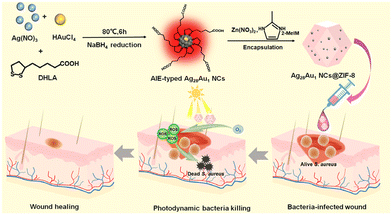 |
| Scheme 1 Schematic diagram of the preparation of Ag28Au1 NCs@ZIF-8 antibacterial agents for enhanced wound healing via rapid photodynamic bacterial eradication. | |
2. Experimental section
2.1. Chemicals and materials
Chloroauric acid (HAuCl4, ≥99.9%), silver nitrate (AgNO3, ≥99.8%), sodium hydroxide (NaOH, ≥96%), ethanol (C2H5OH, ≥99.0%), zinc nitrate (Zn(NO3)2·6H2O, ≥99.0%), 2-methylimidazole (≥99.8%), acetonitrile (CH3CN, ≥99%), glutaraldehyde solution (C5H8O2, 25%–28%), and 2,7-dichlorodihydrofluorescein diacetate (DCFH-DA) dye were purchased from Sinopharm Chemical Reagent Co., Ltd. Alphalipoic acid (DHLA, ≥99%) and sodium tetrahydroborate (NaBH4, ≥96%) were purchased from Shanghai Shanpu Chemical Co., Ltd (China). Formalin solution was purchased from Merck. Ultrapure water was used throughout the experiments. Phosphate buffer solution (PBS), Luria–Bertani (LB) nutrient agar, and LB broth were purchased from Qingdao Hope Bio-Technology Co., Ltd. Carbomer 940 was purchased from Shanghai McLean Biochemical Co., Ltd. A complete Dulbecco's modified Eagle's medium (DMEM) was purchased from Beijing Solarbio Science & Technology Co., Ltd. Standard strains of Staphyloccocus aureus ATCC 6538 (S. aureus) and Escherichia coli ATCC 25922 (E. coli) were purchased from China General Microbiological Culture Collection Center.
The animals were purchased from China Weitong Lihua Animal Co., Ltd., and female ICR mice aged 6 weeks were selected. Animals were maintained under specific pathogen-free conditions and fed ad libitum. Animal experiments were conducted according to the international ethics guidelines and the National Institutes of Health Guide concerning the Care and Use of Laboratory Animals.
2.2. Instrumentation
The size and morphology of samples were observed using a transmission electron microscope (TEM, JEM-2100 Plus Electron Microscope) and a scanning electron microscope (SEM, JSM-6700F). The elemental mapping results of Ag28Au1 NCs@ZIF-8 were obtained using an energy dispersive spectrometer (EDS, OXFORD MAX-80, INCA SYSTEM). The optical properties of Ag28Au1 nanoclusters (NCs) and the turbidity of bacteria were measured by UV-vis absorption spectroscopy using a Shimadzu UV-1800 spectrometer. The molecular weight of Ag28Au1 NCs was analyzed by electrospray ionization-mass spectrometry (ESI-MS, Bruker Impact II). Fourier transform infrared (FTIR) spectra of Ag28Au1 NCs@ZIF-8 and ZIF-8 were recorded from 3800 to 450 cm−1 using a Horiba-Jobin Yvon FluoroMax-4 fluorescence spectrophotometer. The content of the Zn2+ in the hydrogel and that released from the hydrogel was determined by inductively coupled plasma mass spectrometry (ICP-MS, Agilent 720-ES). X-ray photoelectron spectroscopy (XPS) data were obtained using an X-ray photoelectron spectrometer (ESCALAB MK II Axis Ultra Dld system). X-ray diffraction (XRD) patterns were obtained using an X-ray diffractometer (D-Max 2500/PC). The time-resolved photoluminescence (PL) spectrum was recorded using a time-resolved PL spectrometer (FLS-1000).
2.3. Preparation of AIE-type Ag28Au1 NCs
AgNO3 (20 mM) solution and HAuCl4 (20 mM) solution were prepared using water, and DHLA (50 mM) solution was prepared using 3 mL NaOH (71.4 mM) solution. In a typical synthesis of highly luminescent Ag28Au1 NCs, 2.897 mL of AgNO3 (20 mM) and 0.103 mL of HAuCl4 (20 mM) were first added to DHLA (50 mM, 6 mL) solution and stirred for 10 min to synthesize DHLA–metal(I) complexes. Subsequently, a newly prepared NaBH4 (100 mM, 1.2 mL) aqueous solution was added to the reaction system, stirred at room temperature for 6 h, and then heated at 80 °C for 2 h to synthesize a highly luminescent Ag28Au1 NC solution. The obtained Ag28Au1 NCs were purified by centrifuging the mixture (Ag28Au1 NCs
:
acetonitrile = 1
:
3 volume ratio) at 8000 rpm for 5 min. The obtained solids of Au NCs were redissolved in 10 mL of H2O for further use.
2.4. Preparation of DHLA-protected Ag29 NCs
3 mL of AgNO3 (20 mM) was first added to DHLA (50 mM, 6 mL) solution and stirred for 10 min to synthesize DHLA–metal(I) complexes. Subsequently, the newly prepared NaBH4 (100 mM, 1.2 mL) aqueous solution was added to the reaction system, stirred at room temperature for 6 h, and then heated at 80 °C for 2 h to synthesize a DHLA-protected Ag29 NC solution. The obtained NCs were purified by centrifuging the mixture (Ag28Au1 NCs
:
acetonitrile = 1
:
3 volume ratio) at 8000 rpm for 5 min. The obtained solids of Ag28Au1 NCs were redissolved in 10 mL of H2O for further use.
2.5. Preparation of Ag28Au1 NCs@ZIF-8
5.4 mL of Ag28Au1 NC solution was introduced into a beaker containing 34.60 mL of water. Subsequently, 162.8 μL of Zn(NO3)2 (0.49 M) solution was added to the beaker under stirring at room temperature for 1 h, followed by adding 1.62 mL of 2-methylimidazole (3.456 M) under stirring for another 10 min. The reaction was allowed to stay at room temperature for 6 h without stirring. Finally, the red-brown precipitate was collected by centrifugation, washed five times with water, and dried under vacuum at room temperature for 24 h to obtain Ag28Au1 NCs@ZIF-8.
2.6. Preparation of ZIF-8
1.628 mL of Zn(NO3)2 (0.49 M) solution was added to a beaker containing 16.2 mL of 2-methylimidazole (3.456 M) under stirring for 10 min. The reaction was allowed to stay at room temperature for 6 h without stirring. Finally, the white precipitate was collected by centrifugation, washed five times with water, and dried under vacuum at room temperature for 24 h to obtain ZIF-8.
2.7. Photodynamic antibacterial test
Gram-negative E. coli and Gram-positive S. aureus were used as bacterial models to evaluate the photodynamic bactericidal activity of the photodynamic antibacterial agents under visible light irradiation. All the glassware and medium solutions were sterilized in an autoclave at 121 °C for 30 min before the antibacterial test. All tests were performed under sterile conditions. Bacterial cells were cultured in LB medium on a shaking table at 37 °C for 18 h, centrifuged at 8000 rpm for 5 min to remove metabolites, and finally diluted with PBS solution (0.01 M, pH 7.4) to obtain a bacterial suspension with a cell count of 107 CFU mL−1. In a typical antibacterial experiment, ZIF-8 (4.65 mg), Ag28Au1 NCs (0.35 mg), their blended sample (4.65 mg of Ag28Au1 NCs and 0.35 mg of Ag28Au1 NCs, Ag28Au1 NCs + ZIF-8), and Ag28Au1 NCs@ZIF-8 (5 mg) were separately mixed with 5 mL of bacterial suspension, respectively. Irradiation was performed using a commercial 300 W xenon lamp with an optical cut-off filter (λ = 400–1100 nm, 100 mW cm−2, CEL-HXF300, Beijing China Education AuLight Technology (CEAuLight) Co., Ltd). Then, at the given irradiation interval (0, 5, 10, 15, 20 min), 100 μL of bacterial suspension was coated on a fresh agar plate and cultured at 37 °C for 24 h. Finally, the colonies growing on the plate were counted to determine the number of viable bacteria after culture. In addition, the corresponding control tests were performed under the same conditions (for example, antimicrobial tests with or without the use of antimicrobials under visible light irradiation or dark conditions).
2.8. ROS assay
The intracellular ROS levels were analyzed by using fluorescent 2′,7′-dichlorofluorescein (DCF) dye as the indicator, which was produced through the oxidation of DCFH-DA by ROS. In brief, a mixed solution of 5 mL of E. coli and 5 mg of the Ag28Au1 NCs@ZIF-8 antibacterial agent was firstly irradiated with visible light under O2 bubbling for 20 min. Subsequently, 10 μL of DCFH-DA (200 μM) was introduced into the mixed solution and incubated for 20 min at 37 °C to ensure the oxidation of DCFH-DA by ROS. After that, the bacterial suspension was centrifuged at 5000 rpm for 5 min, washed three times with PBS, and re-suspended in PBS to the original volume (1 mL). The concentration of the resulting DCF was determined by recording its PL intensity on a microplate reader at an excitation/emission wavelength of 488/525 nm. In addition, bacterial suspensions treated with Ag28Au1 NCs and ZIF-8 were subjected to the same conditions.
2.9. Cytotoxicity assessment
The cytotoxicity of various antibacterial agents was evaluated using a cell proliferation kit (MTT) with the HeLa cell as a cell model. Firstly, the cells were seeded in a 96-well plate and cultured under standard culture conditions (37 °C, 5% CO2) for 24 h. The antibacterial agent (dispersed in carbomer) was put into wells for irradiation or darkness treatment. After post-seeding for 24 h, 20 μL of the MTT solution was added into the wells and allowed to culture in an incubator for 4 h. Afterwards, the MTT solution was discarded, and DMSO was added to the wells. After stationary culture for 30 min, the optical density of the supernatants in the 96-well plate was measured at 570 nm using a microplate reader.
2.10. Animal experiments
All animal experiments were approved by the Biomedical Ethics Committee of Qingdao Zhong Hao Biological Engineering Co., Ltd (Qingdao, China). The mice were randomly assigned to 4 groups, with 3 mice in each group, according to the samples: control (carbomer), ZIF-8 (dispersed in carbomer), Ag28Au1 NCs (dispersed in carbomer), and Ag28Au1 NCs@ZIF-8 (dispersed in carbomer). The mice were anesthetized with isoflurane (2%) and had their back hair removed with a depilatory cream. A skin biopsy perforator (8 mm in diameter) was used to create a full-thickness wound on the pasteurized dorsal skin. The wound sites were inoculated with S. aureus suspensions (100 μL, 108 CFU mL−1), covered with 3 M films, and left for 1 day to develop severe infection. Subsequently, the infected wounds were treated with various photodynamic antibacterial agents (200 μL, 1 mg mL−1) and carbomer (200 μL, control). Afterwards, the infected wounds were irradiated with a commercial 300 W xenon lamp with an optical cut-off filter (λ > 400 nm, 100 mW cm−2) for 5 min each day. After being treated for eleven days, all mice were sacrificed and the surrounding wound tissues were collected and fixed in paraformaldehyde (4%) for further H&E staining and Masson trichrome staining. Blood was collected and used to detect the levels of IL-6, IL-1β, and TNF-α.
3. Results and discussion
3.1. Synthesis and characterization of Ag28Au1 NCs@ZIF-8
In this study, highly luminescent AuAg alloy NCs with AIE were chosen as the NC model. The red-brown colored NC solution (the inset in Fig. 1a) shows a molecule-like optical absorption nature with two distinct absorption peaks at 476 and 405 in the visible light region (Fig. 1a). The peak at 476 nm arises from the intraband (sp ← sp) transition, while the peak at 405 nm results from the combination of intraband (sp ← sp) and interband (d ← sp) transitions. The well-defined absorption peaks manifest a very high quality of raw NCs, which is evidenced by the electrospray ionization mass spectrometry (ESI-MS) spectrum (Fig. 1b). This spectrum reveals three distinct sets of main peaks at m/z 1934, 1439, and 1173, corresponding to [Ag28Au1(DHLA)12–2H]3−, [Ag28Au1(DHLA)12–3H]4−, and [Ag28Au1(DHLA)12–4H]5−, respectively. The experimental mass distribution of NCs closely aligns with the simulated distribution (Fig. S1†), affirming the successful preparation of Ag28Au1 NCs. Additionally, the transmission electron microscope (TEM) image shows that Ag28Au1 NCs are below 2 nm in size with an average size of ∼1.7 nm (Fig. S2†), further corroborating the successful synthesis of ultrasmall NCs. Notably, the as-synthesized Ag28Au1 NCs show the typical AIE nature with a large Stokes shift exceeding 200 nm, as evidenced by their excitation and emission spectra (emitting red fluorescence (inset) with an emission peak at 645 nm (blue curve) upon excitation at 414 nm). Moreover, reducing the solution polarity by introducing an organic solvent (methanol) reinforces the aggregation state of the AIE-type Ag28Au1 NCs and constrains their nonradiative relaxation, leading to the enhancement of PL intensity (Fig. S3†). It was also found that although the Au-doped Ag28Au1 NCs have similar optical absorption to Ag29 NCs (Fig. S4†), they exhibit almost 4.7 times luminescence enhancement (Fig. 1c). The enhanced FL intensity of Ag29 NCs after a single Au atom doping can primarily be attributed to the enhanced structural stability and intramolecular interaction after Au atom doping, thereby inhibiting non-radiative transitions and ultimately leading to a stronger fluorescence response. This enhanced luminescence makes Ag28Au1 NCs the ideal antibacterial materials in terms of their efficient generation of photoinduced charge carriers, and this is why we chose gold–silver alloy NCs. Concurrently, the time-resolved PL profile of Ag28Au1 NCs contains two components (Fig. 1d): a short-lived PL lifetime of 0.61 μs (τ1, derived from the interband transition (d ← sp) of the metal cores) and a long-lived PL lifetime of 2.49 μs (τ2, caused by the ligand-to-metal or ligand-to-metal–metal charge transfer). The dominant contribution of τ2 (94.64%) indicates that Ag28Au1 NCs possess long-lived photoluminescence (PL) lifetimes up to the μs-level (2.47 μs), surpassing the lifetimes of most AIE-free metal NCs (typically in the ps or ns range). Notably, the large Stokes shift, strong PL and long-lived PL lifetime are typical characteristics of AIE-type metal NCs, which are highly desirable for achieving highly efficient photodynamic antibacterial activity.
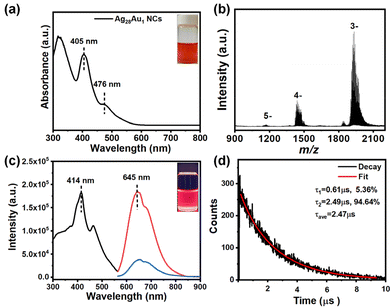 |
| Fig. 1 (a) UV–visible absorption (inset: optical photo of Ag28Au1 NC solution under visible light illumination) and (b) ESI-MS spectrum of Ag28Au1 NCs. (c) Photoexcitation (black curve) and photoemission spectra (red curve) of Ag28Au1 NCs, and photoexcitation (green curve) and photoemission spectrum of Ag29 NCs (blue curve). (d) PL decay profile and the corresponding fit curve of the Ag28Au1 NCs. The inset shows the calculated PL lifetimes according to the fit curve (τave: average PL lifetime). | |
Ag28Au1 NCs@ZIF-8 was synthesized in the presence of AIE-type Ag28Au1 NC stock solution and ZIF-8 precursor. As shown, the obtained Ag28Au1 NCs@ZIF-8 display a regular rhombic dodecahedral structure with a diameter of around 600 nm (Fig. 2a), with ultrasmall black spot particles, each below 2 nm in size, uniformly spread throughout the ZIF-8 (Fig. 2b), indicating the successful encapsulation of AIE-type Ag28Au1 NCs into porous ZIF-8. Furthermore, the energy dispersive X-ray (EDX) elemental mapping (Fig. 2c) images provide visual evidence of good distribution of Ag28Au1 NCs in ZIF-8. Notably, the encapsulation of Ag28Au1 NCs did not change the size and crystal structure of ZIF-8, as evidenced by their similar sizes (Fig. S5†) and X-ray diffraction (XRD) patterns (Fig. 2d). In addition, Fourier-transform infrared (FTIR) analysis was performed to further determine the interaction between AIE-type Ag28Au1 NCs and ZIF-8. The FTIR spectrum of Ag28Au1 NCs@ZIF-8 shows similarity to that of ZIF-8, except for the emergence of a peak at 650 cm−1 corresponding to the Zn–O stretching vibration, confirming the interaction between COO– in NCs and Zn2+ in ZIF-8 (Fig. S6†). Concurrently, the X-ray photoelectron spectra (XPS) reveal that the binding energy of Ag3d and S2p in Ag28Au1 NCs@ZIF-8 shows an increase of about 0.31 eV and 0.44 eV, respectively, compared to that of pristine Ag28Au1 NCs (Fig. 2e and Fig. S7†), suggesting a reduction in the electron density of the Ag and S elements in Ag28Au1 NCs@ZIF-8. Conversely, the binding energy of Zn2p shows a negative shift of approximately 0.36 eV compared to that of pristine ZIF-8 (Fig. 2f), indicating an increase in the electron density of the Zn element in Ag28Au1 NCs@ZIF-8. Based on the above analysis, we may conclude that the AIE-type Ag28Au1 NCs were successfully confined into ZIF-8, and their interaction promotes charge transfer from the Ag and S elements in AIE-type Ag28Au1 NCs to the Zn element in ZIF-8, which contributes to improving the stability and promoting the separation of photoinduced charge carriers in AIE-type Ag28Au1 NCs.
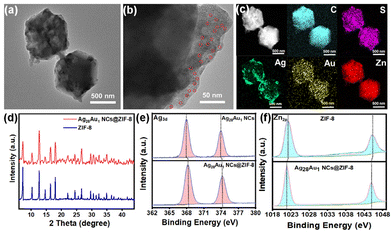 |
| Fig. 2 TEM image (a) and the enlarged TEM image (b) of Ag28Au1 NCs@ZIF-8. (c) The EDX elemental mapping of C, S, Ag, Au and Zn of Ag28Au1 NCs@ZIF-8. (d) XRD patterns of Ag28Au1 NCs@ZIF-8 and ZIF-8. (e) High-resolution XPS of Ag3d in Ag28Au1 NCs@ZIF-8 and Ag28Au1 NCs. (f) High-resolution XPS of Zn2p in Ag28Au1 NCs@ZIF-8 and ZIF-8. | |
3.2. Photodynamic antibacterial tests in vitro
After the successful preparation of Ag28Au1 NCs@ZIF-8, its photodynamic antibacterial activity was investigated by using representative Gram-negative (E. coli) and Gram-positive (S. aureus) bacteria. As shown in Fig. 3, the as-deigned Ag28Au1 NCs@ZIF-8 effectively killed over 97% of E. coli and 91% of S. aureus within 5 min (Fig. 3a and b) and over 99.8% of E. coli (2.7 orders of magnitude reduction in colony-forming unit (CFU; Fig. 3c)) and 99.2% of S. aureus (2.1 orders of magnitude reduction in CFU; Fig. 3d) within 20 min under visible-light illumination (λ ≥ 400 nm) and O2 bubbling. Additionally, Ag28Au1 NCs@ZIF-8 consistently exhibited superior antibacterial efficacy against Gram-negative bacteria compared to Gram-positive bacteria, owing to the heightened susceptibility of Gram-negative bacteria with their thinner cell walls rendering them more vulnerable to destruction by ROS. Conversely, pristine Ag28Au1 NCs, ZIF-8, and their blended sample (Ag28Au1 NCs + ZIF-8) did not exhibit similar photodynamic antibacterial activity under the same conditions (Fig. S8 and S9†). The enhanced antibacterial activity of Ag28Au1 NCs@ZIF-8 can be attributed to the encapsulation of Ag28Au1 NCs into ZIF-8, which improves the stability of Ag28Au1 NCs and facilitates the separation of photoinduced charge carriers, thereby enhancing the generation of destructive ROS for bacterial eradication. The mechanism will be discussed later. Moreover, the control experiment under light illumination and O2 bubbling (light + O2) indicates that light and O2 have no significant effect on bacterial killing. Notably, the bacterial eradication efficacy of Ag28Au1 NCs@ZIF-8 was demonstrated by its ability to eliminate 94.1% of E. coli and 92.2% of S. aureus within 20 min under light illumination and exposure to air (Ag28Au1 NCs@ZIF-8 + light). Conversely, no significant bacterial killing was observed under dark conditions (Ag28Au1 NCs@ZIF-8), indicating the crucial roles of light illumination and O2 (air) in the bacterial eradication process (Fig. S8 and S9†). These results suggest that the encapsulation of Ag28Au1 NCs into ZIF-8 achieves rapid bacterial eradication, making Ag28Au1 NCs@ZIF-8 a promising photodynamic antibacterial agent for the treatment of bacteria-infected wounds.
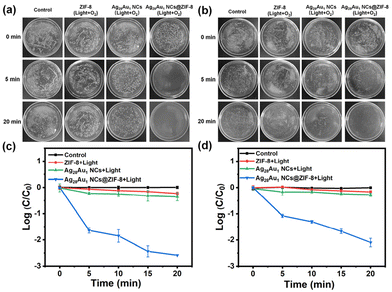 |
| Fig. 3 Bacterial colony growth of (a) E. coli and (b) S. aureus in the presence of various samples under O2 bubbling and visible-light illumination for 20 min, and treatment without any agent under dark conditions (control) for 20 min. Bactericidal activities of different samples against (c) E. coli and (d) S. aureus. | |
3.3. The antibacterial mechanism of Ag28Au1 NCs@ZIF-8
The investigation into the enhanced photodynamic antibacterial mechanism involved measuring the light absorption capability of pristine Ag28Au1 NCs, ZIF-8, and Ag28Au1 NCs@ZIF-8 because light absorption is a prerequisite for photodynamic bacterial eradication. The UV-visible diffuse reflection spectroscopy (DRS) results reveal that the pristine ZIF-8 sample has poor light harvesting ability in the visible light region due to its large energy gap (Eg) (Fig. 4a), which could explain its low photodynamic antibacterial activity under visible light illumination. Conversely, Ag28Au1 NCs@ZIF-8 exhibits characteristic peaks of Ag28Au1 NCs (Fig. 1a) and demonstrates excellent light harvesting capability in the visible-light region (400 to 780 nm), indicating that the optical absorption capability of Ag28Au1 NCs@ZIF-8 is mainly derived from Ag28Au1 NCs. Although the encapsulation of Ag28Au1 NCs into ZIF-8 had no significant effect on enhanced light absorption of Ag28Au1 NCs, it significantly promotes the separation of photoinduced charge carriers in Ag28Au1 NCs, which is another important factor in determining photodynamic antibacterial activity. As shown in Fig. 4b, an apparent quenching effect on the highly luminescent Ag28Au1 NCs was observed after the introduction of ZIF-8. Moreover, the photostability of Ag28Au1 NCs significantly improved when encapsulated into ZIF-8. Fig. S10† shows that the characteristic peaks of Ag28Au1 NCs gradually disappeared under light irradiation within 5 min, implying the destruction of the basic structure of Ag28Au1 NCs. This result suggests that the pristine Ag28Au1 NCs possess poor photostability, which is responsible for the low photodynamic antibacterial activity. Encouragingly, the encapsulation of Ag28Au1 NCs into ZIF-8 resulted in excellent stability against light irradiation for 210 min (Fig. 4c), showcasing the effectiveness of this encapsulation strategy in improving the photostability of metal NCs. These results imply that the encapsulation of Ag28Au1 NCs into ZIF-8 not only promotes the separation of photoinduced charge carriers in Ag28Au1 NCs but also improves the photostability of Ag28Au1 NCs.
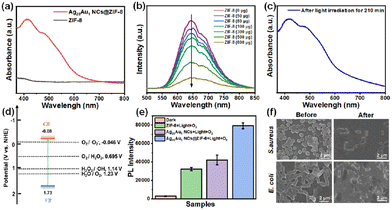 |
| Fig. 4 (a) UV-visible DRS spectra of Ag28Au1 NCs@ZIF-8 and ZIF-8. (b) PL emission spectra of Ag28Au1 NC solution in the presence of different amounts of ZIF-8. (c) UV-visible DRS spectrum of Ag28Au1 NCs@ZIF-8 after light irradiation for 210 min. (d) The diagram of energy levels of Ag28Au1 NCs@ZIF-8. (e) The PL intensity of DCF treated with PBS under dark conditions (control group), and that with antibacterial agents under light irradiation and O2 bubbling for 20 min. (f) SEM images of the bacteria before and after the photodynamic antibacterial test. | |
The conduction band (CB) and valence band (VB) levels of Ag28Au1 NCs@ZIF-8 were measured as they are crucial in determining ROS generation, given that ROS (superoxide (O2˙−), hydrogen peroxide (H2O2), and hydroxyl radicals (˙OH)) are the main active species for bacterial eradication. The energy gap (Eg) and the VB level of Ag28Au1 NCs@ZIF-8 were determined to be 1.81 eV and 1.73 eV (vs. normal hydrogen electrode (NHE)) according to the UV-visible DRS (Fig. S11†) and VB-XPS (Fig. S12†), respectively. Subsequently, the calculated CB level of Ag28Au1 NCs@ZIF-8 was found to be −0.08 eV (vs. NHE), indicating a thermodynamically favorable condition for the generation of O2˙− and H2O2via O2 reduction, as it is more negative than the potentials for O2 reduction (O2/O2˙−, −0.046 V vs. NHE, and O2/H2O2, 0.695 V vs. NHE) (Fig. 4d). Additionally, the VB level is higher than the potentials for H2O and H2O2 oxidation (H2O/O2, 1.23 V vs. NHE, H2O2/˙OH, 1.14 V vs. NHE), and it is sufficient to drive H2O oxidation for O2 generation and H2O2 oxidation for ˙OH generation. To evaluate the production of ROS over various samples under light irradiation, the luminescent 2′,7′-dichlorofluorescein (DCF) was used as an indicator. The PL intensity of DCF treated with Ag28Au1 NCs@ZIF-8 was significantly higher than that of other groups (Fig. 4e), indicating the highest amount of ROS production. This can be attributed to the encapsulation of Ag28Au1 NCs into ZIF-8 which enhances their photostability and promotes the separation of photoinduced charge carriers. Consequently, the results of the ROS generation assay explain the highest photodynamic antibacterial efficiency of Ag28Au1 NCs@ZIF-8. Furthermore, the morphologies of bacteria before and after photodynamic bactericidal treatment were observed using a scanning electron microscope (SEM), revealing drastic changes in the morphologies of S. aureus (round) and E. coli (rod-shaped) from plump, smooth, and intact to collapsed, wrinkled, and cracked (Fig. 4f). These changes can be attributed to the destruction and oxidation of the bacterial cell membrane by photo-induced ROS, ultimately leading to their inactivation.
3.4.
In vivo wound healing assessment
Based on the rapid bacterial eradication achieved by Ag28Au1 NCs@ZIF-8, the therapeutic ability of Ag28Au1 NCs@ZIF-8 (dispersed in carbomer, a commercially available hydrogel) was investigated using S. aureus-infected wounds as a model (Fig. 5a). Before that, the cytotoxicity of various antibacterial agents was assessed on HeLa cells, and the results demonstrated good viability of HeLa cells (89.8 ± 1.6%) after treatment with the hydrogel of Ag28Au1 NCs@ZIF-8 for 24 hours as depicted in Fig. S13.† This can be attributed to the natural biocompatibility of carbomer and the low toxicity of Ag28Au1 NCs@ZIF-8. The wound healing process was recorded using a digital camera, and the images obtained are presented in Fig. 5b. It was observed that all wound sizes began to decrease after 3 days of treatment (Fig. 5c). Notably, the wound size of mice treated with Ag28Au1 NCs@ZIF-8 was smaller than that in other groups on day 3, indicating efficient infection control attributed to the rapid bacterial eradication activity of Ag28Au1 NCs@ZIF-8. By day 7, the wound size of mice treated with Ag28Au1 NCs@ZIF-8 decreased to 6.3 ± 3.1 from 52.3 ± 1.7 mm2 (Fig. 5d), with a wound healing rate of 88.0% (Fig. 5e). In contrast, the wound sizes of the control group and those treated with ZIF-8 and Ag28Au1 NCs were 17.0 ± 2.6, 11.8 ± 3.0, and 10.9 ± 1.5 mm2, respectively, with wound healing rates of 72.8%, 81.8%, and 82.4%. After 11 days, only the wound treated with Ag28Au1 NCs@ZIF-8 was completely repaired and grew new epidermal tissue, while the wounds of the control group and those treated with ZIF-8 and Ag28Au1 NCs remained rough and irregular with 7.9%, 5.8%, and 3.8% of unhealed wound, respectively. Additionally, Ag28Au1 NCs@ZIF-8 showed similar wound size and healing rate to vancomycin (Van), a commercial antibacterial agent, suggesting the great potential of Ag28Au1 NCs@ZIF-8 in clinical application. These findings indicate that Ag28Au1 NCs@ZIF-8 significantly enhances the healing of bacteria-infected wounds by rapidly generating photodynamic ROS for bacterial eradication.
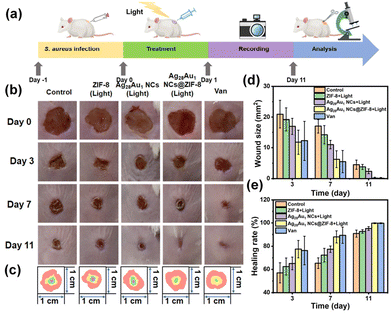 |
| Fig. 5 (a) Schematic illumination of the antibacterial agents for wound healing in vivo. (b) Representative pictures of the incisional skin wounds treated with various antibacterial agents using carbomer as reference (control) at the specified time. (c) The simulation of wound areas in different groups over time. (d) Representative changes in wound size in different groups over time. (e) Representative changes of healing rate in different groups over time. | |
The effect of Ag28Au1 NCs@ZIF-8 on skin regeneration was assessed using hematoxylin and eosin (H&E) and Masson staining. The wounds treated with Ag28Au1 NCs@ZIF-8 displayed the best wound repair effect with respect to inflammatory cells, histological indicators of blood vessels, and the generation of epithelial tissue and collagen. Specifically, the wounds treated with Ag28Au1 NCs@ZIF-8 showed almost no inflammatory cells, while wounds without treatment or those treated with other antibacterial agents still presented some inflammatory cells (Fig. 6a, yellow arrows). This demonstrated that wounds treated with Ag28Au1 NCs@ZIF-8 could efficiently reduce the levels of inflammation, which could be attributed to their rapid photodynamic bacterial eradication activity. This conclusion is further supported by the expression levels of anti-inflammatory factors in wounds. As shown, the expression levels of interleukin-1β (IL-1β, Fig. 6b), tumor necrosis factor-α (TNF-α, Fig. 6c), and interleukin-6 (IL-6, Fig. 6d) in wounds treated with Ag28Au1 NCs@ZIF-8 were lower than those in other groups. Additionally, the distribution of blood vessels in wound tissue was assessed, as blood vessels play a crucial role in accelerating wound healing by transporting oxygen and nutrients. The wounds treated with Ag28Au1 NCs@ZIF-8 have a higher distribution of blood vessels compared to the other groups (red arrows), suggesting that Ag28Au1 NCs@ZIF-8 could promote the generation of new blood vessels in regenerated tissues. Furthermore, the new epithelial tissue in the wound treated with Ag28Au1 NCs@ZIF-8 is more uniform and structured compared with other groups (blue arrows), which is consistent with the observed wound repair results, manifesting the good tissue repair capacity of Ag28Au1 NCs@ZIF-8. Additionally, Ag28Au1 NCs@ZIF-8 showed similar therapeutic effects to Van, as evidenced by the similar H&E and Masson staining results in terms of the level of inflammation, the distribution of blood vessels, and the generation of epithelial tissue and collagen, further supporting the potential of Ag28Au1 NCs@ZIF-8 for clinical applications.
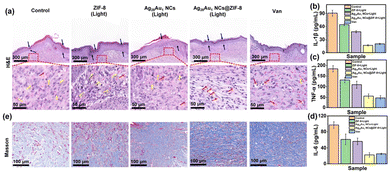 |
| Fig. 6 (a) Representative H&E staining pictures of tissue slices collected from different groups on day 11 (yellow arrows point to the inflammatory cells, red arrows point to the blood vessels and blue arrows point to the epithelial tissue). The expression levels of IL-1β (b), TNF-α (c) and IL-6 (d) in blood collected from different groups on day 11. (e) Representative Masson staining pictures of tissue slices collected from different groups on day 11 (the blue area represents the collagen fiber). | |
The collagen contents (an important indicator to assess the generation stage from the inflammatory period to the recovery period) in the wound area were characterized by Masson staining. As shown in Fig. 6e and Fig. S14,† the collagen content (blue area) in the wound treated with Ag28Au1 NCs@ZIF-8 was higher compared to that in the other groups. Moreover, collagen in wounds treated with Ag28Au1 NCs@ZIF-8 exhibited orderly arranged and dense structures, while collagen in the other groups was in a degradation state, which might be due to the destruction by collagenase secreted by bacteria. Overall, the encapsulation of AIE-type Ag28Au1 NCs into ZIF-8 achieved rapid bacterial eradication, reduced the expression of inflammatory factors, and promoted the generation of new blood vessels, collagen, and epithelial tissue, thus demonstrating a favorable wound repair effect.
4. Conclusion
In summary, we have successfully developed an AIE-type metal NC-based photodynamic antibacterial agent for rapid bacterial eradication to facilitate wound healing under visible light irradiation by encapsulating AIE-type Ag28Au1 NCs into porous ZIF-8. It was found that the encapsulation strategy is beneficial to improving photostability and the separation of charge carriers in Ag28Au1 NCs, thereby facilitating the generation of destructive ROS for bacterial killing. As a result, the as-developed Ag28Au1 NCs@ZIF-8 demonstrated superior photodynamic antibacterial efficacy, killing 97.7% of E. coli and 91.6% of S. aureus within 5 min under visible light irradiation. Moreover, Ag28Au1 NCs@ZIF-8 showed good efficacy in the treatment of bacteria-infected wounds because of their rapid antibacterial action, antiinflammation, and ability to stimulate blood vessel and epithelial tissue growth. The strategy and design presented in this work may inspire the development of other metal NC-based photodynamic antibacterial agents for enhancing wound healing.
Ethics statement
All animal procedures were performed in accordance with the Guidelines for Care and Use of Laboratory Animals of Qingdao University of Science and Technology and approved by both the Animal Ethics Committee of Qingdao University of Science and Technology and the Animal Ethics Committee of Qingdao Zhong Hao Biological Engineering Co., Ltd (Qingdao, China).
Data availability
The data that support the findings of this study are available from the corresponding author (Haiguang Zhu) upon reasonable request.
Conflicts of interest
There are no conflicts to declare.
Acknowledgements
This work is supported by the National Natural Science Foundation of China (22071127, 22371153), the Taishan Scholar Foundation (tsqn201812074, China), and the Youth Innovation Team Development Program of Shandong Higher Education Institutions (2022KJ155).
References
- S. R. Barman, S.-W. Chan, F.-C. Kao, H.-Y. Ho, I. Khan, A. Pal, C.-C. Huang and Z.-H. Lin, Sci. Adv., 2023, 9, eadc8758 CrossRef CAS PubMed.
- Z. Lu, S. Du, J. Li, M. Zhang, H. Nie, X. Zhou, F. Li, X. Wei, J. Wang, F. Liu, C. He, G. Yang and Z. Gu, Adv. Mater., 2023, 35, 2303388 CrossRef CAS.
- W. Zhou, Z. Duan, J. Zhao, R. Fu, C. Zhu and D. Fan, Bioact. Mater., 2022, 17, 1–17 CAS.
- Y. Meng, L. Chen, Y. Chen, J. Shi, Z. Zhang, Y. Wang, F. Wu, X. Jiang, W. Yang, L. Zhang, C. Wang, X. Meng, Y. Wu and W. Bu, Nat. Commun., 2022, 13, 7353 CrossRef.
- L. He, Y. Liu, P. Song, F. Feng, G. Li, Y. Ma, J. Cui, X. Ma, J. Shen and J. Zhang, ACS Sustainable Chem. Eng., 2023, 11, 11529–11540 CrossRef CAS.
- H. Chen, Y. Cheng, J. Tian, P. Yang, X. Zhang, Y. Chen, Y. Hu and J. Wu, Sci. Adv., 2020, 6, eaba4311 CrossRef CAS.
- Y. Hu, H. Zhou, J. Huang, T. Wang, H. Wang, D. Wu, C. Yu, G. Pan, L. Zhang, L. Zhang, D. Gou and J. Zhang, ACS Sustainable Chem. Eng., 2023, 11, 5122–5134 CrossRef CAS.
- J. Wang, Q. Meng, Y. Ma, Y. Yang, R. Zhang, S. Zhong, J. Wang, Y. Gao and X. Cui, ACS Sustainable Chem. Eng., 2023, 11, 18074–18088 CrossRef CAS.
- J. J. Kelly, B. E. Dalesandro, Z. Liu, M. D. Chordia, G. M. Ongwae and M. M. Pires, Angew. Chem., Int. Ed., 2023, 63, e202313870 CrossRef.
- S. Kayama, K. Yahara, Y. Sugawara, S. Kawakami, K. Kondo, H. Zuo, S. Kutsuno, N. Kitamura, A. Hirabayashi, T. Kajihara, H. Kurosu, L. Yu, M. Suzuki, J. Hisatsune and M. Sugai, Nat. Commun., 2023, 14, 8046 CrossRef CAS PubMed.
- A. R. Brochado, A. Telzerow, J. Bobonis, M. Banzhaf, A. Mateus, J. Selkrig, E. Huth, S. Bassler, J. Zamarreño Beas, M. Zietek, N. Ng, S. Foerster, B. Ezraty, B. Py, F. Barras, M. M. Savitski, P. Bork, S. Göttig and A. Typas, Nature, 2018, 559, 259–263 CrossRef CAS PubMed.
- L. Wang, X. Fan, M. Gonzalez Moreno, T. Tkhilaishvili, W. Du, X. Zhang, C. Nie, A. Trampuz and R. Haag, Adv. Sci., 2022, 9, 2105668 CrossRef CAS.
- B. Ran, L. Ran, Z. Wang, J. Liao, D. Li, K. Chen, W. Cai, J. Hou and X. Peng, Chem. Rev., 2023, 123, 12371–12430 CrossRef CAS PubMed.
- W. Li, C. Wang, Y. Yao, C. Wu, W. Luo and Z. Zou, Trends Chem., 2020, 2, 1126–1140 CrossRef CAS.
- N. Yang, C. Cao, X. Lv, T. Zhang, J. Shao, X. Song, W. Wang, P. Chen, W. Huang and X. Dong, BMEMat, 2023, 1, e12005 CrossRef.
- B. Ran, Z. Wang, W. Cai, L. Ran, W. Xia, W. Liu and X. Peng, J. Am. Chem. Soc., 2021, 143, 17891–17909 CAS.
- S. Wang, W.-Y. Wu, J. C. C. Yeo, X. Y. D. Soo, W. Thitsartarn, S. Liu, B. H. Tan, A. Suwardi, Z. Li, Q. Zhu and X. J. Loh, BMEMat, 2023, 1, e12021 CrossRef.
- Y. Genji Srinivasulu, Q. Yao, N. Goswami and J. Xie, Mater. Horiz., 2020, 7, 2596–2618 RSC.
- M. A. Abbas, P. V. Kamat and J. H. Bang, ACS Energy Lett., 2018, 3, 840–854 CrossRef CAS.
- B. Weng, K.-Q. Lu, Z. Tang, H. M. Chen and Y.-J. Xu, Nat. Commun., 2018, 9, 1543 CrossRef.
- H. Wang, X. Liu, W. Yang, G. Mao, Z. Meng, Z. Wu and H.-L. Jiang, J. Am. Chem. Soc., 2022, 144, 22008–22017 CrossRef CAS.
- H. Shan, J. Shi, T. Chen, Y. Cao, Q. Yao, H. An, Z. Yang, Z. Wu, Z. Jiang and J. Xie, ACS Nano, 2023, 17, 2368–2377 CrossRef CAS PubMed.
- F. Hu, R. L. He, Z. J. Guan, C. Y. Liu and Q. M. Wang, Angew. Chem., Int. Ed., 2023, 62, e202304134 CrossRef CAS PubMed.
- S.-S. Zhang, S. Havenridge, C. Zhang, Z. Wang, L. Feng, Z.-Y. Gao, C. M. Aikens, C.-H. Tung and D. Sun, J. Am. Chem. Soc., 2022, 144, 18305–18314 CrossRef CAS PubMed.
- Z. Xie, P. Sun, Z. Wang, H. Li, L. Yu, D. Sun, M. Chen, Y. Bi, X. Xin and J. Hao, Angew. Chem., Int. Ed., 2020, 59, 9922–9927 CrossRef CAS.
- Z. Yin, Z. Wang, X. Dai, N. Liu, S. Wang, G. Li, F. Du and X. Yuan, ACS Sustainable Chem. Eng., 2020, 8, 15336–15343 CrossRef CAS.
- K. Zheng and J. Xie, ACS Nano, 2020, 14, 11533–11541 CrossRef CAS.
- D. Liu, W. Du, S. Chen, X. Kang, A. Chen, Y. Zhen, S. Jin, D. Hu, S. Wang and M. Zhu, Nat. Commun., 2021, 12, 778 CrossRef CAS.
- C. Yao, C. Q. Xu, I. H. Park, M. Zhao, Z. Zhu, J. Li, X. Hai, H. Fang, Y. Zhang, G. Macam, J. Teng, L. Li, Q. H. Xu, F. C. Chuang, J. Lu, C. Su, J. Li and J. Lu, Angew. Chem., Int. Ed., 2020, 59, 8270–8276 CrossRef CAS.
- M. Sugiuchi, J. Maeba, N. Okubo, M. Iwamura, K. Nozaki and K. Konishi, J. Am. Chem. Soc., 2017, 139, 17731–17734 CrossRef CAS.
- X. H. Wu, P. Luo, Z. Wei, Y. Y. Li, R. W. Huang, X. Y. Dong, K. Li, S. Q. Zang and B. Z. Tang, Adv. Sci., 2018, 6, 1801304 CrossRef.
- Y. Yu, Z. Luo, D. M. Chevrier, D. T. Leong, P. Zhang, D.-E. Jiang and J. Xie, J. Am. Chem. Soc., 2014, 136, 1246–1249 CrossRef CAS.
- Y. E. Shi, J. Ma, A. Feng, Z. Wang and A. L. Rogach, Aggregate, 2021, 2, e112 CrossRef CAS.
- S. Qian, Z. Wang, Z. Zuo, X. Wang, Q. Wang and X. Yuan, Coord. Chem. Rev., 2022, 451, 214268 CrossRef CAS.
- Y. Wang, Z. Zuo, Z. Wang, Y. Wu, J. Linghu, Y. Liu, H. Zhu, X. Dou, T. Feng and X. Yuan, Chem. Eng. J., 2024, 492, 152216 CrossRef CAS.
- Y. Duan, J. Luo, S. Zhou, X. Mao, M. W. Shah, F. Wang, Z. Chen and C. Wang, Appl. Catal., B, 2018, 234, 206–212 CrossRef CAS.
- K. Liu, L. Wang, S. Li, H. Liu, D. Zhang, M. Jiang, W. Chen, F. Jiao, X. Zhang and W. Hu, Adv. Funct. Mater., 2023, 33, 2306871 CrossRef CAS.
- M. Wang, L. Nian, Y. Cheng, B. Yuan, S. Cheng and C. Cao, Chem. Eng. J., 2021, 426, 130832 CrossRef CAS.
- H. Lee, W. S. Chi, M. J. Lee, K. Zhang, F. Edhaim, K. Mizrahi Rodriguez, S. J. A. DeWitt and Z. P. Smith, Adv. Funct. Mater., 2022, 32, 2207775 CrossRef CAS.
- Z. Liu, Y. Yi, S. Wang, H. Dou, Y. Fan, L. Tian, J. Zhao and L. Ren, ACS Nano, 2022, 16, 16549–16562 CrossRef CAS PubMed.
|
This journal is © The Royal Society of Chemistry 2024 |
Click here to see how this site uses Cookies. View our privacy policy here.