DOI:
10.1039/D4OB00236A
(Review Article)
Org. Biomol. Chem., 2024,
22, 4006-4030
Natural products from the human microbiome: an emergent frontier in organic synthesis and drug discovery†
Received
14th February 2024
, Accepted 19th April 2024
First published on 19th April 2024
Abstract
Often referred to as the “second genome”, the human microbiome is at the epicenter of complex inter-habitat biochemical networks like the “gut–brain axis”, which has emerged as a significant determinant of cognition, overall health and well-being, as well as resistance to antibiotics and susceptibility to diseases. As part of a broader understanding of the nexus between the human microbiome, diseases and microbial interactions, whether encoded secondary metabolites (natural products) play crucial signalling roles has been the subject of intense scrutiny in the recent past. A major focus of these activities involves harvesting the genomic potential of the human microbiome via bioinformatics guided genome mining and culturomics. Through these efforts, an impressive number of structurally intriguing antibiotics, with enhanced chemical diversity vis-à-vis conventional antibiotics have been isolated from human commensal bacteria, thereby generating considerable interest in their total synthesis and expanding their therapeutic space for drug discovery. These developments augur well for the discovery of new drugs and antibiotics, particularly in the context of challenges posed by mycobacterial resistance and emerging new diseases. The current landscape of various synthetic campaigns and drug discovery initiatives on antibacterial natural products from the human microbiome is captured in this review with an intent to stimulate further activities in this interdisciplinary arena among the new generation.
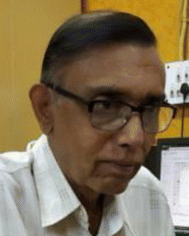 Saumitra Sengupta | Saumitra Sengupta, born in Kolkata, India (1957), was trained at the University of Florida (PhD 1987 A R Katritzky), the University of California-Santa Barbara (post-doc 1987–1989 B H Lipshutz) and the University of Waterloo (post-doc 1989–1991 V Snieckus). He joined the chemistry faculty at Jadavpur University in 1991 where his research spanned Pd-catalyzed reactions of arenediazonium salts, asymmetric synthesis and functional dendrimers. He joined Aurigene Discovery Technologies in 2005 as a Vice President, Medicinal Chemistry, leading several drug discovery programs and later moved to TCG Life Sciences in 2011 as a Distinguished Scientist until his retirement in 2015. Currently, he is a chemistry consultant. |
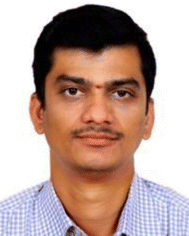 Srihari Pabbaraja | Srihari Pabbaraja obtained his PhD degree in 2004 under the guidance of Dr J. S. Yadav at the CSIR-Indian Institute of Chemical Technology from Osmania University. After completing post-doctoral studies at Rutgers, the State University of New Jersey with Prof. Spencer Knapp, he joined CSIR-IICT and started an independent career in 2008. Presently he is working as a Chief Scientist and his research interests include asymmetric total synthesis of natural products, method development for C–C bond forming reactions, medicinal chemistry, process chemistry for APIs and intermediates and continuous flow chemistry. |
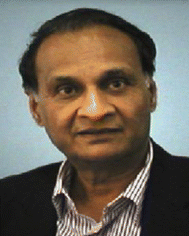 Goverdhan Mehta | Goverdhan Mehta has been associated with the Indian Institute of Technology, Kanpur, University of Hyderabad (Professor, Dean, Vice Chancellor), the Indian Institute of Science, Bangalore (Professor and Director) and held visiting positions in Belgium, UK, France, Germany, Japan, Taiwan and USA. He is a Fellow of the Indian National Science Academy (President 1999–2001), the Royal Society FRS and numerous other academies. He is a recipient of the Royal Society of Chemistry Medals and Honorary Fellowship (HonFRSC). Presently, he is a University Distinguished Professor at the University of Hyderabad with current interests in organic synthesis and in the promotion of chemistry as a sustainability science. |
1. Introduction
The human microbiota is home to trillions of variegated micro-organisms such as archaea, bacteria, fungi, viruses, etc., which have major ramifications on human health. At the beginning of this millennium, the Human Microbiome Project (HMP) was launched as a global initiative to investigate and understand the constitutional diversity of the human microbiota, the modes of microbe–host and microbe–microbe interactions and the pathophysiological role of the human microbiome in human diseases.1 The initial findings from this initiative reinforced the centrality of symbiotic interactions between individual microbes and microbe–hosts, especially the bidirectional communication between enteric and central nervous systems (often referred to as the “gut–brain axis”). This dynamic interplay profoundly influences human pathophysiological processes towards sound health and well-being.2 Disruptions in this critical symbiosis, i.e. dysbiosis of the human microbiome, have been linked to the onset of various diseases like autoimmune disorders, dermatitis, CNS disorders, obesity, diabetes, cancer, colitis, etc.3 Based on this key connection between the human microbiome and pathological states, innovative strategies in disease management via the controlled use of healthy human microbiota have emerged, especially in the areas of immuno-oncology and irritable bowel syndrome (IBS).4 In this regard, the approval of two fecal microbiota transplants, Rebyota and Vowst, in quick succession in 2022 and 2023,5 for the treatment of Clostridioides difficile infections are historical landmarks. These, in turn, have triggered considerable interest in the engineered or de novo construction of the gut microbiome for therapeutic interventions.6
The human microbiome operates via a highly complex, often personalized, mechanism for which details and understanding are scanty and work is in progress.2 However, available evidence points towards a diverse set of cellular signalling events which are specifically mediated by secondary metabolites in maintaining the symbiotic balance between the microbes and the host.7 This has led to intense activities and interest in the identification, biosynthesis and functions of secondary metabolites (natural products) from the human microbiome, especially in those derived from human commensal bacteria.8,9
1.1 Natural products through culturomics and genome mining
Although several natural products have been isolated from the human microbiome, their isolation, in general, has faced severe challenges on account of the cryptic nature of human bacteria under laboratory culture conditions.10 Hence, culture-independent genomic approaches, supported by computational algorithms for processing large volumes of genetic information from HMP reference genomes and interfacing with phenotypic data, were explored.8–17 In this regard, the three commonly pursued approaches are (a) sequence-based metagenome mining, (b) functional genomics and (c) mass spectrometry-based metabolomics analysis.
(a) In sequence-based metagenome mining, applications of algorithms viz. ClusterFinder,11 metaBGC12 or PRISM,9aetc., have revealed thousands of new biosynthetic gene clusters (BGCs) from HMP data, providing the first glimpse of the biosynthetic enormity of the human microbiome.13–15 Since the structures and bioactivities of secondary metabolites from these novel BGCs are largely unknown, attempts have been made to develop structure-prediction models, deploying the retro-biosynthetic assembly prediction and natural products chemoinformatic engines, GRAPE and GARLIC, respectively. For example, the PRISM analysis of 6009 genomes from previously cultured microbes was integrated with GRAPE and GARLIC engines (Fig. 1) which led to the identification of more than 3000 gene clusters encoding for compounds with close structural similarities to known antibiotics, thereby providing strong evidence that the human microbiome is a rich treasure of novel antibacterial natural products. It may be worthwhile to recall that soil-borne bacteria, the major source of conventional antibiotics, are rich in polyketide synthetase (PKS) & non-ribosomal peptide synthetase (NRPS) biosynthetic machineries. In contrast, the human microbiome displays a high abundance of BGCs encoding ribosomally synthesized natural products, flagging the prospect that the human microbiome may be a source of distinctly new classes of antibiotics which may be effective against the growing menace of antimicrobial resistance (AMR).16
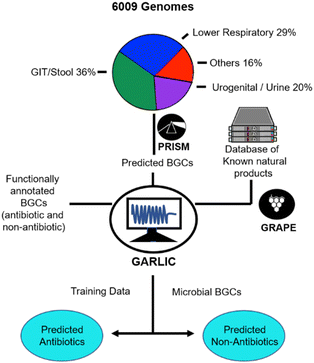 |
| Fig. 1 Chembioinformatics platform for genome mining of the human microbiome.9a | |
(b) Functional genomics have also been explored for the identification and characterization of bioactive secondary metabolites from human microbiota.8c,11 In this approach, metagenomic DNA libraries were constructed and screened for a phenotype of interest in an in vitro bioassay platform. The bioactive clone, once detected, was isolated and sequenced to identify the gene(s) responsible for the production of the bioactive metabolite. This is followed by the expression in heterologous hosts for the production of clone-specific secondary metabolites. Although, a number of novel antibiotics have been identified from the human microbiome by this method, difficulties in the cloning of large BGCs in single clones and their inefficient expression in heterologous hosts are some of the limitations in the broad usage of this approach.
(c) Mass spectrometry-based metabolomics approaches have also been used to characterize chemical metabolites from the human microbiome which, however, is limited to known primary metabolites for which searchable platforms can be generated from reference samples.17 Moreover, the transient existence and instability of some human derived secondary metabolites have complicated their isolation process.18
1.2 Human microbiome-natural products–synthesis of triumvirate
Concurrent with the activities in genome mining of the human microbiome, a sizeable number of novel antibacterial natural products have been isolated and characterized from the human gut, skin, nasal and oral cavities, either via traditional culturomics or through genome mining techniques. These new secondary metabolites have stimulated considerable interest in synthesis-enabled drug discovery, beyond formal total syntheses, in order to unravel and augment a new chemical space for SAR mapping and locating regions for structural modifications towards pharmacological gains. Several notable natural product based drugs e.g. ziconotide, trabectidin, omadacycline, eribulin, etc., have been developed through such synthesis-enabled endeavors.19,20
In light of the foregoing narrative and the evolving role of the human microbiome in health management and its expansive potentials in therapeutic interventions, the time is right to bring into focus the signaling natural products which serve as key mediators in microbial interactions. In this review, we now present an overview of the bioactive natural products from the human microbiome, with particular focus on their total synthesis and synthesis-enabled drug discovery campaigns. The topic, at the interface of chemistry and biology, would entice interest among a broad cross-disciplinary readership, ranging from natural products chemists to chemical biologists and drug discovery scientists.
1.3 Scope of the review
The review is focused on relevant commensal bacterial natural products with established structures and documented biological activities (the literature covered until December 2023). However, microbiome-derived natural products leading to pathogenic phenotypes21 such as the bacterial quorum sensing vectors,22 virulence factors or genotoxic metabolites viz. mycolactone B,23 colibactin,20g,24 tilivalline,25etc. have been reviewed recently and not included here. The narrative is divided into four sections: (i) brief description of the natural product diversity in the human microbiome, (ii) total synthesis campaigns, according to different biological classes, (iii) synthesis-enabled drug discovery initiatives and (iv) prospective natural product targets for total synthesis endeavors, which are discussed with ample schematic descriptions for the ease of reading.
2. Natural product diversity in the human microbiome
The diversity of natural products in the human microbiome can be attributed to their different habitats and biosynthetic origin. Microbial habitat is present in all parts of the human body but to date, the gut intestinal tract has been the major source of bioactive natural products, followed by the skin, vagina, mouth and nasal cavity. The chemical diversity of microbiome-derived natural products range from small molecule indole acrylic acid (1), small terpenes (2), small peptides and alkaloids (3–6), lipid based commendamide (7) to large hybrid heterocycles such as colibactin (8), see Fig. 2.
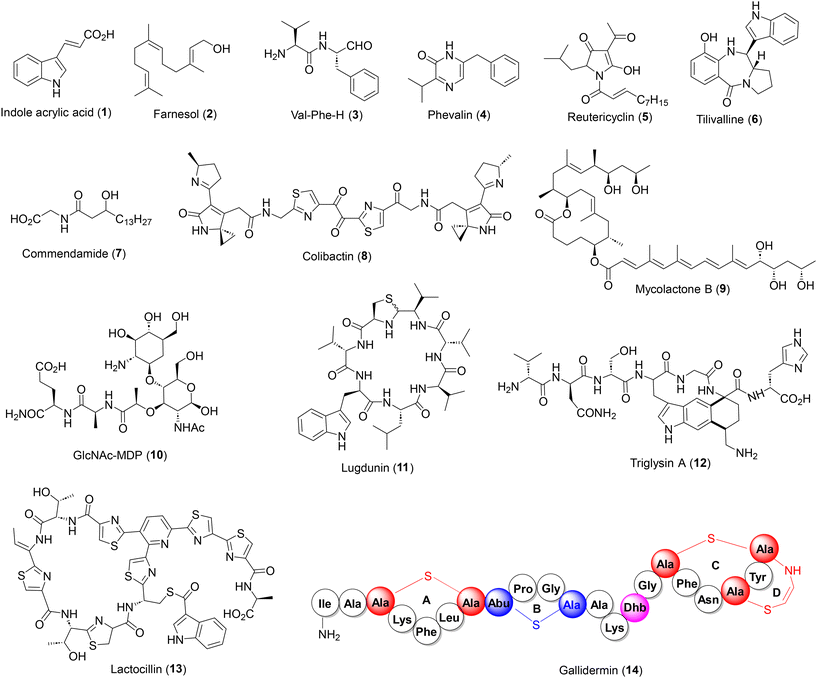 |
| Fig. 2 Diverse natural products isolated from the human microbiome. | |
More complex structures have also been identified viz. the polyketide macrolide mycolactone B (9), peptidoglycan GlcNAc-MDP (10), NRPS derived cyclopeptide lugdunin (11) and highly complex peptides such as sactipeptide triglysin A (12), thiopeptide lactocillin (13) and lantipeptide gallidermin (14). The latter three belong to ribosomally synthesized post-translationally modified peptides (RiPPs),26 which are biosynthetically and structurally distinct from conventional antibiotics found in soil-borne bacteria, further enriching the chemical diversity. In this context, it is worth noting that natural products from the human microbiome, although only a fraction of those isolated from soil-borne bacteria, display wider chemical and structural diversity owing to the presence of a broad range of biosynthetic machineries.
3. Synthesis of human microbiome-derived natural products
Human commensal bacteria are a rich source of antibiotics belonging to diverse chemical classes. These novel antibiotics, especially the structurally intriguing ribosomally synthesized and post-translationally modified peptides (RiPPs), e.g. thiopeptides,27 azole peptides,28 lantipeptides,29 sactipeptides,30etc., have drawn considerable attention from the organic synthesis community, not only due to their structural challenges but also due to their therapeutic potential as novel antibiotics against resistant bacteria. Natural products covered in this section belong to three broad biological classes: (i) bacteriocins, (ii) siderophores and (iii) natural product-like antimicrobial peptides. The discussion on individual natural products covers a brief introduction on their source, biological activities and mode of action, if known, followed by the retrosynthetic analysis and total synthesis campaigns.
3.1 Bacteriocins
Bacteriocins are secreted by bacteria against phylogenetically related strains and hence, show a narrow spectrum of antibacterial activities, less toxicity and minimum perturbation of the commensal gut bacteria.31 In the human microbiome, bacteriocins belong to the RiPP class of modified peptides which are chemically distinct from conventional soil-borne antibiotics and hence, constitute important synthetic targets due to their antibiotic potentials against resistant bacteria. Chemical syntheses of some notable bacteriocins viz. anti-listerial leucocin A, pediocin PA-1, sakacin P, curvacin A, mesentericin Y105, enterocin CRL35, etc., and the heat resistant circular antibiotic enterocin AS-48 have been discussed in detail in a recent review31b and are not discussed here.
3.1.1. Micrococcin P1.
Micrococcin P1 (15) is the first discovered member of RiPP thiopeptide antibiotics,27 whose 26-membered macrocyclic structure was established through the total synthesis by Ciufolini and co-workers (reviewed in 2010).32 Recently, micrococcin P1 was identified in the human skin isolate S. hominis S34 which showed potent activity against several S. aureus strains (MICs 0.5–1.0 μg mL−1), including a virulent MRSA strain, and accelerated the healing of severe S. aureus infected wounds.33 In view of its newly discovered anti-infective properties, two new total syntheses of micrococcin P1 have been reported in the recent literature.
The major challenge in the synthesis of micrococcin P1 lies in the construction of the highly congested 2,5,6-tri-thiazolyl pyridine core. The Walczak group approached this challenge in a linear fashion (Scheme 1) where three thiazolyl rings were successively appended to a pyridine scaffold 17via (a) a C–H activation protocol (16 + 17 → 18), (b) Mo(VI) catalysed cysteine cyclodehydration (20 → 21), and (c) the Hantzsch thiazole synthesis (23 → 25), leading to the tri-thiazolyl pyridine intermediate 25 in a good overall yield.34a The fringe amino acid fragments 26 and 28 (independently synthesized) were then introduced via HATU couplings, followed by macrocyclization with PyAOP to furnish micrococcin P1 (15). The synthetic thiopeptide was found to be highly potent against S. aureus isolates as well as a VRE strain (MICs 0.5–2 μg mL−1), confirming its reported antibacterial activities.
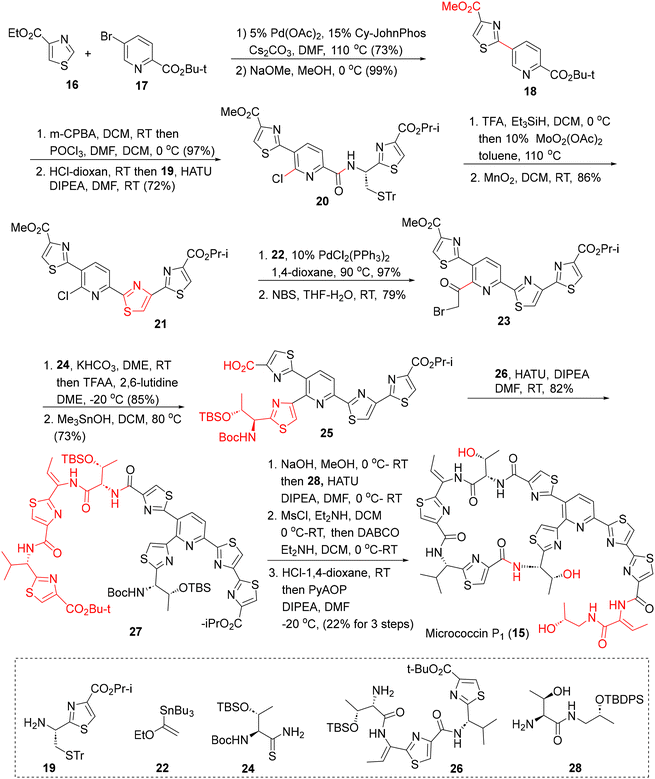 |
| Scheme 1 The Walczak synthesis of micrococcin P1 (15).34a | |
On the other hand, the Siegel synthesis of micrococcin P1 followed a modular approach for assembling the tri-thiazolyl pyridine core (Scheme 2).34b,c Commencing with 2-chloronicotinonitrile (29), the three thiazole rings were constructed, first, via oxidative cyclization with cysteineOMe·HCl (to 30), the second, via the Stille-coupling on 31 with the thiazolyl stannane 33 (assembled via the Ellman chiral sulfoximine protocol) to 34 and the third, via oxidative cyclization with thiazolyl aminothiol 35, to furnish the key tri-thiazolylpyridine core 36. Hydrolysis of the t-Bu ester followed by HATU coupling with the western fringe amino acid fragment 37 then afforded the cyclization precursor 38 (62%). Finally, ester hydrolysis, deprotection of TBS and the Ellman auxiliary, followed by macrocyclization delivered micrococcin P1 (15). The synthesized micrococcin 15 showed potent activities against S. aureus, MRSA and VRE strains (MICs 0.5–1 μg mL−1). This modular approach is amenable to multi-gram, chromatography-free operation in some key steps,34c and could be useful for analoging in SAR studies.
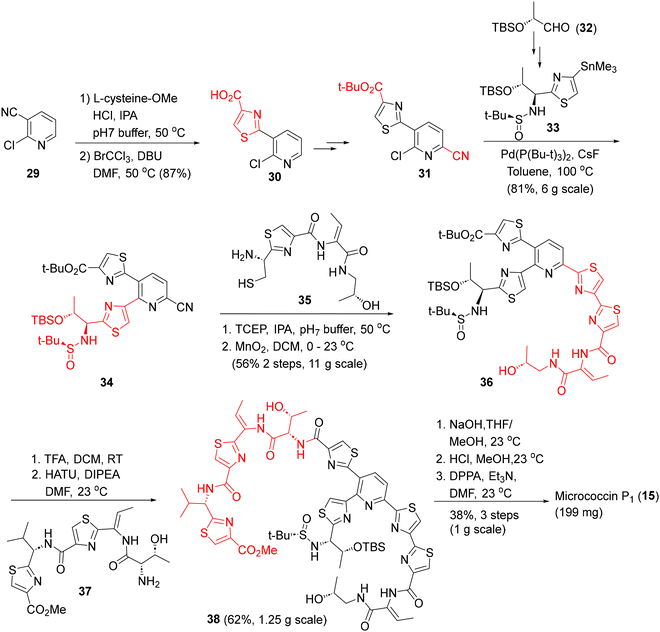 |
| Scheme 2 The Siegel synthesis of micrococcin P1 (15).34b | |
3.1.2. Microcin B17.
The ribosomally synthesized azole rich peptide microcin B17 (39), produced by the commensal gut Enterobacteriaceae, has drawn much attention as a bacterial DNA gyrase inhibitor with potent in vivo activities against pathogenic E. coli.35,36 Although 39 was synthesized by the Jung group via a linear Fmoc-based solid phase peptide synthesis (SPPS)37a and through a bio-engineered strategy in E. coli,38 its sheer size and complexity provided considerable challenge for analoging. This was overcome by Thompson et al. through a modular approach based on a thioester aminolysis-ligation strategy (Scheme 3).37b Microcin B17 39 was dissected into three retrosynthetic fragments: the C-terminal peptide 40, the central thioester fragment 41 and the N-terminal thioester unit 42 which were individually synthesized and coupled via successive Ag(I)-promoted aminolysis ligation reactions to eventuate in the natural product. This approach was exploited to synthesize a variety of analogs, whose antibacterial profiling highlighted the crucial role of the azole units and the full length natural product for cellular activity.37c
 |
| Scheme 3 Ligation-based modular synthesis of microcin B17 (39).37b | |
3.1.3. Microcin C7.
E. coli MC4100 produces a modified-antimicrobial peptide (AMP) conjugate, microcin C7 (48, Fig. 3) which exhibits potent activity against Klebsiella, Salmonella, Shigella, Yersinia strains.38,39 Microcin C7 is believed to act as a “Trojan horse” antibiotic that is recognized by transporters and imported inside targeted bacterial cells.39c The total synthesis of microcin C7 was achieved via straight-forward Fmoc-based SPPS of the heptapeptide and coupling with the modified-AMP.39b
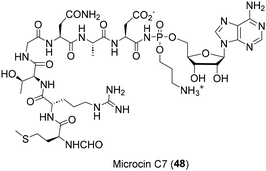 |
| Fig. 3 Structure of microcin C7 (48). | |
3.1.4. Mutanobactin A–D.
Isolated from the S. mutans40 strain UA159, mutanobactin A–D (49–52, Scheme 4) are a small group of hybrid PKS/NRPS lipopeptides with unusual structural features viz. a highly labile β-hetero-β′-keto-γ-amino amide chiral center (C-25/26) and an uncommon 1,4-thiazepan-5-one ring (mutanobactin A–C).41a–c Among them, mutanobactin D (52) was found to be a potent inhibitor of biofilm formation by the oral fungal pathogen Candida albicans (IC50 5.3 μM), drawing attention from the Carreira group towards its total synthesis.42a Retrosynthetically (Scheme 4a), the peptide segment of 52 was planned via SPPS and the sensitive β-hydroxy-β′-keto-γ-amino amide segment was envisaged as a trans-isooxazoline surrogate (53), to be revealed late in the synthesis. In the event, the azido isooxazoline acid 54, derived through asymmetric nitrile oxide cycloaddition (Scheme 4b) and the peptide fragment 60 were coupled together, followed by azide reduction, resin cleavage and macrocyclization to furnish the key retrosynthetic intermediate 53. Reductive cleavage of the isooxazoline ring followed by hydrolysis produced mutanobactin D (52) which showed a 25R,26R configuration, thereby establishing the absolute configurations of these two labile chiral centers. The synthesized mutanobactin D 52 showed potent anti-biofilm activities against three C. albicans strains (table, Scheme 4) and significantly reduced filament growth in ATCC 90028 and 101 strains.42a
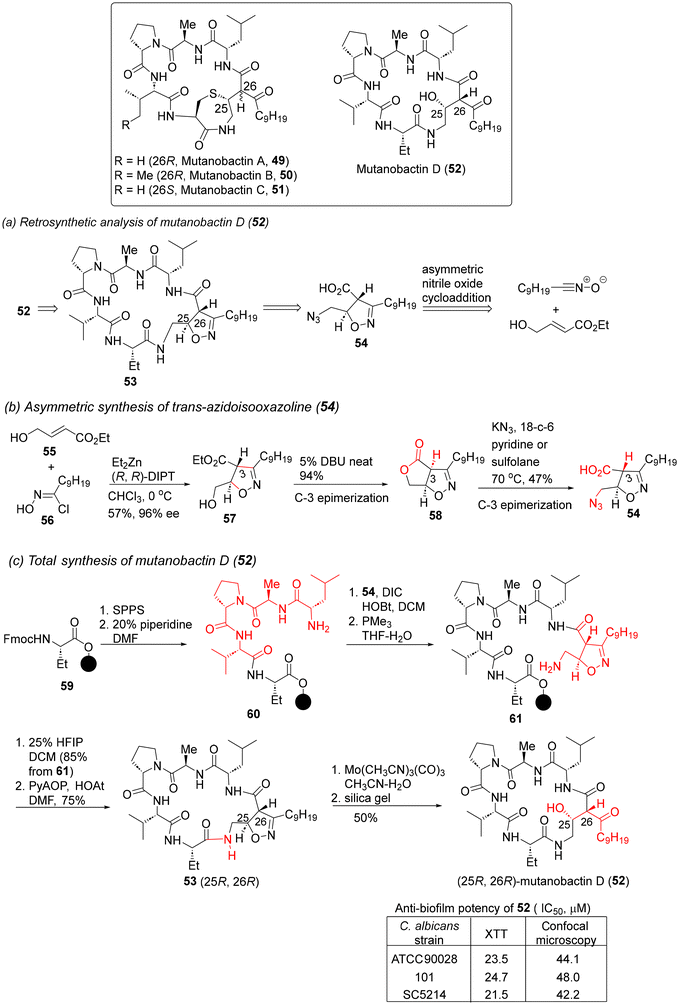 |
| Scheme 4 Retrosynthesis and total synthesis of mutanobactin D (52).42a | |
The Carreira group also reported the total synthesis of the thiazepanone lipopeptides mutanobactin A and B (49 and 50) (Scheme 5),42b despite their weak anti-biofilm properties.41a,b Pursuant to the retrosynthetic analysis shown in Scheme 5a, the tetrapeptide fragments were assembled via Fmoc-based SPPS on 65, leading to the peptide amines 66a and b. HATU coupling with the protected β-keto acid 67 then furnished the key on-resin retrosynthetic intermediates 63a and b. Resin cleavage with aqueous TFA led to the in situ formation of thioacetals 62a and b which spontaneously cyclized under acidic conditions to produce the natural products 49 and 50 in good overall yields. The strategy was also extended to the synthesis of mutanobactin D (52) (Scheme 5c), thereby demonstrating a unified synthetic approach for three mutanobactin siblings.42b
 |
| Scheme 5 Retrosynthesis and total synthesis of mutanobactin A, B, and D (49, 50, and 52).42b | |
3.1.5. Lugdunin.
Lugdunin (11, Fig. 2) is a novel thiazolidine cyclopeptide with an unusual disposition of alternating D- and L-amino acids which was isolated from Staphylococcus lugdunensis IVK28 and exhibited broad antibacterial activities on multiple S. aureus strains, including MRSA, VRE and B. subtilis (MICs 1.5–4 μg mL−1) with a high barrier to resistance.43 In order to build a SAR map of lugdunin, Grond and co-workers carried out a diverted solid-phase total synthesis of lugdunin (11), along with 60 analogs (Schemes 6 and 7).44 In the first-generation synthesis (Scheme 6), macrocyclization was achieved from the linear peptide aldehyde 71 (obtained from the resin bound peptide 69) via macrocyclic imine 72 formation45 followed by a facile intramolecular thiol addition to furnish lugdunin (11).44a However, the strongly acidic conditions used in the cyclization step also led to the epimerization of the Val(7) chiral center, resulting in 4 epimeric products. Later, in the second generation synthesis, the authors installed a configurationally stable thiazolidine amino acid 73, early in peptide build-up (cf.75), followed by macrocyclization via Val(5)–Val(6) amide bond formation which furnished epimerically pure lugdunin (Scheme 7).44b This latter strategy was then applied to a diverted total synthesis of nearly 60 analogs which were screened against MRSA USA300 LAC. Consequently, a SAR map of lugdunin chemical space was developed (see box, Scheme 7) which could be useful for drug discovery efforts.44a,b
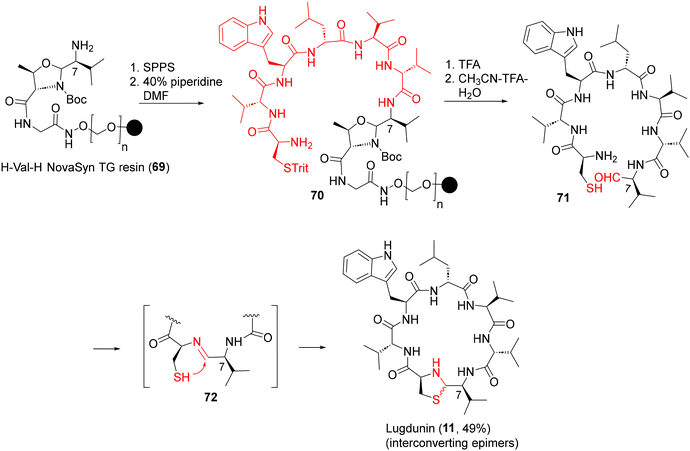 |
| Scheme 6 First-generation SPPS of lugdunin (11) via thiazolidine macrocyclization.44a | |
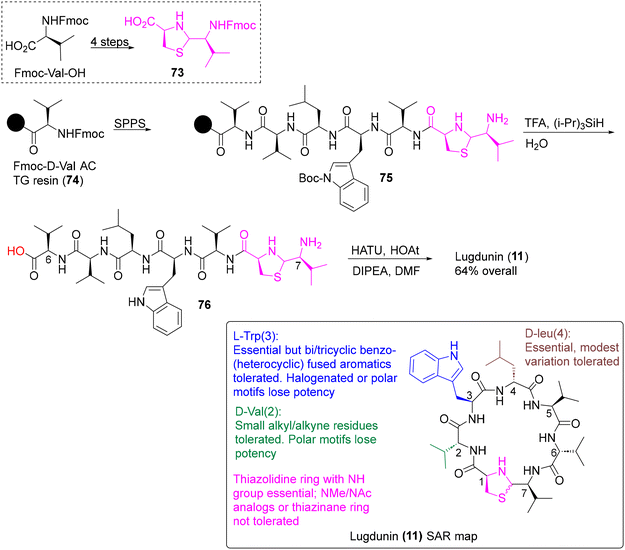 |
| Scheme 7 Second-generation SPPS and SAR map (box) of lugdunin (11) using the thiazolidine building block 73.44b | |
3.1.6 Lantibiotics.
A biologically important sub-group of RiPP bacteriocins is the lantibiotics,29 typically consisting of sulfur-bridged lantionine (Lan) and β-methyllantionine (MeLan) rings that are post-translationally derived via the intramolecular 1,4-addition of cysteines to dehydroalanine (Dha) or dehydrobutyrine (Dhb) residues (Fig. 4).
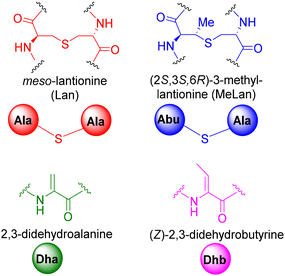 |
| Fig. 4 Lantionine (Lan), β-methyllantionine (MeLan), dehydroalanine (Dha) and dehydrobutyrine (Dhb) notations. | |
Lantionine rings are the key pharmacophores which, while imparting turn-structures to the lanti-peptides, also act as target binding domains or pore-forming motifs for antibiotic action. Examples of antibacterial lantibiotics isolated from the human microbiota are epilancin 15× (77), lacticin 481 (78) and the two-component antibiotics lacticin 3147 (79a,b) and cytolysin (80a,b) (Fig. 5).
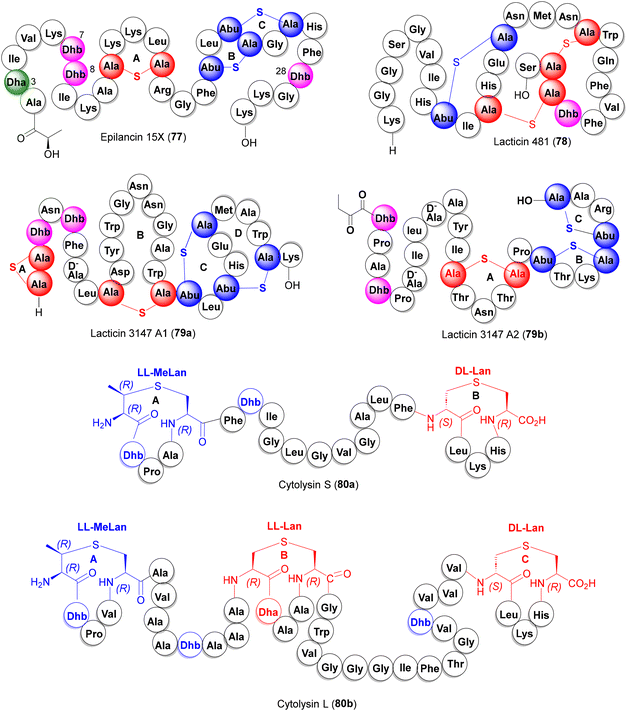 |
| Fig. 5 Lantibiotics isolated from the human microbiome. | |
3.1.6.1. Epilancin 15×.
Isolated from human wound infection and skin colonies, the clinical strain S. epidermidis 15 × 154 produces the tricyclic lantibiotic epilancin 15× (77) which displays strong antibacterial activities against Gram +ve bacteria, including MRSA and VRE strains (MICs 0.1–1 μg mL−1).46 In addition to three Lan and MeLan rings A–C, 77 also features three N-terminal unsaturated residues (Dha3, Dhb7, and Dhb8). Although the total synthesis of epilancin 15× has not yet been described, Knerr and van der Donk reported the synthesis of a few N-terminal analogs 90–92 where the unsaturated Dha3, Dhb7, and Dhb8 residues were replaced with their saturated counterparts (Scheme 8).47 In this synthesis, the orthogonally protected synthons 81–83
48 were deployed as precursors to the Lan and MeLan thioether rings and strategically inserted in the growing peptide chain via SPPS. The thioether rings were then constructed via a three-step protocol: orthogonal deprotection of the lantionine building block, Fmoc removal and ring cyclization using PyAOP, as illustrated in the formation of 86 (ring C), 87 (ring B) and 89 (ring A). The latter intermediate was then used as a scaffold for the synthesis of the desired N-terminal analogs 90–92via the attachment of the remaining N-terminal amino acids. MIC determination against S. carnosus TM300 showed that the full-length analogs 91 and 92 (but not the truncated variant 90) were almost equipotent with the natural products.
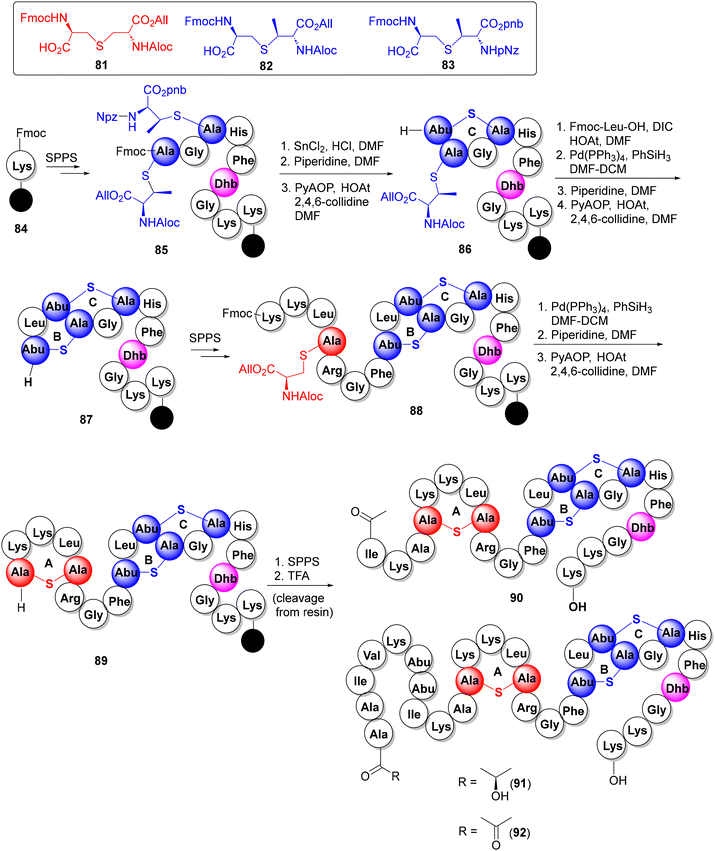 |
| Scheme 8 SPPS synthesis of epilancin 15× N-terminal analogs 90–92.47 | |
3.1.6.2. Lacticin 481.
Lacticin 481 (78, Fig. 5) is a 27-amino acid tricyclic lantibiotic produced by the lactic acid bacteria Lactococcus lactis subspecies lactis, featuring two DL-Lan thioether bridges, a large DL-MeLan bridge and an unsaturated Dhb unit.49 The van der Donk group reported a solid-phase synthesis of 78 and its Lan/MeLan stereoisomers, following the same strategy as described for epilancin 15× (cf.Scheme 8) which revealed the critical dependence of the antibacterial potency on the stereochemistry of the Lan/MeLan bridges.50a Syntheses of a few analogs of 78 have also been reported via mutasynthesis.50b–d
3.1.6.3. Lacticin 3147 (A1 + A2).
Produced by the Lactococcus lactis subspecies lactis DPC3147, lacticin 3147 is a two-component lantibiotic, composed of interlocked thioether bridged peptide A1 (79a) and the linear lanti-peptide A2 (79b) (Fig. 5), exhibiting a broad-spectrum activity against Gram +ve bacteria including MRSA, VRE and mycobacteria.51 Mechanistically, peptides A1 and A2 form a ternary complex with lipid-II on the surface of the cell membrane which inhibits cell wall synthesis, causing pore formation and cell rupture.51e The Vederas group has reported the solid phase synthesis of both components of lacticin 3147, again, deploying the orthogonally protected synthons 81–83 as the thiother ring precursors.52a For peptide A1 79a (Scheme 9), the interlocked MeLan-bridged rings C and D provided a stiff synthetic challenge and were eventually constructed from a precursor peptide 96via successive orthogonal deprotection of MeLan building blocks (via Pd-catalyzed allyl deprotection or SnCl2 removal of nitrobenzyl groups), each followed by Fmoc removal and PyBOP cyclization to produce the desired C/D-interlocked bicyclic intermediate 98. The remaining rings B and A were then constructed on 98 to furnish peptide A1 (79a) in a remarkable 1.4% overall yield from 84 in more than 50 steps.
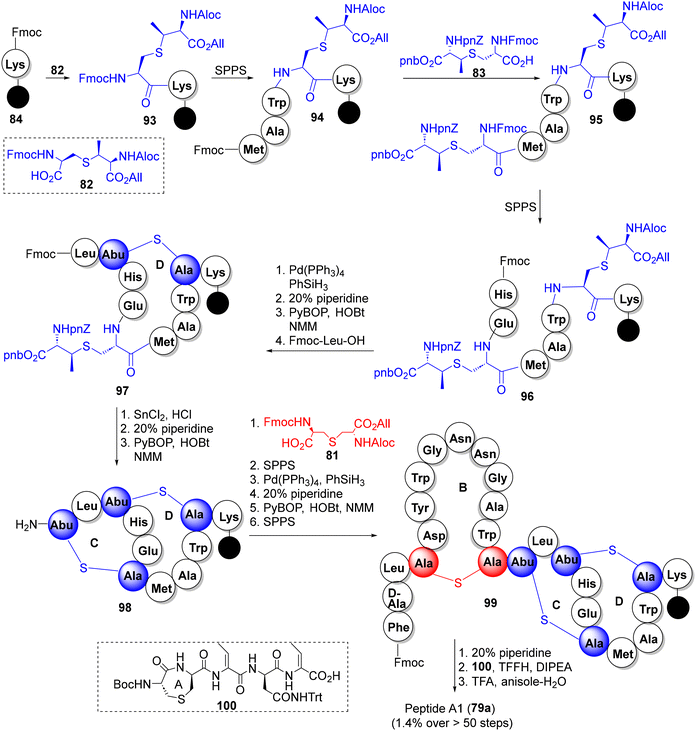 |
| Scheme 9 The Vederas synthesis of lacticin 3147 constituent peptide A1 (79a).52a | |
For the synthesis of peptide A2 (79b), the synthesis commenced with the 2-chlorotrityl polystyrene resin 101, loaded with the MeLan synthon 82. Following a similar strategy as above i.e. incorporation of Lan and MeLan synthons 81 and 82 at appropriate junctures during peptide build-up, the three thioether rings were constructed, successively (C to B to A), via Pd-catalyzed allyl deprotection, Fmoc removal and PyBOP cyclization, furnishing lacticin 3147 peptide A2 (79b) in 1% overall yield (Scheme 10).52a The synthetic peptides A1 and A2 showed excellent synergy against the Lactococcus lactis subspecies cremoris HP, indistinguishable from natural lacticin 3147. The Vederas group also prepared oxa- and desmethyl-Lan analogues of peptide A2 which, however, lost antibacterial activities and synergy (with A1), pointing to the indispensable role of natural MeLan units in A1 and A2 for antibiotic activities.52b,c
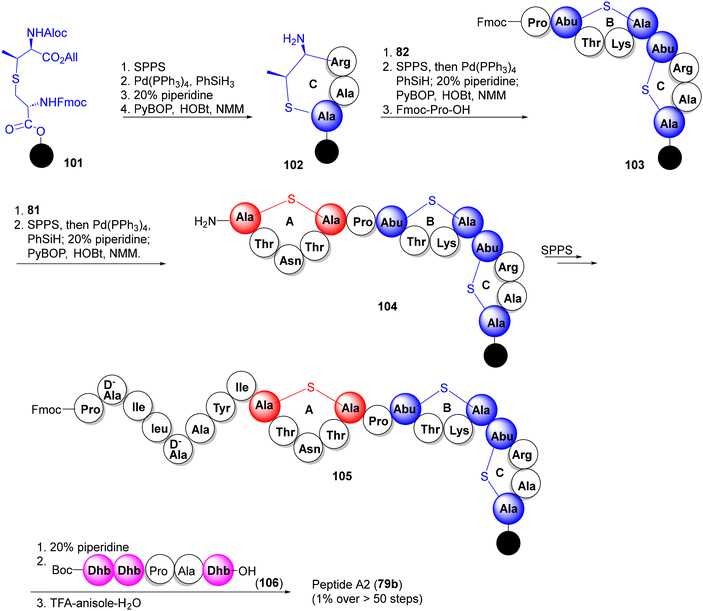 |
| Scheme 10 The Vederas synthesis of lacticin 3147 constituent peptide A2 (65b).52a | |
3.1.6.4. Cytolysin.
Expressed by E. faecalis, cytolysin is a 2-component pore-forming lantibiotic, composed of two lantipeptides cytolysin S (80a) and cytolysin L (80b) (Fig. 5) which exhibits synergistic antimicrobial activity against Gram +ve bacteria.53 Interestingly, some lantionine rings in cytolysin peptides show an unusual LL-stereochemistry which, via diverted total synthesis of all possible Lan/MeLan stereoisomeric peptides, were shown to be crucial for their antibiotic activities.54
3.2 Siderophores
The lack of antibiotic efficiency against Gram −ve pathogens, primarily attributed to the latters’ impervious outer membrane, has forced researchers to pursue non-conventional approaches against Gram −ve pathogens. One such approach makes use of small molecule chelators called siderophores which are secreted under stress by all bacteria for iron transport across the cell membrane for their survival.55 This unique feature of siderophores in penetrating the Gram −ve bacterial membrane has inspired a non-conventional antibacterial strategy where antibiotic-siderophore conjugates are deployed for cell penetration and the subsequent release of antibiotic payloads inside Gram −ve bacteria (“Trojan horse” approach).55b,e
A variety of siderophores, belonging to hydroximate and/or catechol classes, have been isolated from human Gram −ve pathogens.56 Notable examples are enterobactin (107, from E. coli and S. typhimurium),57 acinetobactin (108, from A. baumannii),58 fimsbactin A (109, from A. baumannii),59 pseudopalin (110, from P. aeruginosa),60 the chromophoric chelator pyoverdin D (111, from P. aeruginosa),61etc., (Fig. 6) which have been the focus of extensive investigations as antibiotic carriers and diagnostics. The chemistry and biology of these siderophores, including their total synthesis, have already been captured in recent accounts, as cited above and hence, not elaborated in this review.
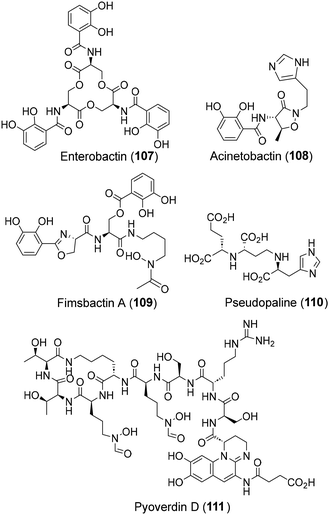 |
| Fig. 6 Siderophores from human associated Gram −ve bacteria. | |
3.3 Genome-mined natural product-like AMPs
Since only a fraction of the bacterial biosynthetic diversity is accessible through the laboratory broth culture, antibacterial screening of bioinformatics predicted peptide libraries (synthesized via SPPS) has been effectively leveraged to discover natural product-like AMPs. In this regard, the Brady group applied the “synthetic-bioinformatic natural products (syn-BNPs)” platform62–64 for the sequence analysis of top 25 NRPS-BGCs from the human microbiome database to predict the encoded natural product-like AMP structures. Based on such inputs, peptide libraries were synthesized via routine SPPS and their antibacterial screening leading to the discovery of a new antibiotic humimycin A (112, Fig. 7) exhibiting potent activity against S. aureus, including MRSA and S. pneumoniae.62 In addition, humimycin A and its analog 113 also potentiated carbenicillin and dicloxacillin activities in MRSA USA300, COL and VRE strains without signs of bacterial resistance (Fig. 7).63
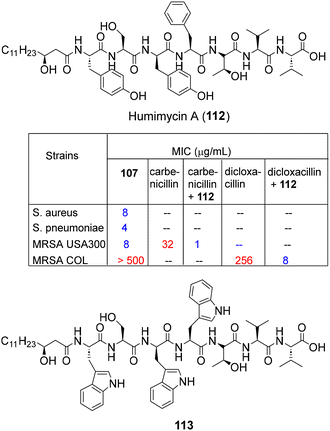 |
| Fig. 7 Syn-BNP antibiotic potentiators humimycin A (112) and 113. | |
In another culture independent approach, Chen and co-workers combined multiple natural language processing neural network models, including LSTM, Attention and BERT, for the autonomous learning of AMP sequences to construct AMP prediction models from large-scale human microbiome data.65a By this exercise, 2349 sequences were identified as candidate AMPs, out of which, 216 peptides were chemically synthesized via routine SPPS and found to be active against Gram −ve pathogens. In a remarkable revelation, the peptide AMP1043 (KQKTLKKVWKLSEKVLIFASAFAKKAGAAEA-TLVL) was found to be highly active against the ESKAPE pathogens E. coli (MIC 2 uM), K. pneumoniae (MIC 2.5 uM) and A. baumannii (MIC 10 uM) as well as MDR resistant strains (MICs < 8 μM). The above AI driven strategies highlight the combination of chemical synthesis and bioinformatics as a versatile platform in deciphering large complex metagenomic information from human microbiota for the accelerated discovery of AMPs.65b,c
4. Drug discovery initiatives
Spurred by the approval of Rebyota and Vowst as live fecal microbiota drugs in 2022–23,5 dozens of clinical trials are currently underway with live fecal microbiota or assorted consortia for the treatment of gut-related syndromes.66,67 However, treatment of more severe infections caused by MRSA, VRE and Gram −ve pathogens still relies on targeted therapies using small molecule drugs. In this context, a few natural products from the human microbiome are emerging as promising leads for clinical applications,68 and are highlighted below.
4.1. Nisin A
Nisin A (114, Fig. 8) and its eight siblings are heat resistant pentacyclic lantibiotics produced by lactic acid bacteria (Lactococcus sp.),69 among which, nisin A and Z have been approved as food preservatives with acceptable safety profiles in humans. Furthermore, in view of the clinical success of nisin extracts as effective probiotics70 and potent activities against MRSA, S. pneumoniae, Enterococci, H. pylori and C. difficile, nisin A is emerging as a promising lead for antibiotic development. Improvements in sub-optimal pharmacokinetics of nisin A through bio-engineered analoging have met with limited success. In this regard, the recently reported SPPS approach to nisin A71 holds much promise in augmenting diversity creation through innovative chemical interventions.26h It is contextual to recall that a truncated variant of nisin A has been recently discovered with similar antibacterial profile and identified as cesin A (115, Fig. 8),69d which appears to be a promising target for global analoging towards pharmacological optimization.
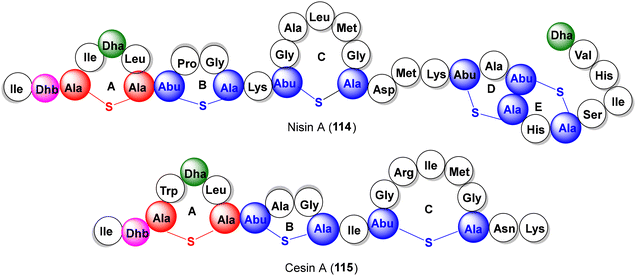 |
| Fig. 8 Nisin A (114) and its truncated analog cesin A (115). | |
4.2. Epidermicin NI01
Produced by the skin isolate Staphylococcus epidermidis 224, epidermicin NI01 is an unmodified 51-residue bacteriocin (MAAFMKLIQFLATKGGKYVSLAWKHK-GTILKWINAGQSFEWITKQKKLWA) which exhibited potent activity against a panel of Gram +ve bacteria, including MRSA, VRE and biofilm-forming S. epidermidis strains (MICs 1–4 μg mL−1).72a In a pre-clinical study conducted at Amprologix, a single dose of epidermicin NI01 demonstrated resistance-free properties and six times more efficacy in reducing in vivo nasal MRSA burden than the “gold standard” antibiotic mupirocin.72b A 14-day safety and tolerability study of epidermicin NI01 formulation returned satisfactory end points with no adverse side effects. Based on these findings, the inventors have planned IND filing and clinical trials of epidermicin NI01 for nasal MRSA de-colonization in 2024.72c
4.3. Mutacin 1140 (mutacin III)
Produced by the oral bacteria Streptococcus mutans JH100, the aminovinyl cysteine lantibiotic mutacin 1140 (mutacin III, 116, Fig. 9) has been the focus of several investigations due to its antibiotic activity (against MRSA, VRE, C. difficile), low susceptibility to resistance and a novel mode of action (lipid-II abduction).73,74 In an effort to develop mutacin 1140 based antibiotics, Oragenics screened nearly 700 analogs of mutacin 1140, locating key point mutations viz. Lys2Ala, Arg13Ala and Phe1Ile as the chemical handles for modulating the pharmacokinetic attributes.75 Recently, as a pre-clinical proof-of-concept, positive results from a detailed pharmacological, toxicological and dose-range assessment of an advanced variant OG716 (117, Phe1Ile, and Arg13Asn) have been reported for treatment against C. difficile infections.76
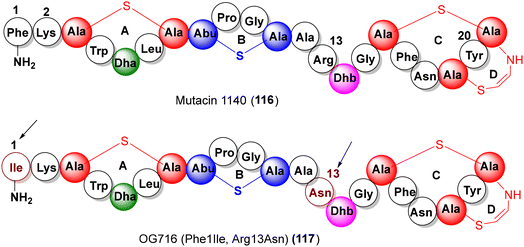 |
| Fig. 9 Structures of mutacin 1140 (116) and the mutated analog OG716 (117). | |
4.4. Linaclotide, plecanatide, dolcanatide
The E. coli derived heat-stable enterotoxin STa, together with the endogenous peptide hormones guanylin and uroguanylin, are potent guanylate cyclase C agonists, which regulate gastrointestinal fluid transits. Hence, it was reasoned that the synthetic analogs of STa, guanylin and uroguanylin could be effective therapeutic agents against irritable bowel syndrome (IBS), chronic idiopathic constipation (CIC), etc.,77 leading to the discovery of three IBS drugs – linaclotide (118),78 plecanatide (122)79 and dolcanatide (123)80 (Fig. 10). Considering its unique structural features (3 disulfide bridges) and clinical utility, linaclotide has received repeated attention from practitioners of total synthesis.81 The most effective among them is by Brik and co-workers, deploying orthogonal cysteine-protecting groups (SNBzl and SAcm), for highly regioselective successive disulphide cross-linkings (red, blue and green) on a SPPS derived linear precursor 119 (Scheme 11).81c Two solid-phase total syntheses of plecanatide have also been reported in the recent literature.79c,d
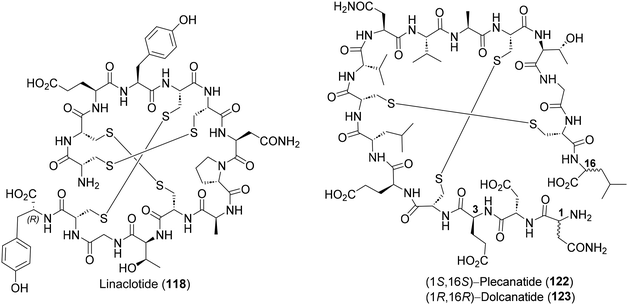 |
| Fig. 10 Linaclotide (118), plecanatide (122) and dolcanatide (123). | |
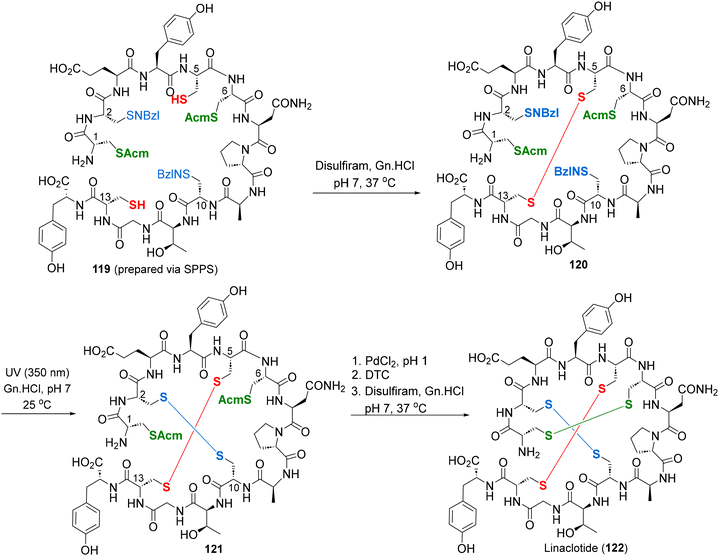 |
| Scheme 11 One-pot synthesis of linaclotide (104) via selective disulphide cross-linking | |
5. Prospective natural product targets for the total synthesis
From the total synthesis and utilitarian perspective, a few potentially attractive natural product targets could be identified for future endeavors (124–134, Fig. 11).82–91 Among them, the highly convoluted sactipeptides streptosactin (128),84 tryglysin A and B (129 and 130)85 and ruminococcin C1 (131),86–88 are of particular interest due to their strong antibiotic action and favourable clinical attributes (non-toxic towards eukaryotic cells and little tendency towards developing resistance). In addition, lasso peptides microcin J25 (134), acinetodin, klebsidin, etc., with potent antibiotic activities against Gram −ve pathogens, are highly challenging synthetic targets due to their unique catenane-like structures (lariat protoknot) where the C-terminal ends are threaded (dotted line) through a peptide macrocycle.92,93
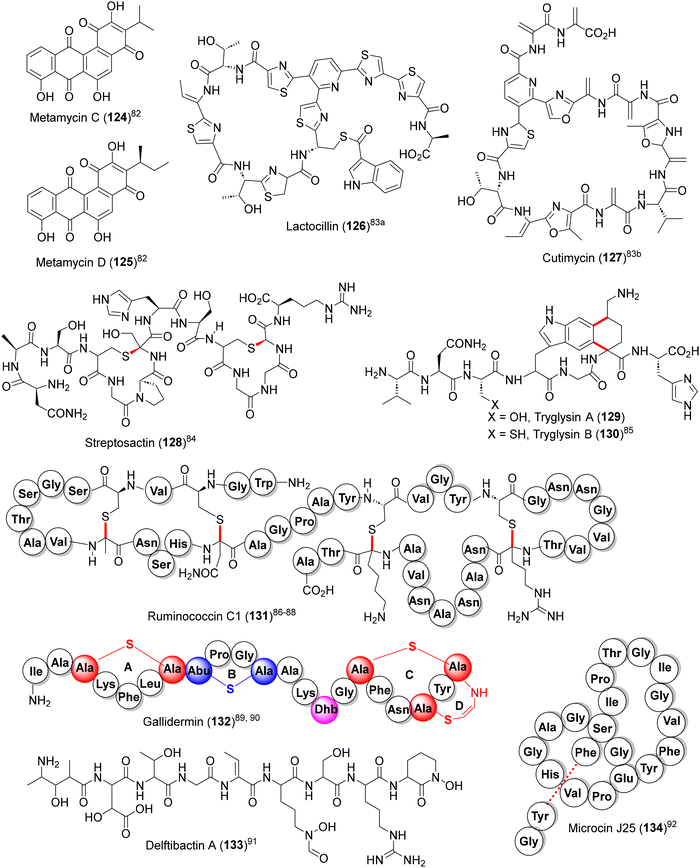 |
| Fig. 11 Natural products from the human microbiome as prospective targets for total synthesis | |
6. Summary and outlook
The evolving role of the human microbiome as a key determinant in human health and the concurrent discovery of encoded secondary metabolites as potent antibiotics have revealed the enormous potential of the human microbiome as a rich new source of antibacterial natural products. In this review, we have captured the emergent landscape of natural products derived from human commensal bacteria and highlighted their salient features. These new natural products viz. metamycin C–D, mutanobactin A–D, lugdunin and the RiPP variants epilancin 15×, ruminococcin C1, etc., have evoked considerable interest in the organic synthesis community due to their potent antibacterial properties and marked distinguishing structural features vis-à-vis conventional antibiotics. These features flagged the prospect of exploring their potential utility against MDR bacteria, pathogenic viruses, fungi and parasites (AMR, in general) as well as gut-related syndromes. In view of their intriguing structures, innovative synthetic strategies like modular building block approaches, deployment of enantiopure orthogonal synthons, novel heterocyclic macrocyclizations, among others, were leveraged as key enabling tactics for total synthesis and diverted organic synthesis to explore new bioactive chemical spaces. The prospects of antibiotic drug discovery through these approaches have been highlighted through a few case studies which include the recently approved IBS drugs linaclotide and plecanatide as archetypal examples.
Looking ahead, it is important to sustain the momentum and the promising advances enumerated above, with major thrust directed towards augmenting laboratory isolation and structural characterization activities through synergistic applications of genome mining techniques and innovations in culturomics.10 At the same time, new imaginative synthetic designs, including merged bioengineering–chemical synthesis approaches,94 need to be explored to tackle the structural and scale-up challenges to reveal new chemical spaces. In addition to the above, efforts directed towards newer areas of microbiome research viz. exploration of probiotic therapy, application of gut microbes for selective biotransformations95 and insights into the chemistry of host–microbe interaction96 would be welcome advances in order to amplify the ambit of the human microbiome research landscape.
Lastly, it is heartening to note that many countries, particularly those with a living traditional knowledge base and cultural moorings,97 have also embarked on initiatives to unveil the mysteries of the diet–season–microbiome triad on human health and harness its role for the overall well-being of people.
Author contributions
All authors contributed equally to the manuscript.
Conflicts of interest
There are no conflicts to declare.
Acknowledgements
GM thanks Dr Reddy's Laboratories for research support at the School of Chemistry, University of Hyderabad. SS is a Visiting Scholar at the School of Chemistry, University of Hyderabad and the CSIR-Indian Institute of Chemical Technology, Hyderabad. SP thanks Director, CSIR-IICT for research support as part of the Indo-French “Joint Laboratory for Natural Products and Synthesis towards Affordable Health (NPSAH)”. CSIR-IICT communication no. IICT/Pubs./2024/051.
References
-
(a) P. J. Turnbaugh, R. E. Ley, M. Hamady, C. M. Fraser-Liggett, R. Knight and J. I. Gordon, Nature, 2007, 449, 804–810 CrossRef CAS PubMed;
(b) The Human Microbiome Project Consortium, Nature, 2012, 486, 207–214 CrossRef PubMed;
(c) The Human Microbiome Project Consortium, Nature, 2012, 486, 215–221 CrossRef PubMed;
(d) G. M. Weinstock, Nature, 2012, 489, 250–256 CrossRef CAS PubMed;
(e) N. Koppel and E. P. Balskus, Cell Chem. Biol., 2016, 23, 18–30 CrossRef CAS PubMed;
(f) J. A. Gilbert, M. J. Blaser, J. G. Caporaso, J. K. Jansson, S. V. Lynch and R. Knight, Nat. Med., 2018, 24, 392–400 CrossRef CAS PubMed;
(g) The Integrative HMP (iHMP) Research Network Consortium, Nature, 2019, 569, 641–648 CrossRef PubMed;
(h) E. P. Balskus, Biochemistry, 2022, 61, 2777–2778 CrossRef CAS PubMed.
-
(a) A. L. Kau, P. P. Ahern, N. W. Griffin, A. L. Goodman and J. I. Gordon, Nature, 2011, 474, 327–336 CrossRef CAS PubMed;
(b) P. A. Smith, Nature, 2015, 526, 312–314 CrossRef CAS PubMed;
(c) M. G. Rooks and W. S. Garrett, Nat. Rev. Immunol., 2016, 16, 341–352 CrossRef CAS PubMed;
(d) Y. Lai, R. Dhingra, Z. Zhang, L. M. Ball, M. J. Zylka and K. Lu, Biochemistry, 2022, 61, 2806–2821 CrossRef CAS PubMed;
(e) N. Aggarwal, S. Kitano, G. R. Y. Puah, S. Kittlemann, I. Y. Hwang and M. W. Chang, Chem. Rev., 2023, 123, 31–72 CrossRef CAS PubMed.
-
(a) S. V. Lynch and O. Pedersen, N. Engl. J. Med., 2016, 375, 2369–2379 CrossRef CAS PubMed;
(b) P. Vernocchi, F. Del Chierico and L. Putignani, Front. Microbiol., 2016, 7, 1144 Search PubMed;
(c) J. R. Marchesi, D. H. Adams, F. Fava, G. D. A. Hermes, G. M. Hirschfield, G. Hold, M. N. Quraishi, J. Kinross, H. Smidt, K. M. Tuohy, L. V. Thomas, E. G. Zoetendal and A. Hart, Gut, 2016, 65, 330–339 CrossRef PubMed;
(d) T. Nakatsuji, T. H. Chen, S. Narala, K. A. Chun, A. M. Two, T. Yun, F. Shafiq, P. F. Kotol, A. Bouslimani, A. V. Melnik, H. Latif, J.-N. Kim, A. Lockhart, K. Artis, G. David, P. Taylor, J. Streib, P. C. Dorrestein, A. Grier, S. R. Gill, K. Zengler, T. R. Hata, D. Y. M. Leung and R. L. Gallo, Sci. Transl. Med., 2017, 9, eaah4680 CrossRef PubMed;
(e) W. van Treuren and D. Dodd, Annu. Rev. Pathol.: Mech. Dis., 2020, 15, 345–369 CrossRef CAS PubMed;
(f) S. N. Chaudhari, M. D. McCurry and A. S. Devlin, Nat. Chem. Biol., 2021, 17, 1046–1056 CrossRef CAS PubMed;
(g) K. Hou, Z.-X. Wu, X.-Y. Chen, J.-Q. Wang, D. Zhang, C. Xiao, D. Zhu, J. B. Koya, L. Wei, J. Li and Z.-S. Chen, Signal Transduction Targeted Ther., 2022, 7, 135 CrossRef PubMed.
-
(a) S. Turjeman and O. Koren, Microb. Biotechnol., 2022, 15, 129–134 CrossRef PubMed;
(b) Y. Zhang, L. Zhou, J. Xia, C. Dong and X. Luo, Front. Mol. Biosci., 2022, 8, 703585 CrossRef PubMed;
(c) N. Aggarwal, S. Kitano, G. R. Y. Puah, S. Kittelmann, I. Y. Hwang and M. W. Chang, Chem. Rev., 2023, 123, 31–72 CrossRef CAS PubMed.
-
(a) A. Mullard, Nat. Rev. Drug Discovery, 2022, 21, 786–787 Search PubMed;
(b) A. Mullard, Nat. Rev. Drug Discovery, 2023, 22, 436 Search PubMed.
-
(a) R. L. Clark, B. M. Connors, D. M. Stevenson, S. E. Hromada, J. J. Hamilton, D. Amador-Noguez and O. S. Venturelli, Nat. Commun., 2021, 12, 3254 CrossRef CAS PubMed;
(b) A. G. Cheng, P.-Y. Ho, A. Aranda-Díaz, S. Jain, F. B. Yu, X. Meng, M. Wang, M. Iakiviak, K. Nagashima, A. Zhao, P. Murugkar, A. Patil, K. Atabakhsh, A. Weakley, J. Yan, A. R. Brumbaugh, S. Higginbottom, A. Dimas, A. L. Shiver, A. Deutschbauer, N. Neff, J. L. Sonnenburg, K. C. Huang and M. A. Fischbach, Cell, 2022, 185, 3617–3636 CrossRef CAS PubMed.
-
(a) N. Dufour and R. P. Rao, FEMS Microbiol. Lett., 2011, 314, 10–17 CrossRef CAS PubMed;
(b) J. K. Nicholson, E. Holmes, J. Kinross, R. Burcelin, G. Gibson, W. Jia and S. Pettersson, Science, 2012, 336, 1262–1267 CrossRef CAS PubMed;
(c) G. Sharon, N. Garg, J. Debelius, R. Knight, P. C. Dorrestein and S. K. Mazmanian, Cell Metab., 2014, 20, 719–730 CrossRef CAS PubMed;
(d) M. Fischbach, Cell, 2018, 174, 785–790 CrossRef CAS PubMed.
-
(a) M. S. Donia and M. A. Fischbach, Science, 2015, 349, 1254766 CrossRef PubMed;
(b) M. R. Wilson, L. Zha and E. P. Balskus, J. Biol. Chem., 2017, 292, 8546–8552 CrossRef CAS PubMed;
(c) A. Milshteyn, D. A. Colosimo and S. F. Brady, Cell Host Microbe, 2018, 23, 725–736 CrossRef CAS PubMed;
(d) L. Wang, V. Ravichandran, Y. Yin, J. Yin and Y. Zhang, Trends Biotechnol., 2019, 37, 492–504 CrossRef CAS PubMed;
(e) J. E. Silpe and E. P. Balskus, ACS Cent. Sci., 2021, 7, 20–29 CrossRef CAS PubMed.
-
(a) W. K. Mousa, B. Athar, N. J. Merwin and N. A. Magarvey, Nat. Prod. Rep., 2017, 34, 1302–1331 RSC;
(b) C. C. Barber and W. Zhang, J. Ind. Microbiol. Biotechnol., 2021, 48, kuab010 CrossRef CAS PubMed;
(c) H. Dai, J. Han, T. Wang, W.-B. Yin, Y. Chen and H. Liu, Nat. Prod. Rep., 2023, 40, 1078–1093 RSC.
-
(a) P. Rutledge and G. Challis, Nat. Rev. Microbiol., 2015, 13, 509–523 CrossRef CAS PubMed;
(b) J.-C. Lagier, G. Dubourg, M. Million, F. Cadoret, M. Bilen, F. Fenollar, A. Levasseur, J.-M. Rolain, P.-E. Fournier and D. Raoult, Nat. Rev. Microbiol., 2018, 16, 540–550 CrossRef CAS PubMed.
-
(a) M. S. Donia, P. Cimermancic, C. J. Schulze, L. C. W. Brown, J. Martin, M. Mitreva, J. Clardy, R. G. Linington and M. A. Fischbach, Cell, 2014, 158, 1402–1414 CrossRef CAS PubMed;
(b) H. Sberro, B. J. Fremin, S. Zlitni, F. Edfors, N. Greenfield, M. P. Snyder, G. A. Pavlopoulos, N. C. Kyrpides and A. S. Bhatt, Cell, 2019, 178, 1245–1259 CrossRef CAS PubMed;
(c) G. Aleti, J. L. Baker, X. Tang, R. Alvarez, M. Dinis, N. C. Tran, A. V. Melnik, C. Zhong, M. Ernst, P. C. Dorrestein and A. Edlund, mBio, 2019, 10, 10–1128 CrossRef PubMed.
- Y. Sugimoto, F. R. Camacho, S. Wang, P. Chankhamjon, A. Odabas, A. Biswas, P. D. Jeffrey and M. S. Donia, Science, 2019, 366, eaax9176 CrossRef CAS PubMed.
-
(a) E. E. Shine and J. M. Crawford, Annu. Rev. Biochem., 2021, 90, 789–815 CrossRef CAS PubMed;
(b) A. S. Devlin, Cell Host Microbe, 2022, 30, 435–438 CrossRef CAS PubMed;
(c) A. Hussain, U. Patwekar, D. S. Mongad and Y. S. Shouche, Drug Discovery Today, 2023, 28, 103459 CrossRef CAS PubMed.
- M. H. Medema and M. A. Fischbach, Nat. Chem. Biol., 2015, 11, 639–648 CrossRef CAS PubMed.
- N. Ziemert, M. Alanjary and T. Weber, Nat. Prod. Rep., 2016, 33, 988–1005 RSC.
-
(a) S. M. Schrader, J. Vaubourgeix and C. Nathan, Sci. Transl. Med., 2020, 12, eaaz6992 CrossRef CAS PubMed;
(b) K. Lewis, Cell, 2020, 181, 29–45 CrossRef CAS PubMed;
(c) M. Miethke, M. Pieroni and T. Weber,
et al.
, Nat. Rev. Chem., 2021, 5, 726–749 CrossRef CAS PubMed;
(d)
https://www.who.int/news/item/27-02-2017-who-publishes-list-of-bacteria-for-which-new-antibiotics-are-urgently-needed
.
- B. Y. L. Peisl, E. L. Schymanski and P. Wilmes, Anal. Chim. Acta, 2018, 1037, 13–27 CrossRef CAS PubMed.
- M. DiBello, A. R. Healy, H. Nikolayevskiy, Z. Xu and S. B. Herzon, Acc. Chem. Res., 2023, 56, 1656–1668 CrossRef CAS PubMed.
- A. Bauer and M. Brönstrup, Nat. Prod. Rep., 2014, 31, 35–60 RSC.
-
(a) P. M. Wright, I. B. Seiple and A. G. Myers, Angew. Chem., Int. Ed., 2014, 53, 8840–8869 CrossRef CAS PubMed;
(b) B. J. Huffman and R. A. Shenvi, J. Am. Chem. Soc., 2019, 141, 3332–3346 CrossRef CAS PubMed;
(c) B. Hong, T. Liu and X. Lei, ACS Cent. Sci., 2020, 6, 622–635 CrossRef CAS PubMed;
(d) N. J. Truax and D. Romo, Nat. Prod. Rep., 2020, 37, 1436–1453 RSC;
(e) Z. C. Wu and D. L. Boger, Nat. Prod. Rep., 2020, 37, 1511–1531 RSC;
(f) P. C. Williams, K. M. Wernke, A. Tirlaa and S. B. Herzon, Nat. Prod. Rep., 2020, 37, 1532–1548 RSC;
(g) S. M. Rowe and D. R. Spring, Chem. Soc. Rev., 2021, 50, 4245–4248 RSC;
(h) B. H. Gan, J. Gaynord, S. M. Rowe, T. Deingruber and D. R. Spring, Chem. Soc. Rev., 2021, 50, 7820–7880 RSC;
(i) S. Sengupta, S. Pabbaraja and G. Mehta, Chem. Commun., 2023, 59, 9445–9456 RSC.
-
(a) N. Garg, T. Luzzatto-Knaan, A. V. Melnik, A. M. Caraballo-Rodríguez, D. J. Floros, D. Petras, R. Gregor, P. C. Dorrestein and V. V. Phelan, Nat. Prod. Rep., 2017, 34, 194–219 RSC;
(b) Z. Hu and W. Zhang, ACS Infect. Dis., 2020, 6, 25–33 CrossRef CAS PubMed.
-
(a) G. D. Geske, J. C. O'Neilla and H. E. Blackwell, Chem. Soc. Rev., 2008, 37, 1432–1447 RSC;
(b) D. A. Rasko and V. Sperandio, Nat. Rev. Drug Discovery, 2010, 9, 117–128 CrossRef CAS PubMed;
(c) M. A. Welsh and H. E. Blackwell, FEMS Microbiol. Rev., 2016, 40, 774–794 CrossRef CAS PubMed.
- Y. Kishi, Proc. Natl. Acad. Sci. U. S. A., 2011, 108, 6703–6708 CrossRef CAS PubMed.
-
(a) A. R. Healy and S. B. Herzon, J. Am. Chem. Soc., 2017, 139, 14817–14824 CrossRef CAS PubMed;
(b) J.-W. Tang, X. Liu, W. Ye, Z.-R. Li and P.-Y. Qian, Nat. Prod. Rep., 2022, 39, 991–1014 RSC.
-
(a) G. Schneditz, J. Rentner, S. Roier, J. Pletz, K. A. T. Herzog, R. Bücker, H. Troeger, S. Schild, H. Weber, R. Breinbauer, G. Gorkiewicz, C. Högenauer and E. L. Zechner, Proc. Natl. Acad. Sci. U. S. A., 2014, 111, 13181–13186 CrossRef CAS PubMed;
(b) E. Dornisch, J. Pletz, R. A. Glabonjat, F. Martin, C. Lembacher-Fadum, M. Neger, C. Hçgenauer, K. Francesconi, W. Kroutil, K. Zangger, R. Breinbauer and E. L. Zechner, Angew. Chem., Int. Ed., 2017, 56, 14753–14757 CrossRef CAS PubMed.
-
(a) J. A. McIntosh, M. S. Donia and E. W. Schmidt, Nat. Prod. Rep., 2009, 26, 537–559 RSC;
(b) M. Montalban-Lopez, T. A. Scott, S. Ramesh, I. R. Rahman, A. J. van Heel, J. H. Viel, V. Bandarian, E. Dittmann, O. Genilloud, Y. Goto, M. J. Grande Burgos, C. Hill, S. Kim, J. Koehnke, J. A. Latham, A. J. Link, B. Martinez, S. K. Nair, Y. Nicolet, S. Rebuffat, H.-G. Sahl, D. Sareen, E. W. Schmidt, L. Schmitt, K. Severinov, R. D. Sussmuth, A. W. Truman, H. Wang, J.-K. Weng, G. P. van Wezel, Q. Zhang, J. Zhong, J. Piel, D. A. Mitchell, O. P. Kuipers and W. A. van der Donk, Nat. Prod. Rep., 2021, 38, 130–239 RSC;
(c) A. Benjdia and O. Berteau, Front. Chem., 2021, 9, 474 Search PubMed;
(d) L. Cao, T. Do and A. James, J. Ind. Microbiol. Biotechnol., 2021, 48, kuab005 CrossRef CAS PubMed;
(e) H. Lee and W. A. van der Donk, Annu. Rev. Biochem., 2022, 91, 269–94 CrossRef CAS PubMed;
(f) C. Ongpipattanakul, E. K. Desormeaux, A. DiCaprio, W. A. van der Donk, D. A. Mitchell and S. K. Nair, Chem. Rev., 2022, 122, 14722–14814 CrossRef CAS PubMed;
(g) R. S. Ayikpoe, C. Shi, A. J. Battiste, S. M. Eslami, S. Ramesh, M. A. Simon, I. R. Bothwell, H. Lee, A. J. Rice, H. Ren, Q. Tian, L. A. Harris, R. Sarksian, L. Zhu, A. M. Frerk, T. W. Precord, W. A. van der Donk, D. A. Mitchell and H. Zhao, Nat. Commun., 2022, 13, 6135 CrossRef CAS PubMed;
(h) G. Zhong, Z.-J. Wang, F. Yan, Y. Zhang and L. Huo, ACS Bio Med Chem Au, 2023, 3, 1–31 CrossRef CAS PubMed.
-
(a) M. C. Bagley, J. W. Dale, E. A. Merritt and X. Xiong, Chem. Rev., 2005, 105, 685–714 CrossRef CAS PubMed;
(b) X. Just-Baringo, F. Albericio and M. Álvarez, Mar. Drugs, 2014, 12, 317–351 CrossRef CAS PubMed;
(c) A. A. Vinogradov and H. Suga, Cell Chem. Biol., 2020, 27, 1032–1051 CrossRef CAS PubMed;
(d) D. C. K. Chan and L. L. Burrows, J. Antibiot., 2021, 74, 161–175 CrossRef CAS PubMed.
-
(a) R. Sinha Roy, A. M. Gehring, J. C. Milne, P. J. Belshaw and C. T. Walsh, Nat. Prod. Rep., 1999, 16, 249–263 RSC;
(b) J. O. Melby, N. J. Nard and D. A. Mitchell, Curr. Opin. Chem. Biol., 2011, 15, 369–378 CrossRef CAS PubMed;
(c) I. V. Smolyar, A. K. Yudin and V. G. Nenajdenko, Chem. Rev., 2019, 119, 10032–10240 CrossRef CAS PubMed.
-
(a) C. Chatterjee, M. Paul, L. Xie and W. A. van der Donk, Chem. Rev., 2005, 105, 633–684 CrossRef CAS PubMed;
(b) J. M. Willey and W. A. van der Donk, Annu. Rev. Microbiol., 2007, 61, 477–501 CrossRef CAS PubMed;
(c) A. J. van Heel, M. Montalban-Lopez and O. P. Kuipers, Expert Opin. Drug Metab. Toxicol., 2011, 7, 675–680 CrossRef CAS PubMed.
-
(a) J. B. Broderick, B. R. Duffus, K. S. Duschene and E. M. Shepard, Chem. Rev., 2014, 114, 4229–4317 CrossRef CAS PubMed;
(b) Y. Chen, J. Wang, G. Li, Y. Yang and W. Ding, Front. Chem., 2021, 9, 595991 CrossRef CAS PubMed;
(c) A. Mendauletova, A. Kostenko, Y. Lien and J. Latham, ACS Bio Med Chem Au, 2022, 2, 53–59 CrossRef CAS PubMed;
(d) J. K. A. Clark, L. B. Bushin and M. R. Seyedsayamdost, ACS Bio Med Chem Au, 2022, 2, 328–339 CrossRef PubMed.
-
(a) P. D. Cotter, R. P. Ross and C. Hill, Nat. Rev. Microbiol., 2013, 11, 95–105 CrossRef CAS PubMed;
(b) F. Bédard and E. Biron, Front. Microbiol., 2018, 9, 1048 CrossRef PubMed;
(c) Z. J. Ng, M. A. Zarin, C. K. Lee and J. S. Tan, RSC Adv., 2020, 10, 38937–38964 RSC;
(d) M. do Carmo de Freire Bastos, F. Miceli de Farias, P. Carlin Fagundes and M. L. Varella Coelho, Appl. Microbiol. Biotechnol., 2020, 104, 10339–10368 CrossRef PubMed;
(e) S. Heilbronner, B. Krismer, H. Brötz-Oesterhelt and A. Peschel, Nat. Rev. Microbiol., 2021, 19, 726–739 CrossRef CAS PubMed.
- M. A. Ciufolini and D. Lefranc, Nat. Prod. Rep., 2010, 27, 330–342 RSC.
- Y. Liu, Y. Liu, Z. Du, L. Zhang, J. Chen, Z. Shen, Q. Liu, J. Qin, H. Lv, H. Wang, L. He, J. Liu, Q. Huang, Y. Sun, M. Otto and M. Li, Microbiome, 2020, 8, 85 CrossRef CAS PubMed.
-
(a) S. Akasapu, A. B. Hinds, W. C. Powell and M. A. Walczak, Chem. Sci., 2019, 10, 1971–1975 RSC;
(b) M. P. Christy, T. Johnson, C. D. McNerlin, J. Woodard, A. T. Nelson, B. Lim, T. L. Hamilton, K. M. Freiberg and D. Siegel, Org. Lett., 2020, 22, 2365–2370 CrossRef CAS PubMed;
(c) T. C. Johnson, M. P. Christy and D. Siegel, Synthesis, 2021, 53, 498–508 CrossRef CAS.
-
(a) S. Duquesne, D. Destoumieux-Garzón, J. Peduzzi and S. Rebuffat, Nat. Prod. Rep., 2007, 24, 708–734 RSC;
(b) F. Baquero, V. F. Lanza, M.-R. Baquero, R. Del Campo and D. A. Bravo-Vázquez, Front. Microbiol., 2019, 10, 2261 CrossRef PubMed.
-
(a) F. Collin and A. Maxwell, J. Mol. Biol., 2019, 431, 3400–3425 CrossRef CAS PubMed.
-
(a) G. Videnov, D. Kaiser, M. Brooks and G. Jung, Angew. Chem., Int. Ed. Engl., 1996, 35, 1506–1508 CrossRef CAS;
(b) R. E. Thompson, K. A. Jolliffe and R. J. Payne, Org. Lett., 2011, 13, 680–683 CrossRef CAS PubMed;
(c) R. E. Thompson, F. Collin, A. Maxwell, K. A. Jolliffe and R. J. Payne, Org. Biomol. Chem., 2014, 12, 1570–1578 RSC.
-
(a) R. S. Roy, N. L. Kelleher, J. C. Milne and C. T. Walsh, Chem. Biol., 1999, 6, 305–318 CrossRef CAS PubMed;
(b) D. B. Zamble, D. A. Miller, J. G. Heddle, A. Maxwell, C. T. Walsh and F. Hollfelder, Proc. Natl. Acad. Sci. U. S. A., 2001, 98, 7712–7717 CrossRef CAS PubMed.
-
(a) J. F. Garcia-Bustos, N. Pezzi and E. Mendez, Antimicrob. Agents Chemother., 1985, 27, 791–797 CrossRef CAS PubMed;
(b) J. I. Guijarro, J. E. González-Pastor, F. Baleux, J. L. San Millán, M. A. Castilla, M. Rico, F. Moreno and M. Delepierre, J. Biol. Chem., 1995, 270, 23520–23532 CrossRef CAS PubMed;
(c) K. Severinov, E. Semenova, A. Kazakov, T. Kazakov and M. S. Gelfand, Mol. Microbiol., 2007, 65, 1380–1394 CrossRef CAS PubMed;
(d) R. F. Roush, E. M. Nolan, F. Löhr and C. T. Walsh, J. Am. Chem. Soc., 2008, 130, 3603–3609 CrossRef CAS PubMed.
- L. Liu, T. Hao, Z. Xie, G. P. Horsman and Y. Chen, Sci. Rep., 2016, 6, 37479 CrossRef CAS PubMed.
-
(a) P. M. Joyner, J. Liu, Z. Zhang, J. Merritt, F. Qi and R. H. Cichewicz, Org. Biomol. Chem., 2010, 8, 5486–5489 RSC;
(b) X. Wang, L. Du, J. You, J. B. King and R. H. Cichewicz, Org. Biomol. Chem., 2012, 10, 2044–2050 RSC;
(c) R. Zvanych, N. Lukenda, X. Li, J. J. Kim, S. Tharmarajah and N. A. Magarvey, Mol. BioSyst., 2015, 11, 97–104 RSC.
-
(a) F. Pultar, M. E. Hansen, S. Wolfrum, L. Böselt, R. Fróis-Martins, S. Bloch, A. G. Kravina, D. Pehlivanoglu, C. Schäffer, S. LeibundGut-Landmann, S. Riniker and E. M. Carreira, J. Am. Chem. Soc., 2021, 143, 10389–10402 CrossRef CAS PubMed;
(b) M. E. Hansen, S. O. Yasmin, S. Wolfrum and E. M. Carreira, Angew. Chem., Int. Ed., 2022, 61, e202203051 CrossRef CAS PubMed.
- A. Zipperer, M. C. Konnerth, C. Laux, A. Berscheid, D. Janek, C. Weidenmaier, M. Burian, N. A. Schilling, C. Slavetinsky, M. Marschal, M. Willmann, H. Kalbacher, B. Schittek, H. Brötz-Oesterhelt, S. Grond, A. Peschel and B. Krismer, Nature, 2016, 535, 511–516 CrossRef CAS PubMed.
-
(a) N. A. Schilling, A. Berscheid, J. Schumacher, J. S. Saur, M. C. Konnerth, S. N. Wirtz, J. M. Beltrán-Beleña, A. Zipperer, B. Krismer, A. Peschel, H. Kalbacher, H. Brötz-Oesterhelt, C. Steinem and S. Grond, Angew. Chem., Int. Ed., 2019, 58, 9234–9238 CrossRef CAS PubMed;
(b) J. S. Saur, S. N. Wirtz, N. A. Schilling, B. Krismer, A. Peschel and S. Grond, J. Med. Chem., 2021, 64, 4034–4058 CrossRef CAS PubMed.
- L. R. Malins, J. N. deGruyter, K. J. Robbins, P. M. Scola, M. D. Eastgate, M. R. Ghadiri and P. S. Baran, J. Am. Chem. Soc., 2017, 139, 5233–5241 CrossRef CAS PubMed.
-
(a)
J. Verhoef, D. Milatovic and M. B. Ekkelenkamp, WO/2005/023852, 2005;
(b) M. B. Ekkelenkamp, M. Hanssen, S.-T. D. Hsu, A. de Jong, D. Milatovic, J. Verhoef and N. A. J. van Nuland, FEBS Lett., 2005, 579, 1917–1922 CrossRef CAS PubMed;
(c) T. J. Oman, T. J. Lupoli, T.-S. A. Wang, D. Kahne, S. Walker and W. A. van der Donk, J. Am. Chem. Soc., 2011, 133, 17544–17547 CrossRef CAS PubMed;
(d) J. E. Velásquez, X. Zhang and W. A. van der Donk, Chem. Biol., 2011, 18, 857–867 CrossRef PubMed.
- P. J. Knerr and W. A. van der Donk, J. Am. Chem. Soc., 2012, 134, 7648–7651 CrossRef CAS PubMed.
-
(a) A. B. Tabor, Org. Biomol. Chem., 2011, 9, 7606–7628 RSC;
(b) A. B. Tabor, Bioorg. Chem., 2014, 55, 39–50 CrossRef CAS PubMed;
(c) D. Field, P. D. Cotter, C. Hill and R. P. Ross, Front. Microbiol., 2015, 6, 1363 Search PubMed;
(d) E. L. Ongey and P. Neubauer, Microb. Cell Fact., 2016, 15, 97 CrossRef PubMed;
(e) T. Denoël, C. Lemaire and A. Luxen, Chem. – Eur. J., 2018, 24, 15421–15441 CrossRef PubMed.
-
(a) D. Thuault, E. Beliard, J. Le Guern and C. M. Bourgeois, J. Dairy Sci., 1991, 74, 1145–1150 CrossRef CAS PubMed;
(b) H. W. van den Hooven, F. M. Lagerwerf, W. Heerma, J. Haverkamp, J.-C. Piard, C. W. Hilbers, R. J. Siezen, O. P. Kuipers and H. S. Rollema, FEBS Lett., 1996, 391, 317–322 CrossRef PubMed;
(c) A. Dufour, T. Hindré, D. Haras and J.-P. Le Pennec, FEMS Microbiol. Rev., 2007, 31, 134–167 CrossRef CAS PubMed.
-
(a) P. J. Knerr and W. A. van der Donk, J. Am. Chem. Soc., 2013, 135, 7094–7097 CrossRef CAS PubMed;
(b) M. R. Levengood, P. J. Knerr, T. J. Oman and W. A. van der Donk, J. Am. Chem. Soc., 2009, 131, 12024–12025 CrossRef CAS PubMed;
(c) T. J. Oman, P. J. Knerr, N. A. Bindman, J. E. Velásquez and W. A. van der Donk, J. Am. Chem. Soc., 2012, 134, 6952–6955 CrossRef CAS PubMed;
(d) P. J. Knerr, T. J. Oman, C. V. Garcia De Gonzalo, T. J. Lupoli, S. Walker and W. A. van der Donk, ACS Chem. Biol., 2012, 11, 1791–1795 CrossRef PubMed.
-
(a) M. P. Ryan, M. C. Rea, C. Hill and R. P. Ross, Appl. Environ. Microbiol., 1996, 62, 612–619 CrossRef CAS PubMed;
(b) M. Galvin, C. Hill and R. P. Ross, Lett. Appl. Microbiol., 1999, 28, 355 CrossRef CAS PubMed;
(c) N. I. Martin, T. Sprules, M. R. Carpenter, P. D. Cotter, C. Hill, R. P. Ross and J. C. Vederas, Biochemistry, 2004, 43, 3049–3056 CrossRef CAS PubMed;
(d) J. Carroll, L. A. Draper, P. M. O'Connor, A. Coffey, C. Hill, R. Paul Ross, P. D. Cotter and J. O'Mahony, Int. J. Antimicrob. Agents, 2010, 36, 132–136 CrossRef CAS PubMed;
(e) A. Bakhtiary, S. A. Cochrane, P. Mercier, R. T. McKay, M. Miskolzie, C. S. Sit and J. C. Vederas, J. Am. Chem. Soc., 2017, 139, 17803–17810 CrossRef CAS PubMed.
-
(a) W. Liu, A. S. H. Chan, H. Liu, S. A. Cochrane and J. C. Vederas, J. Am. Chem. Soc., 2011, 133, 14216–14219 CrossRef CAS PubMed;
(b) V. R. Pattabiraman, S. M. K. McKinnie and J. C. Vederas, Angew. Chem., Int. Ed., 2008, 47, 9472–9475 CrossRef CAS PubMed;
(c) H. Liu, V. R. Pattabiraman and J. C. Vederas, Org. Lett., 2009, 11, 5574–5577 CrossRef CAS PubMed.
-
(a) P. S. Coburn and M. S. Gilmore, Cell. Microbiol., 2003, 5, 661–669 CrossRef CAS PubMed;
(b) C. R. Cox, P. S. Coburn and M. S. Gilmore, Curr. Protein Pept. Sci., 2005, 6, 77–84 CrossRef CAS PubMed;
(c) D. van Tyne, M. J. Martin and M. S. Gilmore, Toxins, 2013, 5, 895–911 CrossRef CAS PubMed.
- S. Mukherjee, L. Huo, G. N. Thibodeaux and W. A. van der Donk, Org. Lett., 2016, 18, 6188–6191 CrossRef CAS PubMed . Also see, N. Mazo, I. R. Rahman, C. D. Navo, J. M. Peregrina, J. H. Busto, W. A. van der Donk and G. Jiménez-Osés, Org. Lett., 2023, 25, 1431–1435 CrossRef PubMed.
-
(a) R. C. Hider and X. Kong, Nat. Prod. Rep., 2010, 27, 637–657 RSC;
(b) K. H. Negash, J. K. S. Norris and J. T. Hodgkinson, Molecules, 2019, 24, 3314 CrossRef CAS PubMed;
(c) F. Garzón-Posse, Y. Quevedo-Acosta, C. Mahecha-Mahecha and P. Acosta-Guzmán, Eur. J. Org. Chem., 2019, 7747–7769 CrossRef;
(d) J. Kramer, Ö. Özkaya and R. Kümmerli, Nat. Rev. Microbiol., 2020, 18, 152–163 CrossRef CAS PubMed;
(e) M. J. Miller and R. Liu, Acc. Chem. Res., 2021, 54, 1646–1661 CrossRef CAS PubMed;
(f) B. Rayner, A. D. Verderosa, V. Ferro and M. A. T. Blaskovich, RSC Med. Chem., 2023, 14, 800–822 RSC.
- D. Ferreira, A. M. L. Seca, D. C. G. A. Pinto and A. M. S. Silva, J. Proteomics, 2016, 145, 153–166 CrossRef CAS PubMed.
-
(a) K. N. Raymond, E. A. Dertz and S. S. Kim, Proc. Natl. Acad. Sci. U. S. A., 2003, 100, 3584–3588 CrossRef CAS PubMed;
(b) P. Klahn, R. Zscherp and C. C. Jimidar, Synthesis, 2022, 54, 3499–3557 CrossRef CAS.
-
(a) Y. Takeuchi, S. Ozaki, M. Satoh, K.-I. Mimura, S.-I. Hara, H. Abe, H. Nishioka and T. Harayama, Chem. Pharm. Bull., 2010, 58, 1552–1553 CrossRef CAS PubMed;
(b) J. Kim, J. E. Lee, H. Ree and H. J. Kim, Bull. Korean Chem. Soc., 2015, 36, 439–441 CrossRef CAS;
(c) T. J. Bohac, J. A. Shapiro and T. A. Wencewicz, ACS Infect. Dis., 2017, 3, 802–806 CrossRef CAS PubMed;
(d) J. A. Shapiro and T. A. Wencewicz, Metallomics, 2017, 9, 463–470 CrossRef CAS PubMed;
(e) K. Conde-Pérez, J. C. Vázquez-Ucha, L. Álvarez-Fraga, L. Ageitos, S. Rumbo-Feal, M. Martínez-Guitián, N. Trigo-Tasende, J. Rodríguez, G. Bou, C. Jiménez, A. Beceiro and M. Poza, Front. Microbiol., 2021, 12, 752070 CrossRef PubMed.
-
(a) A. Proschak, P. Lubuta, P. Grün, F. Löhr, G. Wilharm, V. De Berardinis and H. B. Bode, ChemBioChem, 2013, 14, 633–638 CrossRef CAS PubMed;
(b) S. Kim, H. Lee, W. Y. Song and H. J. Kim, Org. Lett., 2020, 22, 2806–2810 CrossRef CAS PubMed;
(c) T. J. Bohac, L. Fang, V. S. Banas, D. E. Giblin and T. A. Wencewicz, ACS Infect. Dis., 2021, 7, 2138–2151 CrossRef CAS PubMed;
(d) D. Y. Kim and H. J. Kim, Org. Lett., 2021, 23, 5256–5260 CrossRef CAS PubMed;
(e) J. Yang and T. A. Wencewicz, ACS Chem. Biol., 2022, 17, 2923–2935 CrossRef CAS PubMed.
-
(a) J. S. McFarlane and A. L. Lamb, Biochemistry, 2017, 56, 5967–5971 CrossRef CAS PubMed;
(b) J. Zhang, T. Zhao, R. Yang, I. Siridechakorn, S. Wang, Q. Guo, Y. Bai, H. C. Shenb and X. Lei, Chem. Sci., 2019, 10, 6635–6641 RSC;
(c) G. Cullia, R. Fanelli, R. Voulhoux, P. Arnoux and F. Cavelier, Eur. J. Org. Chem., 2020, 3975–3980 CrossRef CAS.
-
(a) J. M. Meyer, A. Neely, A. Stintzi, C. Georges and I. A. Holder, Infect. Immun., 1996, 64, 518–523 CrossRef CAS PubMed;
(b) R. Mashiach and M. M. Meijler, Org. Lett., 2013, 15, 1702–1705 CrossRef CAS PubMed.
- J. Chu, X. Vila-Farres, D. Inoyama, M. Ternei, L. J. Cohen, E. A. Gordon, B. V. B. Reddy, Z. Charlop-Powers, H. A. Zebroski, R. Gallardo-Macias, M. Jaskowski, S. Satish, S. Park, D. S. Perlin, J. S. Freundlich and S. F. Brady, Nat. Chem. Biol., 2016, 12, 1004 CrossRef CAS PubMed.
- J. Chu, X. Vila-Farres, D. Inoyama, R. Gallardo-Macias, M. Jaskowski, S. Satish, J. S. Freundlich and S. F. Brady, ACS Infect. Dis., 2018, 4, 33–38 CrossRef CAS PubMed.
-
(a) X. Vila-Farres, J. Chu, D. Inoyama, M. A. Ternei, C. Lemetre, L. J. Cohen, W. Cho, B. V. B. Reddy, H. A. Zebroski, J. S. Freundlich, D. S. Perlin and S. F. Brady, J. Am. Chem. Soc., 2017, 139, 1404–1407 CrossRef CAS PubMed;
(b) X. Vila-Farres, J. Chu, M. A. Ternei, C. Lemetre, S. Park, D. S. Perlin and S. F. Brady, mSphere, 2018, 3, e00528–17 CrossRef CAS PubMed;
(c) J. Chu, B. Koirala, N. Forelli, X. Vila-Farres, M. A. Ternei, T. Ali, D. A. Colosimo and S. F. Brady, J. Am. Chem. Soc., 2020, 142, 14158–14168 CrossRef CAS PubMed;
(d) M. A. Hostetler, C. Smith, S. Nelson, Z. Budimir, R. Modi, I. Woolsey, A. Frerk, B. Baker, J. Gantt and E. I. Parkinson, ACS Chem. Biol., 2021, 16, 2604–2611 CrossRef CAS PubMed;
(e) Z. Wang, B. Koirala, Y. Hernandez, M. Zimmerman, S. Park, D. S. Perlin and S. F. Brady, Nature, 2022, 601, 606–611 CrossRef CAS PubMed;
(f) Z. Wang, B. Koirala, Y. Hernandez, M. Zimmerman and S. F. Brady, Science, 2022, 376, 991–996 CrossRef CAS PubMed;
(g) C. Wu, Y. Yin, L. Zhu, Y. Zhang and Y.-Z. Li, Drug Discovery Today, 2022, 27, 730–742 CrossRef CAS PubMed.
-
(a) Y. Ma, Z. Guo, B. Xia, Y. Zhang, X. Liu, Y. Yu, N. Tang, X. Tong, M. Wang, X. Ye, J. Feng, Y. Chen and J. Wang, Nat. Biotechnol., 2022, 40, 921–931 CrossRef CAS PubMed;
(b) F. R. Fields, S. D. Freed, K. E. Carothers, M. N. Hamid, D. E. Hammers, J. N. Ross, V. R. Kalwajtys, A. J. Gonzalez, A. D. Hildreth, I. Friedberg and S. W. Lee, Drug Dev. Res., 2020, 81, 43–51 CrossRef CAS PubMed;
(c) B. A. Schneider and E. P. Balskus, Tetrahedron, 2018, 74, 3215–3230 CrossRef CAS.
-
(a) Y. Gerardin, S. Timberlake, J. R. Allegretti, M. B. Smith and Z. Kassam, J. Infect. Dis., 2021, 223, S276–S282 CrossRef CAS PubMed;
(b) Y. Zhang, A. S. Fleur and H. Feng, Gut Microbes, 2022, 14, e2052698 CrossRef PubMed;
(c) C. W. J. McChalicher and J. G. Auniņš, Curr. Opin. Biotechnol., 2022, 78, 102801 CrossRef CAS PubMed.
-
(a) T. Louie;, Y. Golan, S. Khanna, D. Bobilev, N. Erpelding, C. Fratazzi, M. Carini, R. Menon, M. Ruisi, J. M. Norman, J. J. Faith, B. Olle, M. Li, J. L. Silber and D. S. Pardi, J. Am. Med. Assoc., 2023, 329, 1356–1366 CrossRef PubMed;
(b)
https://www.vedantabio.com/news-media/press-releases/detail/3025/vedanta-biosciences-announces-first-patient-dosed-in-phase
.
-
(a) E. L. Ongey, H. Yassi, S. Pflugmacher and P. Neubauer, Biotechnol. Lett., 2017, 39, 473–482 CrossRef PubMed;
(b) L. R. Heinzinger, A. R. Pugh, J. A. Wagner and M. Otto, Antibiotics, 2023, 12, 1256 CrossRef CAS PubMed.
-
(a) J. M. Shin, J. W. Gwak, P. Kamarajan, J. C. Fenno, A. H. Rickard and Y. L. Kapila, J. Appl. Microbiol., 2015, 120, 1449–1465 CrossRef PubMed;
(b) M. Musiejuk and P. Kafarski, Pharmaceuticals, 2023, 16, 1058 CrossRef CAS PubMed;
(c) D. Field, M. F. de Ullivari, R. P. Ross and C. Hill, FEMS Microbiol. Rev., 2023, 47, 1–18 CrossRef PubMed;
(d) L. Guo, J. Wambui, C. Wang, F. Muchaamba, M. V. Fernandez-Cantos, J. Broose, T. Tasara, O. P. Kuipers and R. Stephan, Microbiol. Spectr., 2023, 11, e05319–22 Search PubMed.
-
(a) I. Sakamoto, M. Igarishi, K. Kimura, A. Takagi, T. Miwa and Y. Koga, J. Antimicrob. Chemother., 2001, 47, 709–710 CrossRef CAS PubMed;
(b) L. Fernández, S. Delgado, H. Herrero, A. Maldonado and J. M. Rodríguez, J. Hum. Lact., 2008, 24, 311–316 CrossRef PubMed;
(c)
https://clinicaltrials.gov/study/NCT02928042
;
(d) D. Mitra, A. Yadav, S. Prithyani, L. E. John, S. Rodrigues and R. Shah, J. Indian Soc. Periodontol., 2019, 23, 31–34 CrossRef PubMed.
-
(a) R. Dickman, S. A. Mitchell, A. M. Figueiredo, D. F. Hansen and A. B. Tabor, J. Org. Chem., 2019, 84, 11493–11512 CrossRef CAS PubMed;
(b) R. Dickman, E. Danelius, S. A. Mitchell, D. F. Hansen, M. Erdélyi and A. B. Tabor, Chem. – Eur. J., 2019, 25, 14572–14582 CrossRef CAS PubMed.
-
(a) S. Sandiford and M. Upton, Antimicrob. Agents Chemother., 2012, 56, 1539–1547 CrossRef CAS PubMed;
(b) S. Halliwell, P. Warn, A. Sattar, J. P. Derrick and M. Upton, J. Antimicrob. Chemother., 2017, 72, 778–781 CAS;
(c)
https://www.amprologix.com/project-ni01
.
-
(a) J. D. Hillman, J. Novák, E. Sagura, J. A. Gutierrez, T. A. Brooks, P. J. Crowley, M. Hess, A. Azizi, K.-P. Leung, D. Cvitkovitch and A. S. Bleiweis, Infect. Immun., 1998, 66, 2743–2749 CrossRef CAS PubMed;
(b) L. Smith, C. Zachariah, R. Thirumoorthy, J. Rocca, J. Novák, J. D. Hillman and A. S. Edison, Biochemistry, 2003, 42, 10372–10384 CrossRef CAS PubMed;
(c) L. Smith, H. Hasper, E. Breukink, J. Novak, J. Cerkasov, J. D. Hillman, S. Wilson-Stanford and R. S. Orugunty, Biochemistry, 2008, 47, 3308–3314 CrossRef CAS PubMed;
(d) O. Ghobrial, H. Derendorf and J. D. Hillman, J. Pharm. Sci., 2010, 99, 2521–2528 CrossRef CAS PubMed;
(e) R. Pokhrel, N. Bhattarai, P. Baral, B. S. Gerstman, J. H. Park, M. Handfield and P. P. Chapagain, Phys. Chem. Chem. Phys., 2019, 21, 12530–12539 RSC.
-
(a) S. Chen, S. Wilson-Stanford, W. Cromwell, J. D. Hillman, A. Guerrero, C. A. Allen, J. A. Sorg and L. Smith, Appl. Environ. Microbiol., 2013, 79, 4015–4023 CrossRef CAS PubMed;
(b) J. Escano, A. Ravichandran, B. Salamat and L. Smith, Appl. Environ. Microbiol., 2017, 83, e00668–17 CrossRef CAS PubMed.
-
(a) J. A. Kers, R. E. Sharp, S. Muley, M. Mayo, J. Colbeck, Y. Zhu, A. W. DeFusco, J. H. Park and M. Handfield, Chem. Biol. Drug Des., 2018, 92, 1940–1953 CrossRef CAS PubMed;
(b) J. A. Kers, R. E. Sharp, A. W. Defusco, J. H. Park, J. Xu, M. E. Pulse, W. J. Weiss and M. Handfield, Front. Microbiol., 2018, 9, 415 CrossRef PubMed;
(c) M. Geng, A. Ravichandran, J. Escano and L. Smith, Antimicrob. Agents Chemother., 2018, 62, e01626–18 CrossRef PubMed;
(d) N. V. Rajeshkumara, J. A. Kers, S. Moncrief, A. W. Defuscoc, J. H. Park and M. Handfield, Toxicol. Appl. Pharmacol., 2019, 379, 32–40 CrossRef PubMed;
(e) M. Ju, T. Joseph, N. Hansanant, M. Geng, M. Williams, A. Cothrell, A. R. Buhrow, F. Austin and L. Smith, Front. Microbiol., 2022, 13, 1067410 CrossRef PubMed.
-
(a) J. A. Kers, A. W. DeFusco, J. H. Park, J. Xu, M. E. Pulse, W. J. Weiss and M. Handfield, PLoS One, 2018, 13, e0197467 CrossRef PubMed;
(b) M. E. Pulse, W. J. Weiss, J. A. Kers, A. W. DeFusco, J. H. Park and M. Handfield, Antimicrob. Agents Chemother., 2019, 63, e01904–1 CrossRef CAS PubMed.
-
(a) G. Hannig, B. Tchernychev, C. B. Kurtz, A. P. Bryant, M. G. Currie and I. Silos-Santiago, Front. Mol. Neurosci., 2014, 7, 31 CAS;
(b) S. A. Waldman and M. A. Camilleri, Gut, 2018, 67, 1543–1552 CrossRef CAS PubMed;
(c) A. Fretzen, Bioorg. Med. Chem., 2018, 26, 2863–2872 CrossRef CAS PubMed;
(d) S. M. Brierley, L. Grundy, J. Castro, A. M. Harrington, G. Hannig and M. Camilleri, Trends Pharmacol. Sci., 2022, 43, 110–122 CrossRef CAS PubMed.
-
(a) R. W. Busby, M. M. Kessler, W. P. Bartolini, A. P. Bryant, G. Hannig, C. S. Higgins, R. M. Solinga, J. V. Tobin, J. D. Wakefield, C. B. Kurtz and M. G. Currie, J. Pharmacol. Exp. Ther., 2013, 344, 196–206 CrossRef CAS PubMed;
(b) M. Corsetti and J. Tack, United Eur. Gastroenterol. J., 2013, 1, 7–20 CrossRef PubMed;
(c) P. L. McCormack, Drugs, 2014, 74, 53–60 CrossRef CAS PubMed.
-
(a) Z. T. Al-Salama and Y. Y. Syed, Drugs, 2017, 77, 593–598 CrossRef CAS PubMed;
(b) G. Bassotti, P. U. Satta and M. Bellini, Expert Rev. Clin. Pharmacol., 2019, 12, 1019–1026 CrossRef CAS PubMed;
(c)
Y. G. Shen, Z. L. Xie, Y. M. Cheng, Y. L. Shen and K. A. Fu, P. R. China Patent CN108440652A, 2018 Search PubMed;
(d) H. Li, J. Chao, Z. Zhang, G. Tian, J. Li, N. Chang and C. Qin, Org. Lett., 2020, 22, 3323–3328 CrossRef CAS PubMed.
-
(a) K. Shailubhai, V. Palejwala, K. P. Arjunan, S. Saykhedkar, B. Nefsky, J. A. Foss, S. Comiskey, G. S. Jacob and S. E. Plevy, World J. Gastrointest. Pharmacol. Ther., 2015, 6, 213–222 CrossRef PubMed;
(b) D. S. Weinberg, N. R. Foster, G. D. Zanna, R. P. McMurray, W. K. Kraft, A. Pallotto, D. M. Kastenberg, L. C. Katz, C. H. Henry, S. M. Moleski, P. J. Limburg and S. A. Waldman, Cancer Biol. Ther., 2021, 22, 544–553 CrossRef CAS PubMed;
(c)
https://clinicaltrials.gov/study/NCT01983306
.
-
(a) M. Góngora-Benítez, J. Tulla-Puche, M. Paradís-Bas, O. Werbitzky, M. Giraud and F. Albericio, Pept. Sci., 2011, 96, 69–80 CrossRef PubMed;
(b) C. Chen, S. Gao, Q. Qu, P. Mi, A. Tao and Y.-M. Li, Chin. Chem. Lett., 2018, 29, 1135–1138 CrossRef CAS;
(c) S. Laps, F. Atamleh, G. Kamnesky, H. Sun and A. Brik, Nat. Commun., 2021, 12, 870 CrossRef CAS PubMed;
(d) N. B. Emidio, H. N. T. Tran, A. Andersson, P. E. Dawson, F. Albericio, I. Vetter and M. Muttenthaler, J. Med. Chem., 2021, 64, 8384–8390 CrossRef PubMed;
(e) Z. Qiu, X. Dai, C. Fan, Y. Cao, Z. Lv, X. Liang and F. Meng, Molecules, 2023, 28, 1007 CrossRef CAS PubMed.
- Y. Sugimoto, F. R. Camacho, S. Wang, P. Chankhamjon, A. Odabas, A. Biswas, P. D. Jeffrey and M. S. Donia, Science, 2019, 366, eaax9176 CrossRef CAS PubMed.
-
(a) M. S. Donia, P. Cimermancic, C. J. Schulze, L. C. W. Brown, J. Martin, M. Mitreva, J. Clardy, R. G. Linington and M. A. Fischbach, Cell, 2014, 158, 1402–1414 CrossRef CAS PubMed;
(b) J. Claesen, J. B. Spagnolo, S. F. Ramos, K. L. Kurita, A. L. Byrd, A. A. Aksenov, A. V. Melnik, W. R. Wong, S. Wang, R. D. Hernandez, M. S. Donia, P. C. Dorrestein, H. H. Kong, J. A. Segre, R. G. Linington, M. A. Fischbach and K. P. Lemon, Sci. Transl. Med., 2020, 12, eaay5445 CrossRef CAS PubMed.
- L. B. Bushin, B. C. Covington, B. E. Rued, M. J. Federle and M. R. Seyedsayamdost, J. Am. Chem. Soc., 2020, 142, 16265–16275 CrossRef CAS PubMed.
-
(a) B. E. Rued, B. C. Covington, L. B. Bushin, G. Szewczyk, I. Laczkovich, M. R. Seyedsayamdost and M. J. Federle, mBio, 2021, 12, e02688–20 CAS;
(b) L. B. Bushin, K. A. Clark, I. Pelczer and M. R. Seyedsayamdost, J. Am. Chem. Soc., 2018, 140, 17674–17684 CrossRef CAS PubMed.
-
(a) E. H. Crost, E. H. Ajandouz, C. Villard, P. A. Geraert, A. Puigserver and M. Fons, Biochimie, 2011, 93, 1487–1494 CrossRef CAS PubMed;
(b) S. Chiumento, C. Roblin, S. Kieffer-Jaquinod, S. Tachon, C. Leprètre, C. Basset, D. Aditiyarini, H. Olleik, C. Nicoletti, O. Bornet, O. Iranzo, M. Maresca, R. Hardré, M. Fons, T. Giardina, E. Devillard, F. Guerlesquin, Y. Couté, M. Atta, J. Perrier, M. Lafond and V. Duarte, Sci. Adv., 2019, 5, eaaw9969 CrossRef CAS PubMed;
(c) C. Balty, A. Guillot, L. Fradale, C. Brewee, M. Boulay, X. Kubiak, A. Benjdia and O. Berteau, J. Biol. Chem., 2019, 294, 14512–14525 CrossRef CAS PubMed;
(d) C. Roblin, S. Chiumento, O. Bornet, M. Nouailler, C. S. Müller, K. Jeannot, C. Basset, S. Kieffer-Jaquinod, Y. Couté, S. Torelli, L. Le Pape, V. Schünemann, H. Olleik, B. De La Villeon, P. Sockeel, E. Di Pasquale, C. Nicoletti, N. Vidal, L. Poljak, O. Iranzo, T. Giardina, M. Fons, E. Devillard, P. Polard, M. Maresca, J. Perrier, M. Atta, F. Guerlesquin, M. Lafond and V. Duarte, Proc. Natl. Acad. Sci. U. S. A., 2020, 117, 19168–19177 CrossRef CAS PubMed;
(e) C. Roblin, S. Chiumento, C. Jacqueline, E. Pinloche, C. Nicoletti, H. Olleik, E. Courvoisier-Dezord, A. Amouric, C. Basset, L. Dru, M. Ollivier, A. Bogey-Lambert, N. Vidal, M. Atta, M. Maresca, E. Devillard, V. Duarte, J. Perrier and M. Lafond, Int. J. Mol. Sci., 2021, 22, 3253 CrossRef CAS PubMed;
(f) L. Shamseddine, C. Roblin, I. Veyrier, C. Basset, L. De Macedo, A. Boyeldieu, M. Maresca, C. Nicoletti, G. Brasseur, S. Kieffer-Jaquinod, E. Courvoisier-Dezord, A. Amouric, P. Carpentier, N. Campo, M. Bergé, P. Polard, J. Perrier, V. Duarte and M. Lafond, iScience, 2023, 26, 107563 CrossRef CAS PubMed.
- K. D. Milewska and L. R. Malins, Org. Lett., 2022, 24, 3680–3685 CrossRef CAS PubMed.
- F. Götz, S. Perconti, P. Popella, R. Werner and M. Schlag, Int. J. Med. Microbiol., 2014, 304, 63–71 CrossRef PubMed.
- E. S. Grant-Mackie, E. T. Williams, P. W. R. Harris and M. A. Brimble, JACS Au, 2021, 1, 1527–1540 CrossRef CAS PubMed.
-
(a) C. W. Johnston, M. A. Wyatt, X. Li, A. Ibrahim, J. Shuster, G. Southam and N. A. Magarvey, Nat. Chem. Biol., 2013, 9, 241–243 CrossRef CAS PubMed;
(b) N. Tejman-Yarden, A. Robinson, Y. Davidov, A. Shulman, A. Varvak, F. Reyes, G. Rahav and I. Nissan, Front. Microbiol., 2019, 10, 2377 CrossRef PubMed.
- Y. Zhao, X. Zhong, J. Yan, C. Sun, X. Zhao and X. Wang, Front. Microbiol., 2022, 13, 956378 CrossRef PubMed.
- F. Baquero, K. Beis, D. J. Craik, Y. Li, A. J. Link, S. Rebuffat, R. Salomón, K. Severinov, S. Zirah and J. D. Hegemann, Nat. Prod. Rep., 2024, 41, 469–511 RSC.
- M. Metelev, A. Arseniev, L. B. Bushin, K. Kuznedelov, T. O. Artamonova, R. Kondratenko, M. Khodorkovskii, M. R. Seyedsayamdost and K. Severinov, ACS Chem. Biol., 2017, 12, 814–824 CrossRef CAS PubMed.
-
(a) A. Kirschning, F. Tafta and T. Knobloch, Org. Biomol. Chem., 2007, 5, 3245–3259 RSC;
(b) J. Kennedy, Nat. Prod. Rep., 2008, 25, 25–34 RSC;
(c) R. J. M. Goss, S. Shankar and A. A. Fayad, Nat. Prod. Rep., 2012, 29, 870–889 RSC;
(d) A. Kirschning and F. Hahn, Angew. Chem., Int. Ed., 2012, 51, 4012–4022 CrossRef CAS PubMed;
(e) W. J. Wever, J. W. Bogart, J. A. Baccile, A. N. Chan, F. C. Schroeder and A. A. Bowers, J. Am. Chem. Soc., 2015, 137, 3494–3497 CrossRef CAS PubMed;
(f) E. L. Ongey and P. Neubauer, Microb. Cell Fact., 2016, 15, 97 CrossRef PubMed.
- Y. Zhao, X. Zhong, J. Yan, C. Sun, X. Zhao and X. Wang, Front. Microbiol., 2022, 13, 956378 CrossRef PubMed.
- A. Y. M. Woo, M. A. A. Ramos, R. Narayan, K. C. Richards-Corke, M. L. Wang, W. J. Sandoval-Espinola and E. P. Balskus, Nat. Rev. Chem., 2023, 7, 319–339 CrossRef CAS PubMed.
-
(a) V. K. Gupta, S. Paul and C. Dutta, Front. Microbiol., 2017, 8, 1162 CrossRef PubMed;
(b) R. J. Abdill, E. M. Adamowicz and R. Blekhman, PLoS Biol., 2022, 20, e3001536 CrossRef CAS PubMed;
(c) J. S. Zheng, Nature, 2023 Search PubMed
https://www.nature.com/articles/d41586-023-01843-y
.
Footnote |
† Dedicated with profound respect and admiration to Prof. Sukh Dev for his inspirational leadership. |
|
This journal is © The Royal Society of Chemistry 2024 |
Click here to see how this site uses Cookies. View our privacy policy here.