Expedient, regioselective C–H chalcogenation of 3,4-dihydro-1,4-benzoxazines using a palladium–copper catalyst†
Received
31st March 2024
, Accepted 24th June 2024
First published on 24th June 2024
Abstract
The palladium-catalysed regioselective C–H chalcogenation of benzoxazines with disulfides and diselenides in air has been described. In this protocol, palladium acetate serves as the catalyst in conjunction with copper as an oxidizing agent. Through this approach, a wide array of sulfenylation and selenylation reactions of benzomorpholines have been effected, yielding results ranging from good to excellent. Thus, the established procedure demonstrates superb regioselectivity and a strong tolerance towards various functional groups and is suitable for gram-scale synthesis. Additionally, this synthetic approach offers a practical and convenient pathway for late-stage functionalization leading to the Rosenmund–von Braun reaction.
Introduction
3,4-Dihydro-1,4-benzoxazines are important bioactive heterocyclic scaffolds found in many natural products and pharmaceutical agents.1 1,4-Benzoxazine derivatives are phosphatidylinositol 3-kinase (PI3K) inhibitors that effectively suppress the proliferation of malignant cells, making them useful therapeutic drugs for the treatment of cancer.2 They also find application as intrinsic KDR activity inhibitors, exhibiting potent angiogenic effects (Fig. 1).3
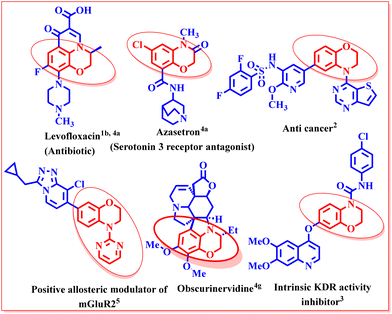 |
| Fig. 1 1,4-Benzoxazines as constituents of significant bioactive moieties. | |
These molecules have potential applications in the treatment of autoimmune disorders, inflammatory-neurodegenerative diseases, diabetes, asthma, and other life-threatening ailments.4 Pyrimidinyl-benzoxazine compounds are effective at treating neurological and psychiatric conditions linked to glutamate dysfunction since they act as positive allosteric modulators of the metabotropic glutamate receptor (mGluR2).5 Consequently, benzo[b][1,4]oxazines are identified as desirable moieties for the creation of drug-like molecules in drug development.6 Benzoxazine moieties also find application in materials sciences.7 Benzoxazine polymers are halogen-free, and they outperform epoxies in terms of flame resistance, electrical properties, superior high temperature resistance and low water absorption values, making them useful for applications such as composites, coatings, adhesives, and encapsulants.8 Owing to such known and potential benefits, the 3,4-dihydro-1,4-benzoxazine moiety has sparked a lot of interest among researchers.9
Furthermore, since the photo-physical and electrical properties of compounds are significantly changed by the introduction of sulfur or selenium into the framework of organic molecules,10 the development of effective catalytic methodologies for C–S and C–Se bond formation reactions has emerged as an intriguing research area. Apart from that, chalcogenation reactions have gained significance owing to the growing importance of organosulfur and organoselenium compounds in pharmaceutical and materials sciences.11 Polyfunctionalised organosulfur motifs also play important roles in the field of photoelectric materials because of the higher resonance energy of the sulfur atom than other heteroatoms.12 These are also explored as fluorescent probes, catalysts in organic synthesis and functional organic materials.13 Considering the efficacy of the organo-chalcogenated products, it has been an ad rem for us to devise a methodology to introduce chalcogenides into benzoxazines. To accomplish this, we utilised the inherent directing abilities of the nitrogen atom in pyrimidine as a tool. The pyrimidine unit not only helped in the regioselective chalcogenation of the benzoxazine molecule but also enhanced its biological activity, as evident from the bioactivity of recent FDA-approved drugs.14
Among the different methodologies developed for the synthesis of chalcogenated molecules such as cross-coupling, intermolecular addition, intramolecular C–H functionalisation and intermolecular C–H functionalisation, the last one needs more attention due to it being less explored.15 Many metals such as Cu, Co, Ni, Rh, Ru, etc. have been employed for affording chalcogenated products,16 but palladium catalysis has become the most intriguing and effective method for producing value-added functionalized compounds.17 Due to the strong coordination of sulfenylating agents with palladium catalytic species, the reaction is rendered ineffective, making palladium-catalysed chalcogenation reactions relatively less well-known than those catalysed by other metal catalysts.18 Nevertheless, palladium-catalyzed C–S and C–Se bond formation is often desirable because it can successfully build structurally varied unsymmetrical aryl(alkyl) sulfides and selenides under mild conditions with excellent regioselectivity.19
Following the ground-breaking work on the direct sulfenylation of arene C–H bonds using diphenyl disulfide and thiophenol as coupling partners by Yu and co-workers, many groups have explored chalcogenation using different substrates and different chalcogenating partners.20 However, these techniques are inefficient, have a limited range of substrate scope and require strong conditions for carrying out sulfenylation and selenylation reactions. Therefore, it is strongly warranted to develop a general and moderate protocol for the direct chalcogenation of arenes. In our quest to demonstrate our accomplishment in chalcogenation, we hereby report the sulfenylation and selenylation of 3,4-dihydro-2H-benzo[b][1,4]oxazine using a palladium catalyst. Although this moiety has many proven and potential benefits, it has been sparingly explored by the chemists for C–H activation. Very recently, our group has carried out the acetoxylation of the 3,4-dihydro-2H-benzo[b][1,4]oxazine moiety and apart from that Chen et al. have reported its hydroxylation.21 To the best of our knowledge, there is no chalcogenation report available on this scaffold.
Optimisation of reaction conditions
The optimisation reaction was carried out with 4-(pyrimidin-2-yl)-3,4-dihydro-2H-benzo[b][1,4]oxazine (1a) and diphenyl disulfide (2a) as chalcogenating partners using Pd(OAc)2 in the presence of CuBr2 as the oxidant and DMF as the solvent. At the outset, 4-(pyrimidin-2-yl)-3,4-dihydro-2H-benzo[b][1,4]oxazine (1a) and diphenyl disulfide (0.6 equiv.) (2a) were allowed to react with DMF at 140 °C in the presence of CuBr2 (2 equiv.) as the oxidant and Pd(OAc)2 (10 mol%) as the catalyst. After 24 h of reaction, the desired product 5-(phenylthio)-4-(pyrimidin-2-yl)-3,4-dihydro-2H-benzo[b][1,4]oxazine (3a) was obtained in 64% yield (Table 1, entry 1). Improvement in the product yield to 89% was obtained when the reaction was performed at a lower temperature of 120 °C (Table 1, entry 2). However, an inferior product yield was obtained when the reaction temperature was lowered further due to incomplete reaction (Table 1, entries 3 and 4). Inferior product yields were also obtained on changing the reaction solvent from DMF to DMSO, DMA, and water (Table 1, entries 5–8). Even changing the catalyst to Pd(TFA)2, PdCl2, Pd(PPh3)Cl2 and Pd2dba3 did not have a beneficial effect on the outcome of this reaction (Table 1, entries 9–12). Moreover, changing the oxidant from CuBr2 to CuCl2 and Cu(OAc)2 could not provide any improvement in the product yield. When screened with oxidants such as CuCl2, Cu(OAc)2 and CuO, it provided 80%, 49% and 56% product yields, respectively (Table 1, entries 13–15). The decrease in the reaction time had a detrimental effect on the product yield (Table 1, entries 16–17) due to incomplete reactions. However, with the increased reaction time, the product yield improved marginally to 90% (Table 1, entry 18). The decrease in the catalyst loading decreased the product yield substantially to 83%, although the yield remained almost stagnant when the catalyst loading was increased further (Table 1, entries 22 and 23). To our surprise, when we lowered the oxidant loading to 1.5 equiv., the product yield improved to 96%; however, further reduction of the oxidant loading to 1 equiv. lowered the product yield, signifying the multiple roles of CuBr2 in this reaction. Moreover, an increase in the oxidant loading is undesirable for this transformation (Table 1, entries 19–21). To establish the significance of the catalyst and the oxidant, blank experiments were carried out. The first experiment was performed in the absence of the catalyst Pd(OAc)2, where no product formation could be seen. On the other hand, when the reaction was carried out in the absence of the oxidant CuBr2, the product was observed in trace amounts (Table 1, entries 24 and 25). These experiments demonstrated the essential role of both in this transformation. Finally, the optimised conditions have been established as 4-(pyrimidin-2-yl)-3,4-dihydro-2H-benzo[b][1,4]oxazine, 0.6 equiv. of commercially available diphenyl disulfide, 10 mol% of Pd(OAc)2, and 1.5 equiv. of CuBr2 in DMF as the solvent.
Table 1 Optimisation of the reaction conditionsa

|
Sr. No. |
Catalyst (10 mol%) |
Oxidant (2 equiv.) |
Solvent (1.5 mL) |
Temp. (°C) |
Time (h) |
Yieldb (%) |
Reagents and conditions: 4-(pyrimidin-2-yl)-3,4-dihydro-2H-benzo[b][1,4]oxazine (1a) and diphenyl disulfide (0.6 equiv.) (2a), catalyst (10 mol%), and oxidant (2 equiv.) were stirred in DMF at 140 °C in open air.
Isolated yields.
Reaction was carried out in a sealed tube.
1.5 equiv. of CuBr2 was used.
1 equiv. CuBr2 was used.
2.5 equiv. of CuBr2 was used.
5 mol% of Pd(OAc)2 and 1.5 equiv. of CuBr2 were used.
15 mol% of Pd(OAc)2 and 1.5 equiv. of CuBr2 were used.
|
1. |
Pd(OAc)2 |
CuBr2 |
DMF |
140 |
24 |
64 |
2. |
Pd(OAc)2 |
CuBr2 |
DMF |
120 |
24 |
89 |
3. |
Pd(OAc)2 |
CuBr2 |
DMF |
110 |
24 |
86 |
4. |
Pd(OAc)2 |
CuBr2 |
DMF |
100 |
24 |
74 |
5. |
Pd(OAc)2 |
CuBr2 |
Dioxane |
120 |
24 |
52c |
6. |
Pd(OAc)2 |
CuBr2 |
DMSO |
120 |
24 |
48 |
7. |
Pd(OAc)2 |
CuBr2 |
DMA |
120 |
24 |
24 |
8. |
Pd(OAc)2 |
CuBr2 |
H2O |
120 |
24 |
47c |
9. |
Pd(TFA)2 |
CuBr2 |
DMF |
120 |
24 |
62 |
10. |
PdCl2 |
CuBr2 |
DMF |
120 |
24 |
44 |
11. |
Pd(PPh3)2Cl2 |
CuBr2 |
DMF |
120 |
24 |
40 |
12. |
Pd2(dba)3 |
CuBr2 |
DMF |
120 |
24 |
52 |
13. |
Pd(OAc)2 |
CuCl2 |
DMF |
120 |
24 |
80 |
14. |
Pd(OAc)2 |
Cu(OAc)2 |
DMF |
120 |
24 |
49 |
15. |
Pd(OAc)2 |
CuO |
DMF |
120 |
24 |
56 |
16. |
Pd(OAc)2 |
CuBr2 |
DMF |
120 |
12 |
69 |
17. |
Pd(OAc)2 |
CuBr2 |
DMF |
120 |
18 |
82 |
18. |
Pd(OAc)2 |
CuBr2 |
DMF |
120 |
30 |
90 |
19. |
Pd(OAc)2 |
CuBr2 |
DMF |
120 |
24 |
96d |
20. |
Pd(OAc)2 |
CuBr2 |
DMF |
120 |
24 |
80e |
21. |
Pd(OAc)2 |
CuBr2 |
DMF |
120 |
24 |
87f |
22. |
Pd(OAc)2 |
CuBr2 |
DMF |
120 |
24 |
83g |
23. |
Pd(OAc)2 |
CuBr2 |
DMF |
120 |
24 |
92h |
24. |
— |
CuBr2 |
DMF |
120 |
24 |
NR |
25. |
Pd(OAc)2 |
— |
DMF |
120 |
24 |
Traces |
Evaluation of substrate scope
With the optimised conditions in hand, an array of 4-(pyrimidin-2-yl)-3,4-dihydro-2H-benzo[b][1,4]oxazines were investigated for sulfenylation to assess the scope and generality of the reaction (Scheme 1). The reaction with unsubstituted substrates delivered the highest product yield of 96%. In general, the reactions were well tolerated with both electron-donating benzoxazines (–H, –CH3, and –NHCOCH3) and electron-withdrawing benzoxazines (halogens and –NO2) (Scheme 1, entries 3a, 3i, 3j, 3k, 3l, and 3m). Various differently substituted diaryl disulfides were subjected to this transformation. Regardless of their electronic character, all of them, for example, the electron-releasing diphenyl disulfides (–H, –OCH3, and –CH3) at para-positions, provided sulfenylated products in good to excellent yields (Scheme 1, entries 3a, 3b, and 3c). Although –CH3 or –OCH3 groups in the disulfides have a higher inductive effect than –H, the latter provided a better product yield, presumably due to steric reasons. Gratifyingly, the disulfides containing the electron-withdrawing –NO2 and halides (–F, –Cl, and –Br) provided the sulfenylated products in moderate to very good yields too (Scheme 1, entries 3d, 3e, 3f, and 3g). The sulfenylation of the 6,7-dibromo benzoxazine substrate delivered the product in 79% yield (Scheme 1, entry 3m). Interestingly, the acetamide group (–NHCOCH3) in the disulfide and that in the benzoxazine substrate afforded the products in good yield (Scheme 1, entries 3h and 3l). Aliphatic disulfides (cyclohexyl and n-propyl) were also well tolerated in the reaction and yielded the products in 61% and 57% yields, respectively (Scheme 1, entries 3p and 3q).
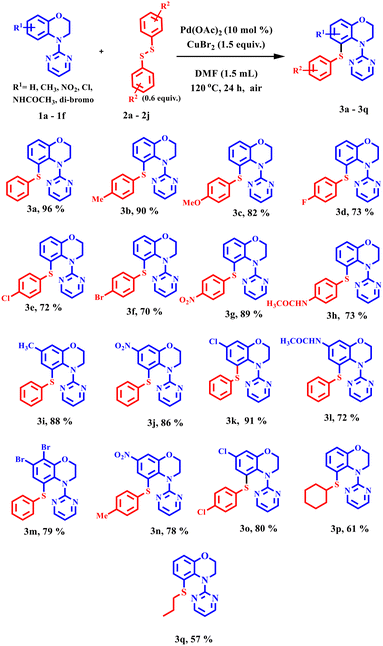 |
| Scheme 1 Scope of sulfenylation of 4-(pyrimidin-2-yl)-3,4-dihydro-2H-benzo[b][1,4]oxazines. | |
This protocol was further subjected to selenylation under these newly developed optimised conditions. The unsubstituted benzoxazine and unsubstituted diphenyl diselenide gave 91% product yield (Scheme 2, entry 5a). The electron-withdrawing groups (–NO2 and –Cl) at the 7-position of the benzomorpholine provided very good yields with diphenyl diselenide (Scheme 2, entries 5f and 5g). Interestingly, electron-withdrawing halogenated diphenyl diselenides (–F, –Cl, and –Br), too, provided the products in yields comparable to unsubstituted disulfide (Scheme 2, entries 5b, 5c, 5d, and 5a). A better product yield of 92% was obtained when the benzoxazine substrate having an electron-withdrawing –NO2 group at the 7-position was allowed to react with 4-bromophenyl diselenide (Scheme 2, entry 5h). These results demonstrated the importance of this newly developed protocol. The selenylation and sulfenylation were thus well tolerated in this reaction. The structure of compound 5h was also confirmed by crystallography (Fig. 2).
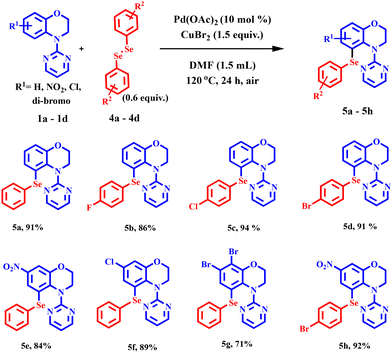 |
| Scheme 2 Scope of selenisation of 4-(pyrimidin-2-yl)-3,4-dihydro-2H-benzo[b][1,4]oxazines. | |
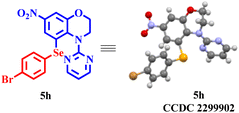 |
| Fig. 2 Crystal structure of compound 5h. | |
Gram-scale synthesis
We conducted a gram-scale sulfenylation of 4-(pyrimidin-2-yl)-3,4-dihydro-2H-benzo[b][1,4]oxazine 1a to illustrate the practicality of our developed methodology. Under the standard reaction conditions (Scheme 3), the reaction produced the desired sulfenylated product 3a effectively at 87% yield, showcasing the industrial and real-time feasibility of the protocol.
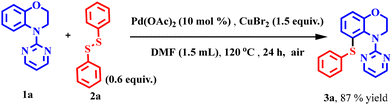 |
| Scheme 3 Gram-scale synthesis of 3a. | |
Late-stage functionalisation
In order to explore late-stage functionalisation, compound 5d was subjected to the Rosenmund–von Braun reaction22 in the presence of CuCN and in DMF, producing compound 6 in 79% yield (Scheme 4).
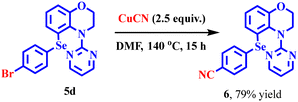 |
| Scheme 4 Rosenmund–von Braun reaction of 5dvia late-stage functionalisation. | |
Synthetic utility
In order to prove the synthetic utility of the scheme, the chalcogenation protocol was attempted on the 1-(pyrimidin-2-yl)indoline derivative, a bioactive heterocyclic moiety 7.20f We obtained the sulfenylated indoline 8 in 78% yield, while the selenylated product 9 was obtained in 88% yield under the standard reaction conditions (Scheme 5).
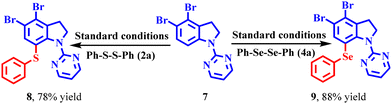 |
| Scheme 5 Scope of chalcogenation of 4,5-dibromo-1-(pyrimidin-2-yl)indoline. | |
Control experiments
To gain insight into the mechanism of the reaction, some control reactions were performed (Scheme 6). First, the reaction between 1a and 2a was performed in the absence of the catalyst Pd(OAc)2. No reaction occurred, indicating the indispensable role of the catalyst in this reaction (Scheme 6a). However, we got traces of the product 3a when the reaction was carried out in the absence of the oxidant (Scheme 6b) under the standard conditions. However, the addition of TEMPO, a radical scavenger, under the standard conditions afforded 60% product yield, suggesting the non-involvement of free radicals during the reaction (Scheme 6c).
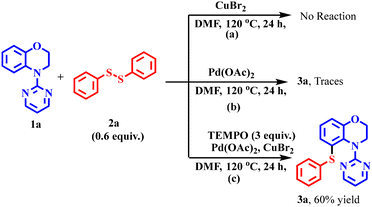 |
| Scheme 6 Preliminary mechanistic investigations. | |
H/D exchange and kinetic experiments
In order to investigate H/D scrambling, the reaction of 1a was carried out under the standard conditions in the absence of 2a and with equal volumes of DMF and D2O. The reactant 1a was recovered in 86% yield, albeit with 4% deuteration as 1a[D1] (Scheme 7a). But when the reaction was carried out in the absence of the palladium catalyst, no deuteration was detected (Scheme 7b). These results indicate the reversibility of the C–H palladation step and there is no involvement of the disulfides in the cleavage of the C–H bond. Furthermore, intermolecular competition reactions between 1a and 2b and 2g (para-tolyl and para-nitro diphenyl disulfides, respectively) in the same reaction vessel led to the formation of the respective products (3b and 3g) in 55% and 45% yields, respectively, displaying a product yield ratio of 1.33 (Scheme 7c), indicating the non-involvement of the C–H activation step in the rate-determining step.23
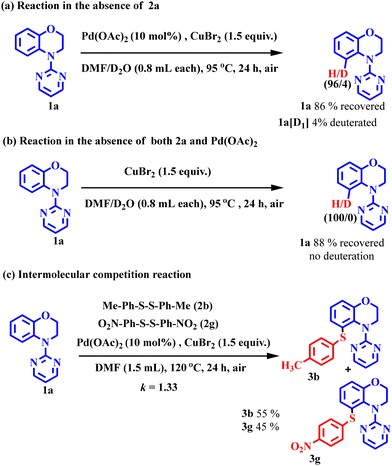 |
| Scheme 7 H/D exchange and kinetic experiment. | |
Plausible mechanism
Based upon these control experiments, kinetic studies, and the literature reports, we propose that the cycle begins with the formation of palladacycle 11, wherein the palladium acetate 10 ligates with benzoxazine moiety 1a (Scheme 8). This is a reversible reaction, selectively occurring at the ortho-position owing to the thermodynamic stability of the five-membered palladacycle intermediate 11.15,19,24 The intermediate further undergoes two pathways, one involving the nucleophilic substitution reaction with 2a to give the product 3a, and the second involving the oxidative addition of 2a into the intermediate 11 to give high-valent palladium(IV) species 12. The reductive elimination of 12 gives the product 3a along with the palladium complex 13. This complex 13 is further captured by another mole of the reactant 1a, to give divalent palladium complex 14. The reductive elimination of the latter leads to the product 3a, and palladium(I) species 15 are generated, which are oxidised to the active species 10 and the cycle continues. Copper bromide may have played a dual role, acting as a Lewis acid to facilitate the oxidative addition of 2a to intermediate 11 and/or acting as an oxidant to regenerate the palladium catalytic system 10. This may be the plausible reason for the use of 1.5 equiv. of CuBr2 during the reaction.15,25
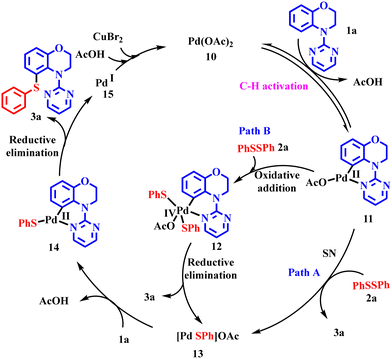 |
| Scheme 8 Plausible reaction mechanism for regioselective chalcogenation. | |
Conclusion
In conclusion, we have reported herein the palladium-catalysed chalcogenation of benzoxazine derivatives with disulfides and diselenides. The chalcogenated compounds were obtained in good to excellent yields due to the nitrogen atom's metal-directing ability. The newly developed protocol was compatible with a large number of diphenyl disulfides and diphenyl diselenides, exhibiting excellent substrate scope and high functional group tolerance. The chalcogenation of the dibromo-indoline derivative, a bioactive natural product, was successfully carried out using this established protocol. Furthermore, late-stage functionalisation with the help of the Rosenmund–von Braun reaction was executed and gram-scale synthesis had been successfully established using this protocol. All these regioselective products were formed via the five-membered, thermodynamically stable palladacycle.
Data availability
The authors confirm that data supporting the findings of the study are available within the article and its ESI.† Crystallographic data for compound 5h have been deposited at the CCDC under [CCDC 2299902] and can be obtained from https://www.ccdc.cam.ac.uk/.
Conflicts of interest
There are no conflicts to declare.
Acknowledgements
We express gratitude to the University of Delhi's IoE (Institute of Eminence) grant 2023-24 for providing financial assistance for this work. We also thank the University Science Instrumentation Centre (USIC), University of Delhi, for their instrumental facilities. Monika acknowledges the University of Delhi for granting her Research Fellowship, while Sandeep Kumar extends thanks to the University Grant Commission for awarding him a Senior Research Fellowship.
References
-
(a) X. Huang, Y. R. Zhang, X. S. Li, D. C. Xu and J. W. Xie, Tetrahedron Lett., 2013, 54, 5857 CrossRef CAS;
(b) B. Achari, S. B. Mandal, P. K. Dutta and C. Chowdhury, Synlett, 2004, 2449 CAS;
(c) F. A. Macias, D. Marin, A. Oliveros-Bastidas and J. M. G. Molinillo, Nat. Prod. Rep., 2009, 26, 478 RSC.
-
R. Kuang and J. Guo, WO Patent Appl, 2012, 136080 Search PubMed.
- D. S. La, J. Belzile, J. V. Bready, A. Coxon, T. DeMelfi, N. Doerr, J. Estrada, J. C. Flynn, S. R. Flynn, R. F. Graceffa, S. P. Harriman, J. F. Larrow, A. M. Long, M. W. Martin, M. J. Morrison, V. F. Patel, P. M. Roveto, L. Wang, M. M. Weiss, D. A. Whittington, Y. Teffera, Z. Zhao, A. J. Polverino and J.-C. Harmange, J. Med. Chem., 2008, 51, 1695 CrossRef CAS PubMed.
-
(a) K. M. H. Nguyen, L. Schwendimann, P. Gressens and M. Largeron, Org. Biomol. Chem., 2015, 13, 3749 RSC;
(b)
D. H. Miles, K. O. Petrovna, S. Naser, S. S. Yurjevich, E. A. Goun and S. V. Michailovich, US Patent, 2003, 6649610 Search PubMed;
(c)
H. Tsutsui, M. Hori, I. Watanabe, K. Harada, J. Maruo, T. Morita, H. Ohtaka and T. Yamamoto, EP Patent Appl, 1996, 719766 Search PubMed;
(d)
G. R. Madhavan, J. Iqbal, D. Bhuniya, R. Chakrabarti and S. K. Das, WO Patent Appl, 2003, 03033481 Search PubMed;
(e)
P. Hoelscher, R. Jautelat, H. Rehwinkel, S. Jaroch, D. Suelzle, M. Hillmann, G. A. Burton and F. M. McDonald, WO Patent Appl, 2001, 0181324 Search PubMed;
(f)
G. A. Burton, H. Rehwinkel, S. Jaroch, P. Hoelscher, D. Suelzle, M. Hillmann and F. M. McDonald, WO Patent Appl, 2000, 0017173 Search PubMed;
(g) K. S. Brown and C. Djerassi, J. Am. Chem. Soc., 1964, 86, 2451 CrossRef CAS.
-
J. M. Cid-Nunez and A. A. Trabanco-Suarez, U.S. Patent, 2014, 8697689 Search PubMed.
- B. S. Reddy, Y. V. Reddy, P. S. Lakshumma, G. Narasimhulu, J. S. Yadav, B. Sridhar, P. P. Reddy and A. C. Kunwar, RSC Adv., 2012, 2, 10661 RSC.
-
(a)
T. Takeichi and N. Furukawa, Polymer Science: A Comprehensive Reference, Elsevier, Amsterdam, 2012 Search PubMed;
(b)
T. J. Dingemans, Polymer Science: A Comprehensive Reference, Elsevier, 2012 Search PubMed.
-
P. Campaner, D. D'Amico, L. Longo, C. Stifani, A. Tarzia and S. Tiburzio, Study of a cardanol-based benzoxazine as reactive diluent and toughening agent of conventional benzoxazines, Handbook of Benzoxazine Resins, Elsevier, 2011 Search PubMed.
- Y. Liu and X. Chen, Tetrahedron, 2018, 74, 3691 CrossRef CAS.
-
(a)
E. J. Lenardão, C. Santi and L. Sancineto, New Frontiers in Organoselenium Compounds, Springer, Cham, 2018, pp. 157–183 CrossRef;
(b) E. Regis, L. D. O. Aguiar, P. Tuzimoto, E. Girotto, T. E. Frizon, A. G. D. Bo, E. Zapp, R. Marra, H. Gallardo and A. A. Vieira, Dyes Pigm., 2018, 157, 109 CrossRef CAS;
(c) G. C. Hoover and D. S. Seferos, Chem. Sci., 2019, 1, 9182 RSC;
(d) A. E. Baumann, D. A. Burns, B. Liu and V. S. Thoi, Commun. Chem., 2019, 2, 86 CrossRef.
-
(a) W. Ma, Y. Zhou, Y. Wang, B. Li, T. Zheng, Z. Cheng and R. Mei, Adv. Synth. Catal., 2022, 364, 3544 CrossRef CAS;
(b) T. K. Vats, A. Mishra and I. Deb, Adv. Synth. Catal., 2018, 360, 2291 CrossRef CAS;
(c) X. Rui, Y. Zhu, R. Dai, C. Huang, C. Wang, D. Si and J. Liu, Asian J. Org. Chem., 2021, 10, 793 CrossRef CAS.
- M. E. Cinar and T. Ozturk, Chem. Rev., 2015, 115, 3036 CrossRef CAS PubMed.
-
(a) S. Ortgies and A. Breder, ACS Catal., 2017, 7, 5828 CrossRef CAS;
(b) A. J. Kronemeijer, E. Gili, M. Shahid, J. Rivnay, A. Salleo, M. Heeney and H. Sirringhaus, Adv. Mater., 2012, 24, 1558 CrossRef CAS PubMed;
(c) S. Yang, J. Sun, P. He, X. Deng, Z. Wang, C. Hu, G. Ding and X. Xie, Chem. Mater., 2015, 27, 2004 CrossRef CAS;
(d) Z. Lou, P. Li and K. Han, Acc. Chem. Res., 2015, 48, 1358 CrossRef CAS PubMed;
(e) S. T. Manjare, Y. Kim and D. G. Churchill, Acc. Chem. Res., 2014, 47, 2985 CrossRef CAS PubMed.
-
(a) S. Prachayasittikul, R. Pingaew, A. Worachartcheewan, N. Sinthupoom, V. Prachayasittikul, S. Ruchirawat and V. Prachayasittikul, Mini-Rev. Med. Chem., 2017, 17, 869 CAS;
(b) M. A. Chiacchio, D. Iannazzo, R. Romeo, S. V. Giofrè and L. Legnani, Curr. Med. Chem., 2019, 26, 7166 CrossRef CAS PubMed;
(c) M. Albratty and H. A. Alhazmi, Arabian J. Chem., 2022, 15, 103846 CrossRef CAS;
(d) J. Rayadurgam, S. Sana, M. Sasikumar and Q. Gu, Org. Chem. Front., 2021, 8, 384 RSC;
(e)
M. Burger, Z. J. Ni, S. Pecchi, G. Atallah, S. Bartulis, K. Frazier and X. Xin, U.S. Patent, 2012, 8217035 Search PubMed;
(f)
D. Flubacher, P. Michel, M. C. Testa and R. Mose, U.S. Patent, 2016, 9452994 Search PubMed;
(g) J. V. Calienni, M. D. La Cruz, D. Flubacher, B. Gong, P. K. Kapa, P. H. Karpinski, H. Liu, P. Michel, R. Mose, M. C. Testa and L. M. Waykole, E.P., 2016, 3040333 (A1) Search PubMed.
- R. Qiu, V. P. Reddy, T. Iwasaki and N. Kambe, J. Org. Chem., 2015, 80, 367 CrossRef CAS PubMed.
- Y. He, S. Hou and J. Hu, Tetrahedron, 2022, 103, 132552 CrossRef CAS.
-
(a)
J. Tsuji, Palladium
reagents and catalysts: new perspectives for the 21st century, John Wiley & Sons, 2006 Search PubMed;
(b) L.-N. Guo, X.-H. Duan and Y.-M. Liang, Acc. Chem. Res., 2011, 44, 111 CrossRef CAS PubMed;
(c) X.-F. Wu, H. Neumann and M. Beller, Chem. Rev., 2013, 113, 1 CrossRef CAS PubMed;
(d) J. Ye and S. Ma, Acc. Chem. Res., 2014, 47, 989 CrossRef CAS PubMed;
(e) G. Yin, X. Mu and G. Liu, Acc. Chem. Res., 2016, 49, 2413 CrossRef CAS PubMed;
(f) A. Düfert and D. B. Werz, Chem. – Eur. J., 2016, 22, 16718 CrossRef PubMed;
(g) D. Wang, A. B. Weinstein, P. B. White and S. S. Stahl, Chem. Rev., 2018, 118, 2636 CrossRef CAS PubMed.
- C. Hedberg, K. S. Jessen, R. F. Hansson, M. Heuckendorff and H. H. Jensen, Org. Lett., 2020, 22, 7068 CrossRef CAS PubMed.
- J. Li, S. Yang, W. Wu and H. Jiang, Org. Chem. Front., 2020, 7, 1395 RSC.
-
(a) X. Chen, X. S. Hao, C. E. Goodhue and J. Q. Yu, J. Am. Chem. Soc., 2006, 128, 6790 CrossRef CAS PubMed;
(b) P. Saravanan and P. Anbarasan, Org. Lett., 2014, 16, 848 CrossRef CAS PubMed;
(c) M. Iwasaki, M. Iyanaga, Y. Tsuchiya, Y. Nishimura and Y. Nishihara, Eur. J. Chem., 2014, 20, 2459 CrossRef CAS PubMed;
(d) M. Iwasaki, W. Kaneshika, Y. Tsuchiya, K. Nakajima and Y. Nishihara, J. Org. Chem., 2014, 79, 11330 CrossRef CAS PubMed;
(e) Y. He, S. Hou and J. Hu, Tetrahedron, 2022, 103, 132552 CrossRef CAS;
(f) P. Gandeepan, J. Koeller and L. Ackermann, ACS Catal., 2016, 7, 1030 CrossRef.
-
(a) M. Gupta, S. Kumar, P. Kumar, A. K. Singh, V. Bahadur and B. K. Singh, ChemistrySelect, 2019, 4, 13992 CrossRef CAS;
(b) C. Chen, Y. Pan, H. Zhao, X. Xu, Z. Luo, L. Cao and L. Xu, Org. Lett., 2018, 20, 6799 CrossRef CAS PubMed.
- F. Koelsch and A. G. Whitney, J. Org. Chem., 1941, 6, 795 CrossRef.
-
(a) J. H. Chu, S. T. Chen, M. F. Chiang and M. J. Wu, Organometallics, 2015, 34, 953 CrossRef CAS;
(b) E. M. Simmons and J. F. Hartwig, Angew. Chem., Int. Ed., 2012, 51, 3066 CrossRef CAS PubMed.
- I. P. Beletskaya and V. P. Ananikov, Chem. Rev., 2022, 122, 16110 CrossRef CAS PubMed.
- J. Liu, Z. Xue, Z. Zeng, Y. X. Chen and G. Chen, Adv. Synth. Catal., 2016, 358, 3694 CrossRef CAS.
|
This journal is © The Royal Society of Chemistry 2024 |
Click here to see how this site uses Cookies. View our privacy policy here.