DOI:
10.1039/D4NR02433H
(Communication)
Nanoscale, 2024,
16, 15984-15994
Repurposing an antimicrobial peptide for the development of a dual ion channel/molecular receptor-like platform for metal ion detection†
Received
12th June 2024
, Accepted 5th August 2024
First published on 9th August 2024
Abstract
The presence of non-essential metals in the environment as contaminants is prone to cause hazardous health problems following accumulation in the human body and the ensuing toxic effects. This calls for continuous discovery and innovation in the realm of developing easy-to-operate, cheap and sensitive sensors. Herein, we describe the proof of concept approach for designing a molecular receptor-like, chimeric sensor based on the pore-forming peptide alamethicin (Alm), tethered via a linker with an ultrashort peptide nucleic acid (PNA) moiety, capable of generating functional ion channel oligomers in planar lipid membranes. The working principle of the sensor exploits the ability of Hg2+ ions to complex mismatching thymine–thymine sequences between the PNA receptor moiety on Alm oligomers and free, thymine-based, single-stranded DNAs (ssDNAs) in solution, thus creating a stable base pair at the oligomer entrance. This generates a transducing mechanism which converts the metal ion complexation into a specific electrical signature of the self-assembled Alm oligomers, enabling selective Hg2+ ion detection. The platform is programmable, whereby the simple exchange of the PNA sequence and its ssDNA counterpart in solution rendered the system selective for Cu2+ ion detection. With further optimization, the presented solution has the potential to translate into miniaturized, cost-effective biosensors suitable for the real-time, label-free and continuous detection of metal ions or other biomolecules.
1. Introduction
In addition to unquestionable economic and societal benefits, the rapid growth over the last few decades of industrial development and the advent of modern technologies have led to dire consequences on the environment through the release of nonessential toxic heavy metal ions, especially Hg2+ ions, as contaminants. The prolonged or systematic exposure and bioaccumulation of such non-degradable contaminants trigger harmful effects and lead to illnesses associated with the central nervous system, the immune system, various organ failure or cancer and cardiovascular diseases.1–5
The stringent demand for the design of real-time, reagentless and noninvasive Hg2+ sensitive detectors remains a fundamental challenge despite the existing rich detection strategies involving spectroscopy, colorimetry, voltammetry or impedimetric approaches.6–25 Apart from their technological maturity, most of the existing detection techniques involve multi-step processes for Hg2+ assessment (i.e., sample collection and pretreatment), rendering them complex to deploy, labor-intensive, and time-consuming and they often require advanced laboratories. These challenges preclude their use for the development of fast-tracking sensors suited for real-time monitoring and assessment in remote sites from the analytical laboratory unit. To mitigate these, there is a constant need for new generations of techniques that embody essential qualities such as cost-effectiveness, accuracy, and portability.
Fortunately, nanopore-based, single-molecule biosensors have evolved considerably over the past decades as powerful tools and hold great promise for advancing single-molecule detection and analysis in applications covering a large variety of analytes, including but not restricted to stochastic sensing of metal ions.26–28
In one of its original, most useful configurations for metal detection purposes, the nanopore system implicated a hetero-heptameric α-hemolysin (α-HL) protein containing a single metal-binding site engineered in the lumen, whose reversible interaction with metals generated stochastic blockades of the ionic current recorded through the voltage-biased nanopore. The off-line analysis of such blockade events was useful for the multiplex detection and quantification of metal ions.29 As a distinct innovative strategy, various biomolecules working as chelating agents for distinct metals were coupled with nanopores to achieve sensitive and selective detection of metal ions. For example, it was known that DNA molecules interact with metal ions,30,31 and inspired by the specific binding of Hg2+ ions to thymine–thymine base pairs (T–Hg2+–T),32,33 T-rich ssDNA probes were designed, which turned into stable hairpin structures upon interaction with Hg2+, leading to a distinct current response and metal detection.34,35
In a different approach based on the same chemical mechanism, two DNA strands with thymine–thymine (T–T) mismatches were rationally designed, and their Hg2+-mediated hybridization ensued a characteristic ion current blockade whilst interacting with α-HL nanopores, thus enabling specific Hg2+ detection.36 By exploiting the metal-induced folding of guanine-rich DNA probes into G quadruplex structures, and their subsequent detection with an α-HL nanopore, the same concept proved useful for the simultaneous detection of Pb2+ and Ba2+ ions.37 Witness to its versatility, the same paradigm of employing metal chelating molecules as probes to bind specific metal ions, then interact with a single nanopore, generate specific ionic current blockades and eventually accomplish metal detection was extended to other substrates such as polypeptides,38–44 small organic molecules45–48 or enzymes.49,50
Despite the successes in detecting and characterizing various analytes at the uni-molecular level, nanopore-based technologies are often affected by experimental drawbacks, including (i) difficulties in isolating and maintaining a single functional nanopore in a free-standing lipid bilayer and (ii) the rate at which molecules are captured, which governs the throughput of blockade events collection and hence the statistical accuracy of the target recognition, is known to be detrimentally affected by: adsorption processes, electroosmosis contributions or even the (sometimes) reduced temporal resolution, in nanopore experiments conducted with widespread amplifiers.51
To alleviate these, we set to deliver a solution bridging the desirable qualities such as the elimination of the need for attaining a single nanopore in a supporting lipid membrane and clustering of active probe surfaces to trigger the engagement of multiple spatially constrained probe–target interactions, leading finally to metal detection. In line with these goals and building up on the previous work in which synthetic ion channels based on gramicidin A were proposed for analyte detection,52–59 we integrated peptide nucleic acids (PNA) on alamethicin (Alm), generating ion channels with dynamic pore sizes. The oligomerization of the resulting (Alm-PNA) chimeras in lipid membranes was found to be controlled by conformational changes following Alm-PNA–ssDNA target recognition events, thus facilitating real-time sensing of short ssDNAs in aqueous solution.60
Herein, we extend this discovery and propose a novel class of sensing elements based on structure-switching (Alm-PNA) chimeras, with the ability to detect aqueous Hg2+ ions. At its core, the solution was based on appending ultra-short thymine (T)-rich PNA probes on Alm peptides via a flexible linker, generating chimeric Alm-PNA molecules. Under optimal conditions, such constructs successfully co-assemble in planar lipid membranes, forming diameter-variable oligomers while maintaining the PNA probe in the proximity of the oligomer opening.
As a proof of concept, we showcased that the Hg2+ ion-mediated hybridization of T-rich PNA moieties from Alm-PNA monomers with mismatched T-rich ssDNA targets present in the aqueous solution vigorously altered Alm-PNA chimera assembly and the mediated ionic current, thus favoring Hg2+ detection even in the presence of other metals. By changing the sequence of the PNA moiety, this approach enabled the detection and differentiation of Cu2+ ions. We posit that this strategy may be useful for generating highly programmable biosensors, underlining their feasibility for applications including waste management, environmental, or biological monitoring.
2. Results and discussion
2.1 Construction principles of the Alm-PNA chimera and proof of concept of active Alm_T oligomer generation via electrical recordings
We rationally designed Alm-PNA modular constructs consisting of a membrane-active domain, endowed by a modified Alm backbone, and a linker domain connecting a PNA sequence working as a probe to detect Hg2+ ions at the Alm C-terminus (Fig. 1, I, a) (for further details see the ESI†).
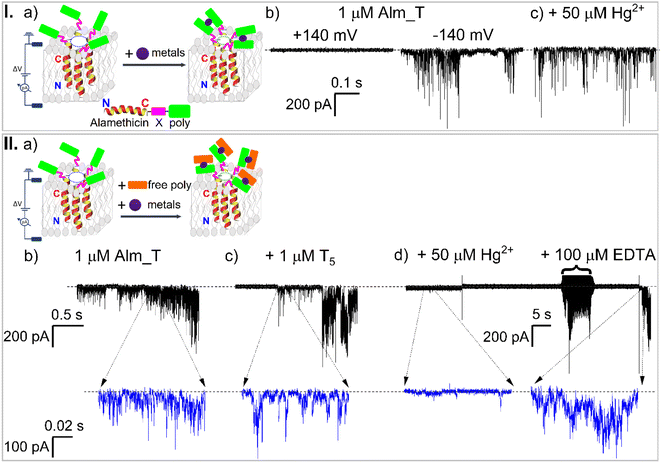 |
| Fig. 1 Schematic representation of the detection strategy. (I) (a) Mutated variants of Rf30 alamethicin (Alm) were engineered at the C-terminus, via suitable (Gly–Gly–Ser–Gly) linkers (in purple, in the inset), with either poly(T)5 or poly(G)5 PNA segments (in green, in the inset). When added on the grounded, cis side of a lipid membrane subjected to a negative potential (ΔV) on the trans side, Alm-PNA chimeras – denoted herein by Alm_T or Alm_G – insert across the lipid membrane with the N-terminus head-on, oligomerize under the applied potential (ΔV), generate functional ion channel-like receptors with the PNA segments exposed to the bulk electrolyte, and mediate ionic current fluctuations across the membrane. As hypothesized, the oligomer-mediated ionic currents were likely to be affected by intermolecular T5 PNA–Hg2+–T5 PNA interactions taking place on the same oligomer. (b) Selected electrophysiology traces displaying the Alm_T oligomer activity at trans positive or negative potentials, as indicated. The ionic current fluctuations through functional oligomers at negative potentials were recorded as downwardly oriented spikes. (c) Subsequent cis side addition of Hg2+ [50 μM] does not interfere with oligomer activity recorded at ΔV = −140 mV. (II) (a) The cartoon model of Hg2+-induced complexation of freely added ssDNA fragments (denoted by free poly, in orange) with Alm_T oligomers, indicating that the metal-driven hybridization of T5 ssDNAs (free poly) with dangling PNA segments from functional Alm_PNA oligomers would trigger conformational changes on the Alm_T oligomer. Selected recordings illustrating the electrical currents mediated by Alm_T oligomers at ΔV = −140 mV before (b) and after T5 ssDNA addition at a 1 : 1 molar ratio, on the cis chamber (c). Subsequent cis side addition during the same experiment of Hg2+ [50 μM] leads to a considerable decrease in the Alm_T activity (d), whereas cis side addition of EDTA [100 μM] restores the initial activity of Alm_T oligomers. All experiments were carried out in an electrolyte containing 3 M KCl buffered at pH = 7. | |
For the Alm-PNA chimeras used herein, we posit a working mechanism similar to the most accepted one applicable to the native Alm,61 which involves the interaction of the peptide's dipole moment with the transmembrane electric fields, leading to the transition from a surface-bound to an inserted orientation followed by the transmembrane lateral aggregation and reversible association of monomers into functional oligomers (Fig. 1, I, a). A first glimpse of encouragement came at the end of a series of screening experiments, whereby we demonstrated that the application of trans negative potentials led to the vigorous electrical behavior of the engineered Alm_T chimeras in planar lipid membranes (Fig. 1, I, b). We also concluded that the multiple conductance levels associated with the oligomer electrical activity, evidenced in Fig. 1, I, b, constituted a good proof of the continuous, reversible accretion of Alm_T monomers to an oligomer, resembling most likely the ‘barrel-stave’-like topology.
2.2 Hg2+ metal detection with Alm_T oligomers
To delineate our detection strategy, we hypothesized that in the presence of Hg2+ ions added on the cis side of a membrane containing functional Alm_T oligomers, the metal ions would complex neighboring intermolecular T5 PNA moieties belonging to the same oligomer and alter its overall kinetics and/or conductive properties, thus signaling the presence of Hg2+ ions (Fig. 1, I, a). To our dismay, the experimental evidence contradicted this hypothesis, as Hg2+ addition to the aqueous electrolyte bathing the Alm_T oligomers did very little to their overall kinetic and transport activity (Fig. 1, I, c). The inability of Hg2+ ions to mediate the formation of mismatched T–Hg2+–T duplexes between adjacent T5 PNA moieties on active oligomers indicates that the relative compactness of the Gly–Gly–Ser-based linkers62 precludes the favorable intermolecular T5 PNA domain–domain interactions within individual Alm_T oligomers.
Hence, we shifted our focus and reasoned instead that Hg2+-induced complexation of T5 PNA moieties on active oligomers with thymine-rich mismatched ssDNA (T5) dissolved in the same electrolyte could be another way to achieve Hg2+ ion detection. The underlying hypothesis was that due to their spatial mobility in the electrolyte, the T5 ssDNA fragments would be prone to be oriented more easily and achieve efficient Hg2+-induced complexation to the T5 PNA probes on the lipid membrane-embedded Alm_T oligomers (Fig. 1, II, a). In this scenario though, new control experiments were needed to shed light on any putative T5 ssDNA–Alm_T interactions, whose manifestation would interfere with actual Hg2+-induced blockade events. Nevertheless, as all electrophysiology experiments were carried out at trans negative potentials, as needed to trigger Alm_T activity in membranes, we had little reason to believe that the anionic T5 ssDNA fragments would be drawn electrophoretically near membrane-embedded Alm_T oligomers, and behave as non-specific blockers.
The collected data demonstrated that in the presence of thymine-rich mismatched ssDNA (T5) added to the cis electrolyte, the Alm_T oligomer activity remained largely unchanged (Fig. 1, II, b and c), underpinning the near absence of non-specific Alm_T–T5 interactions. Remarkably, the subsequent cis side addition of Hg2+ triggered a dramatic decrease of Alm_T oligomer activity and the effect was fully reversible, as further addition of EDTA recovered the oligomer activity (Fig. 1, II, d). We concluded at this point that the recorded decrease of Alm_T activity was very likely due to the Hg2+-mediated, duplex formation between the T5 ssDNAs and respective PNA moiety on Alm_T fragments. Hence, the resulting complexes affected subsequent membrane oligomerization of Alm_T chimeras and ionic transport through the formed oligomers, and thereby indirectly signaled Hg2+ presence.
As a next logical step, we sought to elucidate in subsequent experiments the specificity of Alm_T–Hg2+–T5 interactions pertaining to successful Hg2+ detection. To test this, we monitored the effect of Hg2+ added together with guanine-containing short polynucleotides (G5) on the Alm_T activity. The data displayed in Fig. S1† demonstrate that successive cis side additions during the same experiment of Hg2+ and G5 ssDNA on a membrane containing Alm_T oligomers contributed little to the overall kinetic and transport activity of embedded Alm_T oligomers. This is indicative of the absence of non-specific interactions between the Alm_T oligomers and Hg2+ ions or G5 ssDNA. Interestingly, further addition during the same experiments of T5 ssDNA fragments led again to a vigorous decrease in oligomer activity (Fig. S1d†), demonstrating that the Alm_T–Hg2+–T5 complexation occurs regardless of the presence of the non-competitive G5 ssDNA in the buffer. As a quantitative analysis, the integrated values of the power spectra of current fluctuations recorded under the conditions specified above (Fig. S1e†) and respectively the I–ΔV diagrams drawn under similar circumstances (Fig. S1f†) provide an estimate of the inhibitory effect triggered by the concomitant presence of T5 ssDNA and Hg2+ metals on the Alm_T activity. This is in accordance with previous findings, which established that duplex formation with the T–T mismatch upon the addition of Hg2+ is highly specific.63
Subsequent experiments carried out at different transmembrane potentials and over a wider range of cis-added Hg2+ metals confirmed unambiguously Hg2+ detection (Fig. 2).
 |
| Fig. 2 Hg2+ concentration- and voltage-driven effects on the Alm_T–Hg2+–T5 activity recorded in planar lipid membranes. While bathed together with T5 fragments at a 1 : 1 molar ratio (a), subsequent addition of Hg2+ with incremental concentrations (b–d) led to the continuous decrease of Alm_T oligomer activity, as recorded at ΔV = −100 mV. The downward spikes designate the electrical currents mediated by functional oligomers. Adjacent to each recording we display representative zoomed-in excerpts and the all-point histograms of ionic current fluctuations. (e) The voltage-dependent ionic transport through the membrane residing, self-assembled oligomers, revealing disruption of Alm_T self-assembly (or channel blockage) as a function of Hg2+ concentration. (f) Alm_T–Hg2+–T5 complex activity is dependent on the applied holding potentials and Hg2+ concentration. At the used transmembrane potentials (ΔV), the reported inhibition values were calculated as percentages of the integrated power spectra of ionic current fluctuations corresponding to various concentrations of the cis side added Hg2+ relative to the initial state ([Hg2+] = 0). The sigmoidal fits of Alm_T–Hg2+–T5 activity percent inhibition vs. [Hg2+] yielded the mean ± S.E.M. values for the half maximal effective concentration (EC50) and Hill slope (p) of the curves (see also Table 1). All experiments were carried out in an electrolyte containing 3 M KCl buffered at pH = 7. | |
As reflected by the zoomed-in traces and all-point histograms presented in Fig. 2a–d, the Hg2+ effect on individual conductance substrates – indicative of reversible Alm_T monomer accretion to an existing oligomer under various experimental conditions – was hardly discernible, but easily seen in the I–ΔV diagrams (Fig. 2e) or via fluctuation analysis (vide infra). Thus, in order to efficiently quantify the metal detection capability in our experiments, we resorted to a simpler strategy involving the power-spectrum density analysis of the ionic current fluctuations, specified representatively in Fig. 2a–d, from which we derived the average power in the corresponding current fluctuations over the 1–5000 Hz frequency domain, in the absence and presence of various metals or reagents. We later fitted the concentration dependence of the (Alm_T–Hg2+–T5) complex activity – quantified as the average power of ionic current fluctuations – at three different applied potentials, to a dose–response curve (Fig. 2f)
.64 In this expression, ymin and ymax refer to the lower and respectively upper asymptotic value of the sigmoidal function, respectively, x corresponds to [Hg2+] in the bath, EC50 is the half maximal effective concentration and p is the Hill slope of the curve. At the fit initialization, we set ymin to 0, because we considered that at vanishingly small Hg2+ concentrations, the modification in the (Alm_T–Hg2+–T5) complex activity is virtually absent. The results of the best fit analysis are shown in Table 1.
Table 1 The parametrization of the [Hg2+]- and ΔV-dependent Alm_T–Hg2+–T5 activity through the Hill equation fitted to the experimentally derived dose–response curves
|
ΔV = −100 mV |
ΔV = −120 mV |
ΔV = −140 mV |
EC50 (μM) (average ± S.E.M.) |
20.1 ± 1.3 |
12 ± 1.2 |
32.3 ± 1.9 |
p (average ± S.E.M.) |
3.3 ± 0.6 |
2.2 ± 0.5 |
2.6 ± 0.4 |
As evidenced by the data shown in Table 1, the EC50 of the Hg2+ interaction with the presented system lies in a range of tens of μM at the different transmembrane potentials employed, and the corresponding threshold for detection is higher (μM range) as compared to those of other sensors based on the T–Hg2+–T coordination chemistry, lying between tens of nM to 100 nM.12,32,34
There are a number of reasons that could explain the low sensitivity of our detection solution, indicating also the possible venues for optimization, in order to successfully compete with other established sensors, on the sensitivity scale. The most obvious one is represented by the manifestation of intrinsic current fluctuations of the Alm_T oligomer activity in the absence of metals, which constitute a background noise for Hg2+ detection, particularly in the low range of the metal concentration, and precluding the reliable recognition of Hg2+-mediated signals. The second reason is related to the rather small size of the single-stranded PNA overhang (five thymine bases) serving as a detection probe for the aqueous Hg2+via induced stabilization of correspondingly mismatched T5 ssDNA fragments in solution. A third reason may lie in the peptide linker length and constitution, which control the rotational freedom of the PNA domains engineered on Alm monomers, the steric hindrance between adjacent probes and ultimately the dynamics of changes among different conformations of the Alm_T oligomers coordinated with ssDNA fragments. Last but not least, while it is known that Cl− ions can bind Hg2+ to decrease its effective concentration in aqueous solution,34,65 we recall that in our experiments, the salt solution contained 3 M KCl, which might render the effective free Hg2+ concentration lower than the weighted value.
2.3 Quantifying the detection specificity of Hg2+ with the Alm_T-based chimeras
An important aspect for future consideration of the proposed solution as a useful approach for Hg2+ detection was the elucidation of nonspecific interactions of other metals with functional Alm_T oligomers, which could compromise the detection. To test this, we monitored in different experiments the propensity of the formation of Alm_T–T5 duplexes or Alm_T–metal interactions, in the presence of the cis-added Al3+, Zn2+ and Cu2+. As demonstrated in Fig. 3, successive addition of Al3+ [50 μM] (a), followed by Zn2+ [50 μM] and Cu2+ [50 μM], to an electrolyte containing a mixture of Alm_T [1 μM] and T5 ssDNA [1 μM] left the activity of Alm_T oligomers largely unaffected. This phenomenon remained invariant even when the concentration of the aforementioned metals was doubled during the same experiment (Fig. 3e).
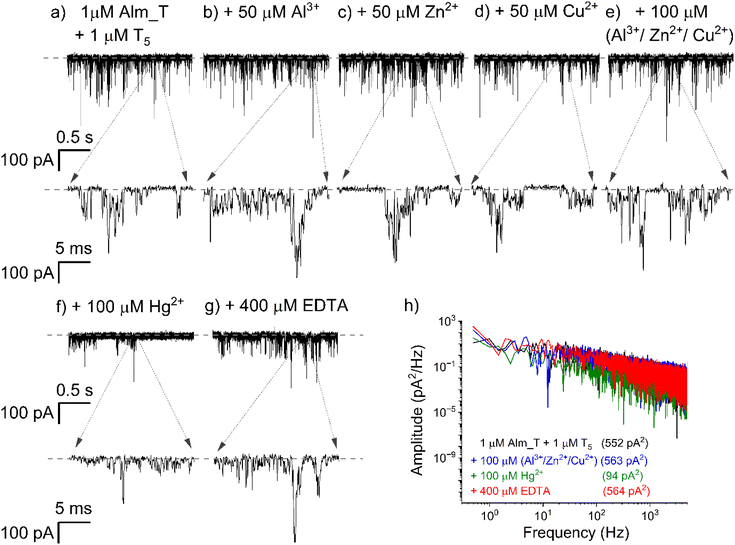 |
| Fig. 3 The real-time metal detection via Alm_T–T5 hybridization is selective to Hg2+. Selected electrical recordings displaying the membrane activity of the Alm_T oligomers in a planar lipid membrane subjected to a potential difference of ΔV = −140 mV, when added together with the T5 ssDNA (1 μM) at a 1 : 1 molar ratio on the cis side of the system (a), and in the presence of successively added Al3+ [50 μM] (b), Zn2+ [50 μM] (c), Cu2+ [50 μM] (d) and respectively all metals at a concentration of 100 μM each (e). The ionic currents mediated by the Alm_T oligomers are represented by the downwardly oriented spikes, seen also in detail in the corresponding zoomed-in excerpts below. (f) Further addition of Hg2+ [100 μM] during the same recording determined a decrease in the Alm_T oligomer activity, which was recovered after EDTA [400 μM] addition (g). (h) The power-spectrum analysis of the ionic current fluctuations shown representatively in traces (a–g) resulted in the average power contained in the corresponding current fluctuations, and are displayed in the corresponding legend. The near superposition of the shown power spectra suggests the putative lack of effect of the reagents tested on the oligomer kinetics. All experiments were carried out in an electrolyte containing 3 M KCl buffered at pH = 7. | |
To our delight, we noted that further addition of Hg2+ [100 μM] indeed led to a dramatic decrease of Alm_T activity (Fig. 3f) – which we attributed to the formation of Alm_T–Hg2+–T5 complexes – and this effect was fully reversible upon the addition of excess EDTA (Fig. 3g). The integrated values of the power-spectra of current fluctuations recorded in the presence of various metals shown in Fig. 3a, e and f provided a quantitative assessment of the Hg2+-induced, selective inhibition of Alm_T oligomer activity and EDTA-triggered recovery (Fig. 3h).
2.4 Feasibility of an alternative chimera design for probing Cu2+ ions
Based on the compelling evidence suggesting the suitability of our system for Hg2+ detection, we set out to investigate the extension and modularity of the proposed paradigm to probing other metal ions. To this end, we used the same detection strategy as above, preserving the overall architecture of the chimeric receptor, but substituted the thymidine in the PNA moiety with guanine bases, which are known to selectively bind Cu2+ ions.66,67
The representative outcome of a series of such experiments is shown in Fig. 4. As a first noticeable observation, we discovered that under the exact same conditions, Alm_G chimeras generated oligomers with a considerably larger activity than Alm_T, so that in order to prevent lipid membrane rupture, their added concentration on the recording setup was diminished by 10-fold.
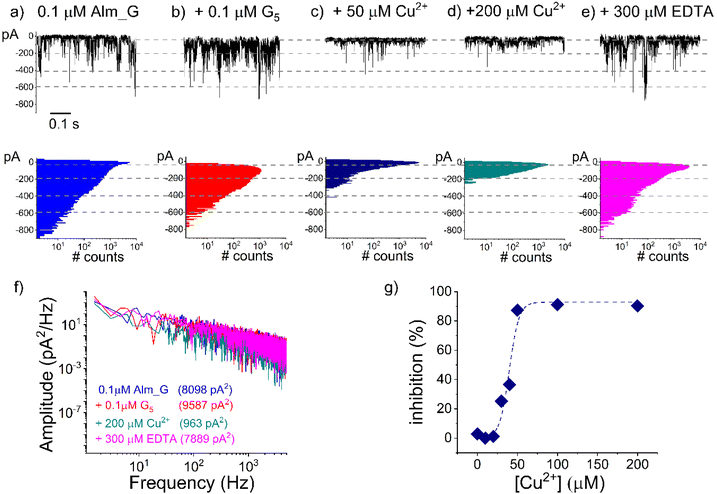 |
| Fig. 4 Cu2+ ions mediate complexation between Alm_G oligomers and G5 ssDNAs in aqueous solution. The ionic current signature of Alm_G chimeras self-assembled as oligomers in planar lipid membranes held at ΔV = −100 mV, in the presence of successive cis side additions at the indicated micro-molar concentrations of G5 ssDNAs (b), Cu2+ metals (c and d) and EDTA (e). In the panels beneath each representative trace, we display the all-point histograms of the corresponding current fluctuations. (f) The Fourier decomposition-based, power-spectrum analysis of the traces is shown in panels (a, b, d and e). In the legend we display the extracted average power values contained in such current fluctuations, calculated as the graph's integrals as indicated above. (g) Alm_G–Cu2+–G5 activity vs. Cu2+ concentration reported as inhibition values which were calculated as percentages of the integrated power spectra of ionic current fluctuations corresponding to various concentrations of the cis side added Cu2+ with respect to the initial state ([Cu2+] = 0). The sigmoidal fits of the experimentally derived percent inhibition vs. [Cu2+] yielded the mean ± S.E.M. values for the half maximal effective concentration (EC50) and Hill slope (p) of the curves (see the text). All experiments were carried out in an electrolyte containing 3 M KCl buffered at pH = 7. | |
Once we pinned down the optimal experimental conditions enabling long-lasting ionic current recordings mediated by Alm_G oligomers (Fig. 4a), we continued by adding G5 ssDNA fragments during the same experiment, and noted the virtual absence of alterations in the recorded current fluctuations (Fig. 4b). As pointed out above, this experimental observation indicated the inability of G5 to interfere with either reversible formation of Alm_G oligomers or ion transport mediated by them. The subsequent Cu2+ addition triggered a sharp decrease in the Alm_G oligomer activity (Fig. 4c and d) and the effect was reversible upon chelating Cu2+ ions with excess EDTA (Fig. 4e). Quantitatively, these traces were analyzed by estimating the integral power spectra of current fluctuations recorded under the indicated conditions (Fig. 4f), later used to draw the concentration dependence of the (Alm_G–Cu2+–G5) complex activity (Fig. 4g). The non-linear fit of the derived dose–response curve done as in the preceding experiments resulted in EC50 = 39.3 ± 2.3 μM and p = 6.1 ± 2. In the absence of additional structural information, we speculate that the increase in the Hill coefficient (p) in Cu2+ detection experiments – which provides a minimum estimate of the number of binding sites involved – as compared to that of Hg2+ (vide supra), may reflect the distinct coordination numbers (4 > 6 for Cu2+ and respectively 2 > 4.6 for Hg2+) and metal-base complex geometries of the two ionic species (square planar for Cu2+ and respectively linear for Hg2+).68
As shown above, to test the nonspecific interactions between other reagents and the sensing element engineered in the sensor's architecture, we undertook additional control experiments, demonstrating that Cu2+ ion detection with the (Alm_G; G5 ssDNA) system is selective. Data shown in Fig. S2† reveal that cis side addition of Hg2+ ions on a lipid membrane containing Alm_G oligomers and bathed in an electrolyte containing G5 ssDNAs contibuted very little to the overall kinetic and transport activity of the embedded oligomers (Fig. S2a and b†). By virtue of a similar rationale as in the case of the presented Alm_T system, this strongly suggests the absence of nonspecific interactions between the Alm_G oligomers and Hg2+ ions alone and reveals the inability of Hg2+ ions to mediate mismatched duplex formation between the G5 PNA moiety and the G5 ssDNA. Subsequent addition of Cu2+ ions led, however, to a clear decrease in the Alm_G oligomer activity (Fig. S2c†), demonstrating that the Alm_G–Cu2+–G5 complexation not only occurs, but it does so regardless of the presence of Hg2+ ions in the electrolyte.
To argue more strongly in favor of the validity of the presented detection strategy, we challenged our system by reversing the addition order of Cu2+ ions, and bathed the Alm_G sensor with T5 instead of the G5 sequence. As displayed in Fig. S3a and b,† the electrical fluctuations elicited by Alm_G oligomers in a lipid membrane were hardly influenced by the presence of the cis-added Cu2+ ions alone. The observed mild increase in the activity (see Fig. S3f,† for quantitative estimations) is associated most likely with re-homogenization effects in the buffer following reagent addition and stirring. This demonstrates that by their presence alone, Cu2+ ions are not capable of generating inter-oligomer Alm_G–Cu2+ complexes and a subsequent decrease in their membrane activity. As suggested above, it still remains to be established whether a longer, more flexible linker connecting the Cu2+ binding moiety on the oligomers (poly G PNA) to the modified alamethicin backbone would result in a more efficient transduction mechanism, needed to convert the physical capture of Cu2+ binding between adjacent Alm_G monomers in an oligomer into a specific electrical signature of the sensor. Further cis side addition of the non-specific T5 fragments leaves the activity of Alm_G oligomers virtually un-altered (Fig. S3c†). This important observation demonstrates the inability of Cu2+ ions to generate Alm_G–Cu2+–T5 complexes, pointing to the presence of G5 fragments as a critical prerequisite for Cu2+ ion detection. As expected, a decrease in Alm_G oligomer activity occurred immediately following G5 addition (Fig. S3d†), in line with data and interpretation provided in Fig. 4, suggesting the formation of Alm_G–Cu2+–G5 complexes, and implicitly, Cu2+ ion detection in such a heterogeneous electrolyte. Furthermore, the Cu2+ ion-mediated formation of Alm_G–G5 complexes, was found to be reversible upon excess EDTA cis side addition (Fig. S3e†), supporting the validity of Alm_G–Cu2+–G5 complex formation as a main cause for the recorded decrease in the oligomers’ electrical activity.
3. Conclusions
To summarize, we propose a novel construct having at its core ultrashort PNA segments appended to a modified Alm peptide, which could be employed as an ion channel-like platform to detect and monitor selectively the presence of metal ions in a heterogeneous electrolyte. We demonstrate that by changing only the sequence of the PNA binding interface on the Alm-PNA chimeric construct, a different binder-containing nanopore sensor can be obtained and readily implemented into detecting specifically Hg2+ or Cu2+ in solution. Speaking in favor of the system's robustness, its stability spans tens of minutes to hours, and under experimental conditions used herein it depends mostly on the initially added concentration of the Alm-PNA construct and the applied ΔV. As we noticed in our experiments, exceedingly larger concentration values beyond those reported (1 μM for Alm_T and respectively 0.1 μM for Alm_G) usually lead to the lipid bilayer destabilization following peptide penetration, especially under larger applied trans-negative potentials. A critical benefit of the proposed solution is facilitated by the modular nature of the sensors. By creating combinatorial libraries of tethered PNA probe binders of varying binding affinity and specificity, our sensors may be further developed to achieve new nanopore sensing systems for concurrently identifying more metal species. Taking into account the concentration threshold set for total mercury values in drinking water by the United States Environmental Protection Agency (USEPA) (2 μg L−1),69 we admit that the Hg2+ detection sensitivity of the sensor presented herein situates below the maximum permitted levels in water. Nevertheless, we remind that the presented proof of concept solution is only a first step in the direction of devising simpler and easier to use optimized constructs, complementing traditional instruments designed for the same goal. Speaking in favor of the presented approach though, one should note that in comparison with the existing methods for Hg2+ detection, the paradigm presented herein has the advantage that it can be harnessed to detect and monitor the presence of metals in solution in real-time, involving a relatively simple to deploy and unexpensive setup, and with minimum preparatory steps on the active reagents. Rough estimates made regarding the cost of the most expensive ingredients needed for the detection experiments described herein, including only Alm-PNA and ssDNA fragments and excluding all other reagents (e.g., buffers, lipids, and solvents), resulted in a price of ∼10 euros per detection trial. It therefore appears that the presented method is quite acceptable in financial terms.
For enhanced Hg2+ detection sensitivity prospects – which are applicable for other metal species as well – future steps may include, for instance, the identification of more flexible linker domains to permit metal-mediated hybridization between any two PNA moieties from Alm-PNA-generated oligomers and eliminate the need for ssDNA presence in the recording electrolyte, thus rendering the detection system even simpler. Additionally, optimized length values of the PNA sequence motifs appended to the Alm backbone to determine more stable Alm_T–Hg2+–T5 complexes in the lower regime of metal concentration may boost detection sensitivity. Last but not least, coupling the presented design with microfluidic systems enabling the washout of Alm chimeras from the recording electrolyte upon oligomer formation in the lipid membrane, and therefore eliminating the generation of the bulk-manifested Alm_T–Hg2+–T5 pairs, which constitute a parallel pathway for target depletion in the recording solution,60 is also expected to improve detection sensitivity.
We envision that the presented strategy is prone to generalization, whereby by tethering various receptor-like moieties, e.g. aptamers, nanobodies or monobodies,70,71 to Alm peptides, generating diameter-variable Alm-based oligomers as presented herein, will produce a library of ion channel-like biosensors whose activity becomes highly target-dependent. This, in conjunction with automated and microfabricated lipid bilayer systems72–74 and the already commercially available technologies for the portable, integrated use of lipid bilayer generation and recording systems (i.e., the bilayer lipid membrane chip from ELEMENTS SRL, Italy), has the potential to improve the stability of membranes, minimize the volume of electrolyte solutions required and provide a more robust lipid membrane-based chimeric sensor.
Author contributions
The manuscript was written through contributions of all authors. All authors have given approval to the final version of the manuscript.
Data availability
The data supporting this article have been included as part of the ESI.† Data are available upon request from the authors.
Conflicts of interest
The authors declare no conflicts of interest.
Acknowledgements
This work was supported by the National Research Foundation of Korea (NRF) grant from the Korean Government (grant numbers 2023R1A2C3006368; RS-2023-00228746, RS-2024-00401422, and RS-2023-00253613) and the Institute for Information & Communications Technology Promotion (IITP) grant from the Korean government (MSIT) (grant number no. RS-2017-II171714, Development of Antimicrobial Peptide using Deep Learning).
References
- M. E. Sears, K. J. Kerr and R. I. Bray, Arsenic, Cadmium, Lead, and Mercury in Sweat: a Systematic Review, J. Environ. Public Health, 2012, 2012, 184745 Search PubMed.
- E. K. Silbergeld, I. A. Silva and J. F. Nyland, Mercury and Autoimmunity: Implications for Occupational and Environmental Health, Toxicol. Appl. Pharmacol., 2005, 207, 282–292 CrossRef PubMed.
- P. Grandjean, P. Weihe, R. F. White and F. Debes, Cognitive Performance of Children Prenatally Exposed to “Safe” Levels of Methylmercury, Environ. Res., 1998, 77, 165–172 CrossRef CAS PubMed.
- R. A. Bernhoft, Mercury Toxicity and Treatment: a Review of the Literature, J. Environ. Public Health, 2012, 2012, 460508 Search PubMed.
- A. Philibert, M. Fillion, J. Da Silva, T. S. Lena and D. Mergler, Past Mercury Exposure and Current Symptoms of Nervous System Dysfunction in Adults of a First Nation Community (Canada), Environ. Health, 2022, 21, 34 CrossRef CAS PubMed.
- F. A. da Silva Cunha, M. J. de Oliveira, P. P. Florez-Rodriguez and J. C. C. Santos, Mercury Speciation in Estuarine Water Using Dithiol-Based Magnetic Solid-Phase Extraction and Cold Vapor Atomic Fluorescence Spectrometry, Spectrochim. Acta, Part B, 2022, 192, 106412 CrossRef CAS.
- M. L. Astolfi, C. Protano, E. Marconi, D. Piamonti, L. Massimi, M. Brunori, M. Vitali and S. Canepari, Simple and Rapid Method for the Determination of Mercury in Human Hair by Cold Vapour Generation Atomic Fluorescence Spectrometry, Microchem. J., 2019, 150, 104186 CrossRef CAS.
- F. Tukur, P. Tukur, S. Hunyadi Murph and J. Wei, Advancements in Mercury Detection Using Surface-Enhanced Raman Spectroscopy (SERS) and Ion-Imprinted Polymers (IIPs): A Review, Nanoscale, 2024, 16, 11384–11410 RSC.
- Q. Li, H. Li, K. Li, Y. Gu, Y. Wang, D. Yang, Y. Yang and L. Gao, Specific Colorimetric Detection of Methylmercury Based on Peroxidase-Like Activity Regulation of Carbon Dots/Au NPs Nanozyme, J. Hazard. Mater., 2023, 441, 129919 CrossRef CAS PubMed.
-
N. Ozbek, A. Baysal and S. Akman, Heavy Metal Determinatıon in Environmental Samples, in Environmental Applications of Instrumental Chemical Analysis, ed. M. Barbooti, Apple Academic Press, New York, 2015, pp. 441–470 Search PubMed.
- X. B. Zhang, R. M. Kong and Y. Lu, Metal Ion Sensors Based on DNAzymes and Related DNA Molecules, Annu. Rev. Anal. Chem., 2011, 4, 105–128 CrossRef CAS PubMed.
- J. S. Lee, M. S. Han and C. A. Mirkin, Colorimetric Detection of Mercuric Ion (Hg2+) in Aqueous Media using DNA-Functionalized Gold Nanoparticles, Angew. Chem., Int. Ed., 2007, 46, 4093–4096 CrossRef CAS PubMed.
- X. F. Liu, Y. L. Tang, L. H. Wang, J. Zhang, S. P. Song, C. H. Fan and S. Wang, Optical Detection of Mercury(II) in Aqueous Solutions by Using Conjugated Polymers and Label-Free Oligonucleotides, Adv. Mater., 2007, 19, 1471–1474 CrossRef CAS.
- Y. Tang, D. Lee, J. Wang, G. Li, J. Yu, W. Lin and J. Yoon, Development of Fluorescent Probes Based on Protection–Deprotection of the Key Functional Groups for Biological Imaging, Chem. Soc. Rev., 2015, 44, 5003–5015 RSC.
- M. Hollenstein, C. Hipolito, C. Lam, D. Dietrich and D. M. Perrin, Highly Selective DNAzyme Sensor for Mercuric Ions, Angew. Chem., Int. Ed., 2008, 47, 4346–4350 CrossRef CAS PubMed.
- Z. Lin, X. Li and H. B. Kraatz, Impedimetric Immobilized DNA-Based Sensor for Simultaneous Detection of Pb2+, Ag+, and Hg2+, Anal. Chem., 2011, 83, 6896–6901 CrossRef CAS PubMed.
- G. Chen, Z. Guo, G. Zeng and L. Tang, Fluorescent and Colorimetric Sensors for Environmental Mercury Detection, Analyst, 2015, 140, 5400–5443 RSC.
- T. Samanta and R. Shunmugam, Colorimetric and Fluorometric Probes for the Optical Detection of
Environmental Hg(II) and As(III) ions, Mater. Adv., 2021, 2, 64–95 RSC.
- Q. Li and Y. Zhou, Recent Advances in Fluorescent Materials for Mercury(II) Ion Detection, RSC Adv., 2023, 13, 19429–19446 RSC.
- G. M. Escandar and A. C. Olivieri, A Critical Review on the Development of Optical Sensors for the Determination of Heavy Metals in Water Samples. The Case of Mercury(II) Ion, ACS Omega, 2022, 7, 39574–39585 CrossRef CAS PubMed.
- M. Schmittel and H. Lin, Quadruple-Channel Sensing: a Molecular Sensor with a Single Type of Receptor Site for Selective and Quantitative Multi-Ion Analysis, Angew. Chem., Int. Ed., 2007, 46, 893–896 CrossRef CAS PubMed.
- K. R. Kim, J. Oh and J. I. Hong, A Photoluminescent and Electrochemiluminescent Probe Based on an Iridium(III) Complex with a Boronic Acid-Functionalised Ancillary Ligand for the Selective Detection of Mercury(II) ions, Analyst, 2023, 148, 5619–5626 RSC.
- L. Prodi, F. Bolletta, M. Montalti and N. Zaccheroni, Luminescent Chemosensors for Transition Metal Ions, Coord. Chem. Rev., 2000, 205, 59–83 CrossRef CAS.
- E. M. Nolan and S. J. Lippard, Tools and Tactics for the Optical Detection of Mercuric Ion, Chem. Rev., 2008, 108, 3443–3480 CrossRef CAS PubMed.
- Z. Yu, Z. Tian, Z. Li, Z. Luo, Y. Li, Y. Li and J. Ren, A New Chromogenic and Fluorogenic Chemosensor for Hg(II) with High Selectivity Based on the Hg2+-Promoted Deprotection of Thioacetals, Sens. Actuators, B, 2016, 223, 172–177 CrossRef CAS.
- O. Braha, B. Walker, S. Cheley, J. J. Kasianowicz, L. Song, J. E. Gouaux and H. Bayley, Designed Protein Pores as Components for Biosensors, Chem. Biol., 1997, 4, 497–505 CrossRef CAS PubMed.
- S. Howorka and Z. Siwy, Nanopore Analytics: Sensing of Single Molecules, Chem. Soc. Rev., 2009, 38, 2360–2384 RSC.
- N. Varongchayakul, J. Song, A. Meller and M. W. Grinstaff, Single-Molecule Protein Sensing in a Nanopore: a Tutorial, Chem. Soc. Rev., 2018, 47, 8512–8524 RSC.
- O. Braha, L. Q. Gu, L. Zhou, X. F. Lu, S. Cheley and H. Bayley, Simultaneous Stochastic Sensing of Divalent Metal Ions, Nat. Biotechnol., 2000, 18, 1005–1007 CrossRef CAS PubMed.
- F. Xia, X. Zuo, R. Yang, Y. Xiao, D. Kang, A. Vallée-Bélisle, X. Gong, J. D. Yuen, B. B. Y. Hsu, A. J. Heeger and K. W. Plaxco, Colorimetric Detection of DNA, Small Molecules, Proteins, and Ions Using Unmodified Gold Nanoparticles and Conjugated Polyelectrolytes, Proc. Natl. Acad. Sci. U. S. A., 2010, 107, 10837–10841 CrossRef CAS PubMed.
- W. Zhou, R. Saran and J. Liu, Metal Sensing by DNA, Chem. Rev., 2017, 117, 8272–8325 CrossRef CAS PubMed.
- A. Ono and H. Togashi, Highly Selective Oligonucleotide-Based Sensor for Mercury(II) in Aqueous Solutions, Angew. Chem., 2004, 116, 4400–4402 CrossRef.
- H. Torigoe, Y. Miyakawa, A. Ono and T. Kozasa, Positive Cooperativity of the Specific Binding Between Hg2+ ion and T:T Mismatched Base Pairs in duplex DNA, Thermochim. Acta, 2012, 532, 28–35 CrossRef CAS.
- S. Wen, T. Zeng, L. Liu, K. Zhao, Y. Zhao, X. Liu and H. C. Wu, Highly Sensitive and Selective DNA-Based Detection of Mercury(II) with α-Hemolysin Nanopore, J. Am. Chem. Soc., 2011, 133, 18312–18317 CrossRef CAS PubMed.
- G. Wang, Q. Zhao, X. Kang and X. Guan, Probing Mercury(II)-DNA Interactions by Nanopore Stochastic Sensing, J. Phys. Chem. B, 2013, 117, 4763–4769 CrossRef CAS PubMed.
- T. Zeng, T. Li, Y. Li, L. Liu, X. Wang, Q. Liu, Y. Zhao and H. C. Wu, DNA-Based Detection of Mercury(II) Ions through Characteristic Current Signals in Nanopores with High Sensitivity and Selectivity, Nanoscale, 2014, 6, 8579–8584 RSC.
- C. Yang, L. Liu, T. Zeng, D. W. Yang, Z. Y. Yao, Y. L. Zhao and H. C. Wu, Highly Sensitive Simultaneous Detection of Lead(II) and Barium(II) with G-Quadruplex DNA in α-Hemolysin Nanopore, Anal. Chem., 2013, 85, 7302–7307 CrossRef CAS PubMed.
- R. I. Stefureac and J. S. Lee, Nanopore Analysis of the Folding of Zinc Fingers, Small, 2008, 4, 1646–1650 CrossRef CAS PubMed.
- R. I. Stefureac, C. A. Madampage, O. Andrievskaia and J. S. Lee, Nanopore Analysis of the Interaction of Metal Ions with Prion Proteins and Peptides, Biochem. Cell Biol., 2010, 88, 347–358 CrossRef CAS PubMed.
- A. Asandei, S. Iftemi, L. Mereuta, I. Schiopu and T. Luchian, Probing of Various Physiologically Relevant Metals: Amyloid-β Peptide Interactions with a Lipid Membrane-Immobilized Protein Nanopore, J. Membr. Biol., 2014, 247, 523–530 CrossRef CAS PubMed.
- G. M. Roozbahani, X. Chen, Y. Zhang, R. Xie, R. Ma, D. Li, H. Li and X. Guan, Peptide-Mediated Nanopore Detection of Uranyl Ions in Aqueous Media, ACS Sens., 2017, 2, 703–709 CrossRef CAS PubMed.
- G. M. Roozbahani, X. Chen, Y. Zhang, O. Juarez, D. Li and X. Guan, Computation-Assisted Nanopore Detection of Thorium Ions, Anal. Chem., 2018, 90, 5938–5944 CrossRef CAS PubMed.
- G. H. Wang, L. Wang, Y. J. Han, S. Zhou and X. Y. Guan, Nanopore Detection of Copper Ions Using a Polyhistidine Probe, Biosens. Bioelectron., 2014, 53, 453–458 CrossRef CAS PubMed.
- G. M. Roozbahani, X. Chen, Y. Zhang, L. Wang and X. Guan, Nanopore Detection of Metal Ions: Current Status and Future Directions, Small Methods, 2020, 4, 2000266 CrossRef CAS PubMed.
- Y. Guo, F. Jian and X. F. Kang, Nanopore Sensor for Copper Ion Detection Using a Polyamine Decorated β-Cyclodextrin as the Recognition Element, RSC Adv., 2017, 7, 15315–15320 RSC.
- K. Wei, F. Yao and X.-F. Kang, Single-Molecule Porphyrin-Metal Ion Interaction and Sensing Application, Biosens. Bioelectron., 2018, 109, 272–278 CrossRef CAS PubMed.
- A. Asandei, A. Ciuca, A. Apetrei, I. Schiopu, L. Mereuta, C. H. Seo, Y. Park and T. Luchian, Nanoscale Investigation of Generation 1 PAMAM Dendrimers Interaction with a Protein Nanopore, Sci. Rep., 2017, 7, 6167 CrossRef PubMed.
- A. Asandei, L. Mereuta and T. Luchian, The Kinetics of Ampicillin Complexation by γ-Cyclodextrins. A Single Molecule Approach, J. Phys. Chem. B, 2011, 115, 10173–10181 CrossRef CAS PubMed.
- G. Liu, L. Zhang, D. Dong, Y. Liu and J. Li, A Label-Free DNAzyme-Based Nanopore Biosensor for Highly Sensitive and Selective Lead Ion Detection, Anal. Methods, 2016, 8, 7040–7046 RSC.
- G. M. Roozbahani, Y. Zhang, X. Chen, M. H. Soflaee and X. Guan, Enzymatic Reaction-Based Nanopore Detection of Zinc Ions, Analyst, 2019, 144, 7432–7436 RSC.
- C. Plesa, S. W. Kowalczyk, R. Zinsmeester, A. Y. Grosberg, Y. Rabin and C. Dekker, Fast Translocation of Proteins through Solid State Nanopores, Nano Lett., 2013, 13, 658–663 CrossRef CAS PubMed.
- R. Capone, S. Blake, M. Rincon Restrepo, J. Yang and M. Mayer, Designing Nanosensors Based on Charged Derivatives of Gramicidin A, J. Am. Chem. Soc., 2007, 129, 9737–9745 CrossRef CAS PubMed.
- S. Blake, T. Mayer, M. Mayer and J. Yang, Monitoring Chemical Reactions by Using Ion-Channel-Forming Peptides, ChemBioChem, 2006, 7, 433–435 CrossRef CAS PubMed.
- S. Blake, R. Capone, M. Mayer and J. Yang, Chemically Reactive Derivatives of Gramicidin A for Developing Ion Channel-Based Nanoprobes, Bioconjugate Chem., 2008, 19, 1614–1624 CrossRef CAS PubMed.
- S. Futaki, M. Fukuda, M. Omote, K. Yamauchi, T. Yagami, M. Niwa and Y. Sugiura, Alamethicin-Leucine Zipper Hybrid Peptide: A Prototype for the Design of Artificial Receptors and Ion Channels, J. Am. Chem. Soc., 2001, 123, 12127–12134 CrossRef CAS PubMed.
- A. Hirano, M. Wakabayashi, Y. Matsuno and M. Sugawara, A Single-Channel Sensor Based on Gramicidin Controlled by Molecular Recognition at Bilayer Lipid Membranes Containing Receptor, Biosens. Bioelectron., 2003, 18, 973–983 CrossRef CAS PubMed.
- M. X. Macrae, S. Blake, X. Jiang, R. Capone, D. J. Estes, M. Mayer and J. Yang, A Semi-Synthetic Ion Channel Platform for Detection of Phosphatase and Protease Activity, ACS Nano, 2009, 3, 3567–3580 CrossRef CAS PubMed.
- M. Mayer, V. Semetey, I. Gitlin, J. Yang and G. M. Whitesides, Using Ion Channel-Forming Peptides to Quantify Protein-Ligand Interactions, J. Am. Chem. Soc., 2008, 130, 1453–1465 CrossRef CAS PubMed.
- Y. H. Kim, L. Hang, J. L. Cifelli, D. Sept, M. Mayer and J. Yang, Frequency-Based Analysis of Gramicidin A Nanopores Enabling Detection of Small Molecules with Picomolar Sensitivity, Anal. Chem., 2018, 90, 1635–1642 CrossRef CAS PubMed.
- L. Mereuta, A. Asandei, I. Schiopu, J. Park, Y. Park and T. Luchian, Synthetic Receptor Based on a Peptide Antibiotic-Functionalized Chimera for Hybridization-Based Polynucleotide Detection, ACS Appl. Mater. Interfaces, 2023, 15, 33159–33168 CrossRef CAS PubMed.
- D. S. Cafiso, Alamethicin – a peptide model for voltage gating and protein membrane interactions, Annu. Rev. Biophys. Biomol. Struct., 1994, 23, 141–165 CrossRef CAS PubMed.
- T. H. Evers, E. M. W. M. van Dongen, A. C. Faesen, E. W. Meijer and M. Merkx, Quantitative Understanding of the Energy Transfer between Fluorescent Proteins Connected via Flexible Peptide Linkers, Biochemistry, 2006, 45, 13183–13192 CrossRef CAS PubMed.
- H. Torigoe and F. Arakawa, Importance of Isothermal Titration Calorimetry for the Detection of the Direct Binding of Metal Ions to Mismatched Base Pairs in Duplex DNA, Dalton Trans., 2023, 52, 13089–13096 RSC.
- S. Goutelle, M. Maurin, F. Rougier, X. Barbaut, L. Bourguignon, M. Ducher and P. Maire, The Hill Equation: a Review of its Capabilities in Pharmacological Modelling, Fundam. Clin. Pharmacol., 2008, 22, 633–648 CrossRef CAS PubMed.
- D. Zhang, Y. Yin and J. Liu, Removal of Hg2+ and Methylmercury in Waters by Functionalized Multi-Walled Carbon Nanotubes: Adsorption Behavior and the Impacts of Some Environmentally Relevant Factors, Chem. Speciation Bioavailability, 2017, 29, 161–169 CrossRef CAS.
- D. Monchaud, P. Yang, L. Lacroix, M. P. Teulade-Fichou and J. L. Mergny, A Metal-Mediated Conformational Switch Controls G-Quadruplex Binding Affinity, Angew. Chem., Int. Ed., 2008, 47, 4858–4861 CrossRef CAS PubMed.
- A. Erxleben, Interactions of Copper Complexes with Nucleic Acids, Coord. Chem. Rev., 2018, 360, 92–121 CrossRef CAS.
-
S. A. Kazakov and S. M. Hecht, Nucleic Acid–Metal Ion Interactions, Encyclopedia of Inorganic Chemistry, John Wiley & Sons, Ltd, 2006 Search PubMed.
- Y. Date, A. Aota, S. Terakado, K. Sasaki, N. Matsumoto, Y. Watanabe, T. Matsue and N. Ohmura, Trace-Level Mercury Ion (Hg2+) Analysis in Aqueous Sample Based on Solid-Phase Extraction Followed by Microfluidic Immunoassay, Anal. Chem., 2013, 85, 434–440 CrossRef CAS PubMed.
- X. Zhang, N. S. Galenkamp, N. J. van der Heide, J. Moreno, G. Maglia and J. Kjems, Specific Detection of Proteins by a Nanobody-Functionalized
Nanopore Sensor, ACS Nano, 2023, 17(10), 9167–9177 CrossRef CAS PubMed.
- M. Ahmad, J.-H. Ha, L. A. Mayse, M. F. Presti, A. J. Wolfe, K. J. Moody, S. N. Loh and L. Movileanu, A generalizable nanopore sensor for highly specific protein detection at single-molecule precision, Nat. Commun., 2023, 14, 1374 CrossRef CAS PubMed.
- J. Shim and L. Q. Gu, Stochastic Sensing on a Modular Chip Containing a Single Ion Channel, Anal. Chem., 2007, 79, 2207–2213 CrossRef CAS PubMed.
- T. Mach, C. Chimerel, J. Fritz, N. Fertig, M. Winterhalter and C. Fuetterer, Miniaturized Planar Lipid Bilayer: Increased Stability, Low Electric Noise and Fast Fluid Perfusion, Anal. Bioanal. Chem., 2008, 390, 841–846 CrossRef CAS PubMed.
- M. Sondermann, M. George, N. Fertig and J. C. Behrends, High-Resolution Electrophysiology on a Chip: Transient Dynamics of Alamethicin Channel Formation, Biochim. Biophys. Acta, 2006, 1758, 545–551 CrossRef CAS PubMed.
|
This journal is © The Royal Society of Chemistry 2024 |
Click here to see how this site uses Cookies. View our privacy policy here.