DOI:
10.1039/D3QI01824E
(Research Article)
Inorg. Chem. Front., 2024,
11, 133-141
Boosting the efficiency of deep-red Ir(III) complexes by modulating nitrogen atoms for high-performance OLEDs†
Received
9th September 2023
, Accepted 27th October 2023
First published on 2nd November 2023
Abstract
Constructing deep-red Ir(III) phosphors featuring sufficient luminous efficiency is highly desirable but remains challenging owing to their severe nonradiative decay. Herein, two structurally simple Ir(III) complexes, namely Ir(iqbt)2IPO and Ir(qabt)2IPO, with cyclometalated ligands modulated by nitrogen atoms were developed. Both complexes showed efficient deep-red phosphorescence with the desired emission peaked at approximately 680 nm and shoulder peaks at 735 nm. Complex Ir(qabt)2IPO with a high nitrogen atom doping ratio exhibited a much higher emission intensity accompanied by a better PLQY of 21.4% relative to that of Ir(iqbt)2IPO (16.3%). Analysis of the excited electronic structures of both complexes indicated that such high emission efficiency could stem from the augmentation of the MLCT character. Consequently, Ir(qabt)2IPO based OLED displayed more than twofold improvement in the maximum external quantum efficiency, exceeding that of Ir(iqbt)2IPO and affording the deep-red electroluminescence with CIE coordinates at (0.72, 0.27). Therefore, designing cyclometalated ligands by introducing extra nitrogen atoms provides an effective strategy for exploring deep-red Ir(III) complexes and OLEDs.
Introduction
As promising energy-saving lighting and display techniques in our daily lives, organic light-emitting diodes (OLEDs) have attracted sustained attention from academics and business fields.1–3 Motivated by this, there is an increasing demand for developing robust emitting materials to achieve high-performance OLEDs.4–7 Among all the solid-state emitters employed in devices, phosphorescent transition metal complexes featuring a large spin–orbital coupling (SOC) effect are proposed to replace the fluorescent ones because of their capacity to harvest all electrogenerated exciton. Well-investigated phosphorescent Ir(III) complexes have come to the fore as promising emitters, which possess high luminous efficiency, excellent thermal and electrochemical stabilities, and enabled control of their excited-state properties.8–12 To date, efficient devices composed of Ir(III) complexes with emissions located in the blue to red region have been successfully explored and systematically studied.13–17 However, it remains challenging for researchers to synthesize long-wavelength Ir(III) complexes, such as deep-red phosphorescence.
Bright and efficient deep-red-emitting Ir(III) complexes with maximum or large part of their emissions beyond 650 nm are still scarce despite the great potential for applications in night vision, bioimaging, and photodynamic therapy. Twofold reasons are responsible for hindering their development of a high PLQY. First, according to the energy-gap laws, deep-red phosphors are generally subject to more severe nonradiative deactivation and low radiative deactivation caused by their narrow bandgap.18–20 Second, the planar aromatic units employed to push the emission toward the deep-red region are favorable for face-to-face π–π stacking, resulting in unwanted luminescence quenching in the solid state.21 To inhibit the performance losses caused by the harmful nonradiative transitions, the prevalent designed strategy is to reinforce the rigidity and conjugation of organic ligands for achieving low-energy emission.22–24 However, this strategy not only worsens emission quenching triggered by π–π interaction, but also weakens the participation of the d orbital of the Ir(III) atom and the metal-to-ligand charge-transfer (MLCT) character, thus bringing about severe PLQY and device efficiency drops. In addition, anchoring electron-rich ancillary ligands on the molecular structure is another effective strategy for synthesizing high-efficiency Ir(III) complexes.25–27 Despite some progress in molecular design, there are still relatively few Ir(III) complexes achieving deep-red emission as well as high photoluminescence and electroluminescence (EL) performance.28–31 Therefore, a simple molecular design strategy is urgently needed for deep-red emitters.
With the purpose of designing efficient deep-red Ir(III) complexes by a simple method, a new cyclometalated ligand was synthesized by introducing an extra nitrogen atom into a common ligand 1-(benzo[b]thiophen-2-yl)-isoquinoline unit, as it is known to emit red and NIR emission paired with Ir(III) atom and other ancillary ligand.32,33 The ancillary ligand including a bulky electron-deficient unit was employed to increase the steric hindrance and improve electron injection and transportation ability. Two emitters, Ir(iqbt)2IPO and Ir(qabt)2IPO, were found to emit intense deep-red phosphorescence with peaks located at about 680 nm. With a similar emission wavelength, complex Ir(qabt)2IPO exhibits much stronger emission and better PLQY of 21.4% compared with 16.3% for Ir(iqbt)2IPO in the solution. As a result, Ir(iqbt)2IPO supported its deep-red OLED with the desired emission peaking at 680 nm and a maximum external quantum efficiency (EQE) of 3.29%. The device performance can be improved to 8.43% of the maximum EQE in devices based on Ir(qabt)2IPO. Moreover, the double emitting layer OLED using Ir(qabt)2IPO could achieve a maximum EQE of 9.12% and CIE values of (0.72, 0.27). These results propose a simple method for constructing high-performance deep-red Ir(III) complexes and OLEDs.
Results and discussion
Synthesis, characterization, and thermal stability
The synthetic routes of designed complexes with the type of Ir(C^N)2(LX) composed of 1-(benzo[b]thiophen-2-yl)isoquinoline (iqbt) and 4-(benzo[b]thiophen-2-yl)quinazoline (qabt) as cyclometalated ligands are summarized in Scheme 1. The iqbt ligand is well known to prepare Ir(III) complexes with red and NIR luminescence. The ligand qabt with higher nitrogen doping was designed to improve the emission efficiency. Both iqbt and qabt ligands were produced via the Suzuki coupling reaction and then reacted with IrCl3·nH2O to obtain the dimers using the method described by Nonoyama et al.34 The dimers were then converted into complexes Ir(iqbt)2IPO and Ir(qabt)2IPO by reacting with the ancillary ligand 1-(4-(diphenylphosphoryl)phenyl)-3-methyl-1H-imidazol-3-ium (IPO) with the isolated yields of 38% and 15%. After the purification, two Ir(III) emitters were structurally identified by mass spectrometry, elemental analyses, and nuclear magnetic resonance (NMR) spectroscopy (Fig. S1–S10 ESI†). Thermogravimetric analysis (TGA) and differential scanning calorimetry (DSC) measurements were carried out to determine their thermal stabilities, which are essential data for fabricating OLEDs. As shown in Fig. S11 (ESI†), Ir(iqbt)2IPO and Ir(qabt)2IPO exhibit good stabilities with decomposition temperatures of 333 °C and 395 °C at 5% degradation, respectively. Meanwhile, high glass transition temperatures (Tg) are observed at 222 °C and 221 °C for Ir(iqbt)2IPO and Ir(qabt)2IPO, suggesting that both complexes can be employed in OLEDs via the thermal evaporation method.
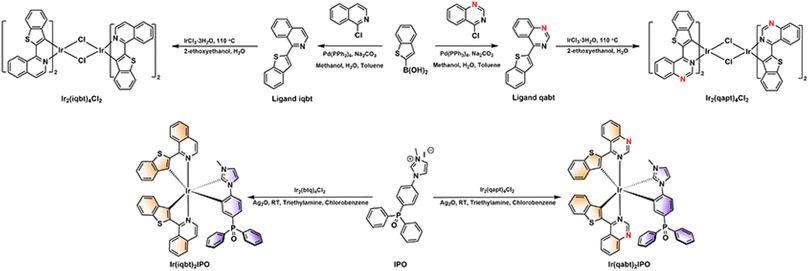 |
| Scheme 1 The synthesis route and molecular structures of Ir(iqbt)2IPO and Ir(qabt)2IPO. | |
Single-crystal structure analysis
The molecular configuration and packing modes of the complex Ir(iqbt)2IPO were confirmed by X-ray diffraction analysis. The detailed crystal parameters are listed in Tables S1 and S2 (ESI†). As shown in Fig. 1A, three organic ligands coordinated with the iridium center comprise a typical octahedral geometry with a six-coordinated structure. The isoquinoline moiety is almost co-planar to the benzothiophene moiety with the corresponding dihedral angle of 5.0(8) Å, which could be induced by the intramolecular interaction of C–H⋯S in a single cyclometalated ligand. These hydrogen-bonding interactions with the distances of 2.39(2) and 2.42(9) Å were observed in both cyclometalated ligand planes, which can rigidify the molecular conformation and restrain structural relaxation. As shown in Fig. 1B, numerous intermolecular interactions can be observed from the crystal packing diagram. The multiple C–H⋯π interactions between the neighboring emitters with distances of ca. 2.49–3.27 Å probably help to restrict the molecular motions, and thus decrease the nonradiative transition. Owing to the large steric hindrance stemming from the distortion of the ancillary ligand, no directly close π⋯π stacking from adjacent molecules is observed, which can effectively suppress intermolecular stacking and the quenching effect.
 |
| Fig. 1 Single-crystal (A) molecular configuration with 30% probability level and (B) intermolecular/intramolecular interactions (CCDC 2155915†). | |
Photophysical properties
To thoroughly study the influence of introducing nitrogen atoms, the photophysical investigation of Ir(iqbt)2IPO and Ir(qabt)2IPO was performed by spectroscopy measurements and summarized in Table 1. As depicted in Fig. 2A, both complexes display intense electronic absorption bands below 400 nm, corresponding to the π–π* transition of cyclometalated ligands. The overlapping features in the absorption spectra at 400–600 nm are derived from spin-allowed and spin-forbidden intraligand charge-transfer (ILCT), ligand–ligand charge-transfer (LLCT), and MLCT.35 Obviously, the introduction of nitrogen atoms into Ir(qabt)2IPO accounts for a slight red-shift in the longest-wavelength absorption at 450–600 nm relative to that of Ir(iqbt)2IPO. The energy gaps (Eg) for Ir(iqbt)2IPO and Ir(qabt)2IPO were calculated to be 2.01 and 1.87 eV according to the UV-vis absorption spectra, respectively. The PL spectra of dilute solutions were also affected in the increase of nitrogen atoms. As shown in Fig. 2B, the complex Ir(iqbt)2IPO exhibits intense deep-red phosphorescence with maximum emission peaks at 682 nm and a shoulder emission at 735 nm. The significant vibronic fine structure was observed for Ir(iqbt)2IPO, indicating the predominant 3LC character in the excited state. The emission spectrum for Ir(qabt)2IPO is similar but has less vibrational characteristics with a peak at 680 nm, demonstrating that its emission mainly originates from the 3MLCT character. The Stokes shift between λmax for the absorption and emission spectra of Ir(iqbt)2IPO and Ir(qabt)2IPO were 161 and 133 nm, respectively, whereas a significantly larger shift was observed for Ir(iqbt)2IPO. The significant difference in the Stokes shift can be attributed to the different transitions in the excited state induced by the number of nitrogen atoms in the main ligand.36 Moreover, the complex Ir(qabt)2IPO shows a much stronger emission with a PLQY of 21.4% than that of Ir(iqbt)2IPO (PLQY: 16.3%), which indicates that extra nitrogen influences the emission efficiency. Furthermore, the PL lifetimes were determined to be 1.06 μs for Ir(iqbt)2IPO and 1.56 μs for Ir(qabt)2IPO. The lifetimes in microseconds substantiate their phosphorescent characteristics. The radiative decay rate constants (kr) were determined to be 1.54 × 105 s−1 and 1.39 × 105 s−1 for Ir(iqbt)2IPO and Ir(qabt)2IPO, while the nonradiative decay rate constants (knr) were calculated to be 7.90 × 105 s−1 and 5.10 × 105 s−1 for Ir(iqbt)2IPO and Ir(qabt)2IPO. Meanwhile, the PL spectra recorded in pure films and solid states exhibited an obvious red-shift of the emission peaks at 717 and 698 nm for Ir(iqbt)2IPO as well as 737 and 742 nm for Ir(qabt)2IPO, as shown in Fig. S12.† The emission spectra in the degassed 2-methyltetrahydrofuran frozen matrix measured at 77 K (Fig. S13†) showed an emission with a maximum intensity at 663 nm for Ir(iqbt)2IPO and 657 nm for Ir(qabt)2IPO. Both complexes exhibited an obvious rigidochromism effect.
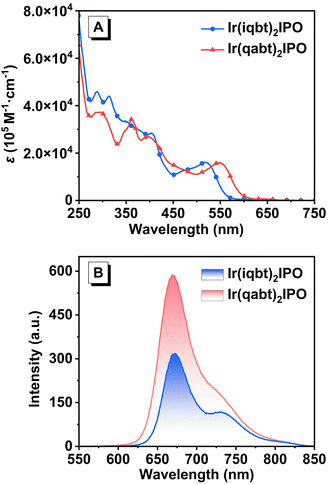 |
| Fig. 2 (A) UV-vis absorption and (B) PL spectra of the Ir(iqbt)2IPO and Ir(qabt)2IPO recorded in degassed dichloromethane (1 × 10−5 M and λex = 520 nm) at room temperature. | |
Table 1 Photophysical properties and electrochemical data of Ir(iqbt)2IPO and Ir(qabt)2IPO complexes
Complex |
Absorptiona |
Emissiona |
Emissionb |
Electrochemistryc |
λ
abs (nm) |
E
g
|
λ
em (nm) |
PLQY |
τ (μs) |
k
r (105 s−1) |
k
nr (105 s−1) |
λ
em (nm) |
HOMO (eV) |
LUMO (eV) |
In CH2Cl2 solution at room temperature, concentration = 1.0 × 10−5 M.
In a CH2Cl2 frozen matrix at 77 K.
From the onset of oxidation and reduction potentials according to the equation of EHOMO = −(Eox + 4.8) eV and ELUMO = −(Ered + 4.8) eV.
|
Ir(iqbt)2IPO
|
312, 403, 521 |
2.01 |
682, 735 |
0.163 |
1.06 |
1.54 |
7.90 |
663 |
−5.06 |
−2.88 |
Ir(qabt)2IPO
|
294, 363, 547 |
1.87 |
680 |
0.214 |
1.54 |
1.39 |
5.10 |
657 |
−5.32 |
−3.24 |
Computational calculation
To clarify the influence of the incorporation of extra nitrogen atoms on the excited state and optical transitions of two deep-emitting Ir(III) phosphors, theoretical investigations based on their optimized lowest triplet excited state (T1) configuration were carried out by time-dependent density functional theory (TD-DFT).37 In addition, unpaired electrons occupied orbitals of T1 states based on the unrestricted open-shell Kohn–Sham (UKS) method. Cartesian coordinates of T1-optimized structures are given in Table S3.† The lowest unoccupied molecular orbitals (LUMOs) of Ir(iqbt)2IPO and Ir(qabt)2IPO are dispersed on one of the main ligands and also slightly expand to the Ir(III) atom. Meanwhile, the highest occupied molecular orbitals (HOMOs) are basically localized at the Ir(III) atom and part of cyclometalated ligand, while there is only a tiny contribution from the ancillary ligand into HOMOs and LUMOs, as shown in Fig. 3 and Fig. S14.† Similar transition components consisting of HOMO to LUMO and HOMO − 1 to LUMO transition were observed in T1 states for Ir(iqbt)2IPO and Ir(qabt)2IPO, which feature a mixed MLCT, LLCT, and LC character. Notably, the 3MLCT character of Ir(qabt)2IPO (16.45%) is more pronounced than that of Ir(iqbt)2IPO (13.72%), which can account for the relatively high PLQY value of Ir(qabt)2IPO. The HOMO and LUMO energy levels were also calculated, which turned out to be −4.60/–1.91 and −4.94/–2.20 eV for Ir(iqbt)2IPO and Ir(qabt)2IPO, respectively, as listed in Table S4.† The frontier molecular orbital energy levels of Ir(qabt)2IPO are obviously deeper than that of Ir(iqbt)2IPO, indicating the improved electron-withdraw ability as the number of nitrogen atoms increases. Meanwhile, the energy levels of unpaired electrons occupied orbitals display the same trend, as shown in Fig. 3B.
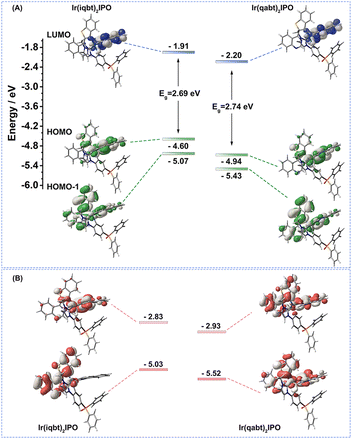 |
| Fig. 3 (A) Theoretical contours and energy levels of the HOMO, HOMO − 1, and LUMO based on their optimized T1 geometry. (B) Unpaired electrons occupied orbitals of T1 states. | |
Electrochemical properties
The electrochemical characterization of newly designed Ir(III) complexes were explored by cyclic voltammetry (CV) experiments. As demonstrated in Fig. 4 and Table 1, two complexes show clear oxidation peaks with the onset oxidation potentials (Eox) of 0.26 and 0.52 V for Ir(iqbt)2IPO and Ir(qabt)2IPO, respectively, corresponding to the oxidation of the metal iridium. The Eox of Ir(qabt)2IPO is more positive than that of Ir(iqbt)2IPO. The obvious onset reduction potentials (Ere) were detected for Ir(iqbt)2IPO and Ir(qabt)2IPO as −1.92 and −1.56 V, respectively. Furthermore, the measured HOMO and LUMO energy levels are −5.06 and −2.88 eV for Ir(iqbt)2IPO, and −5.32 and −3.24 eV for Ir(qabt)2IPO, respectively. These results demonstrate that an extra nitrogen atom can greatly improve the electron-withdrawing ability, and modulated the electrochemical properties and HOMO/LUMO energy levels.
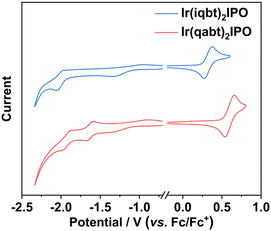 |
| Fig. 4 Cyclic voltammograms of Ir(iqbt)2IPO and Ir(qabt)2IPO in solutions (1 × 10−3 mol L−1) at the scan rates from 100 mV s−1. | |
Electroluminescent performance
To evaluate the superiority of Ir(iqbt)2IPO and Ir(qabt)2IPO for optoelectronic devices, the thermally evaporated OLEDs were fabricated with a configuration of ITO/MoO3 (3 nm)/TAPC (35 nm)/TCTA (5 nm)/CBP (90%): Ir(III) emitter (10%) (20 nm)/TmPyPB (50 nm)/LiF (1 nm)/Al (100 nm), in which LiF, TmPyPB, CBP, TCTA, TAPC, and MoO3 were employed as electron-injecting material, electron-transporting material, host material, electron-blocking material, hole-transporting material, and hole-injection material, respectively. To improve the EL performance of OLEDs, we chose the CBP as the host, which has a high T1 energy level of 2.6 eV for blocking the excitons within the Ir(III) complexes. Moreover, CBP is a superior host with a favorable electron trapping character with a low injection barrier of −0.2 eV. The devices that comprised complexes Ir(iqbt)2IPO and Ir(qabt)2IPO in the CBP host were named A1 and A2, respectively. The energy-level diagram and chemical structure of the selected materials are summarized in Fig. 5. The EL performance characteristics are depicted in Fig. 6, while the summary of the EL efficiencies data is listed in Table 2.
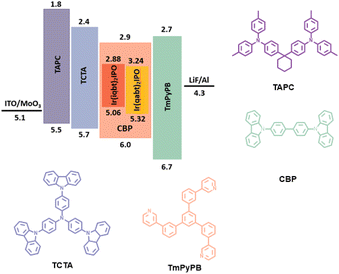 |
| Fig. 5 Energy level diagrams and functional materials structures of fabricated OLEDs. | |
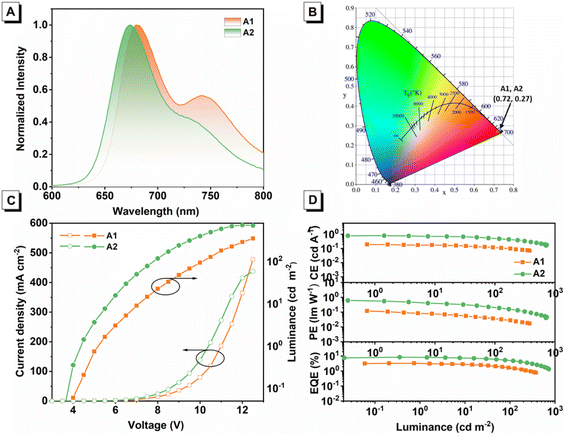 |
| Fig. 6 (A) EL spectra of both devices. (B) CIE coordinates. (C) Current density–voltage–luminance (JVL) characteristics. (D) CE, PE, EQE vs. luminance. | |
Table 2 Key EL performances of deep-red devices based on Ir(iqbt)2IPO and Ir(qabt)2IPO
Device |
V
turn–on a (V) |
L
max(cd m−2) |
CEb (cd A−1) |
PEb (lm W−1) |
EQEb (%) |
λ
max c (nm) |
CIEc |
V
turn-on: turn-on voltage recorded at a luminance of 1 cd m−2.
Maximum EL efficiency and EL efficiency at 100 cd m−2.
Measured at 6 V.
|
A1 |
5.1 |
375 |
0.18 |
0.11 |
3.29/1.62 |
680/743 |
(0.72, 0.27) |
A2 |
4.3 |
727 |
0.79 |
0.61 |
8.43/5.04 |
675/727 |
(0.72, 0.27) |
B1 |
5.3 |
322 |
0.15 |
0.08 |
2.73/1.58 |
681/742 |
(0.72, 0.27) |
B2 |
4.2 |
930 |
0.89 |
0.62 |
9.12/6.45 |
675/727 |
(0.72, 0.27) |
The EL spectra of devices A1 and A2 shown in Fig. 6A well resemble the corresponding PL profiles in their solutions, suggesting that emissions in two devices exactly originated from guest emitters Ir(iqbt)2IPO and Ir(qabt)2IPO with efficient energy transfer and carrier recombination with the emitting layer. The emission wavelength displays a small difference in the peak position between the solution and doped CBP films, which can be affected by the polarity of the environment and weak intermolecular interactions between the adjacent phosphors and host materials.38 After the turn-on voltage (Von) of 5.1 and 4.3 V, Ir(iqbt)2IPO and Ir(qabt)2IPO-based devices exhibited intense deep-red EL with the maximum peaks at 680 nm and 675 nm, respectively, corresponding to the same CIE coordinate of (0.72, 0.27). As for device A1, the EL performance of a maximum EQE, a maximum current efficiency (CE), and a maximum power efficiency (PE) of 3.29%, 0.18 cd A−1, and 0.11 lm W−1 were achieved; the maximum EL intensity was determined to be 375 cd m−2. Introducing nitrogen atoms into the Ir(III) complexes leads to better PLQY, which is critical for improving EL performance. Therefore, device A2 afforded the higher EL performance with EQE, CE, and PE of 8.43%, 0.79 cd A−1, and 0.61 lm W−1, respectively, along with a maximum luminescence of 727 cd m−2. Notably, the EQE value of device A2 increased and it was 2.5 times that of device A1.
Moreover, the double-EML OLEDs were prepared to further optimize the EL performance. The double-EMLs devices based on Ir(iqbt)2IPO and Ir(qabt)2IPO emitter were fabricated with a structure of ITO/MoO3 (3 nm)/TAPC (40 nm)/Ir(III) complex (10 wt%): TCTA (10 nm)/Ir complex (10 wt%): 26DCzPPy (10 nm)/TmPyPB (60 nm)/LiF (1 nm)/Al (100 nm), namely B1 and B2, respectively. Diagrams of the energy levels and EL performance characteristics are shown in Fig. S15† and Table 2. In double-EML devices, the 26DCzPPy and TCTA were chosen as the host material to ensure the LUMO and HOMO levels of Ir(iqbt)2IPO and Ir(qabt)2IPO fully contained in those of 26DCzPPy and TCTA. In addition, as shown in Fig. S15,† the gradual changes in the LUMO and HOMO energy levels are favorable for carrier transport and injection, thus broadening the exciton recombination zone for high device performance.39,40 The EL spectra of double-EML devices are similar to those of single-EML ones with the CIE of (0.72, 0.27). More importantly, double-EML devices based on Ir(qabt)2IPO displayed improved EL performance with a maximum EQE of 9.12%. In addition, the poor EL performance of device B1 can be attributed to the improved turn-on voltage from 5.1 to 5.3 V.
Conclusions
By utilizing the accessible and structurally simple cyclometalated ligands, two newly designed deep-red-emitting complexes Ir(iqbt)2IPO and Ir(qabt)2IPO, were constructed and investigated. Their emission performance can be regulated by heteroatomic engineering. Benefiting from the introduction of the nitrogen atom, the complex Ir(qabt)2IPO exhibited increased 3MLCT character in emission, which is favorable for the increases in emission efficiency in both photoluminescence and electroluminescence processes. Their evaporation OLEDs can achieve deep-red electroluminescence at 680 nm and 675 nm with the maximum EQEs of 3.29% and 8.43% for Ir(iqbt)2IPO and Ir(qabt)2IPO, respectively. The study demonstrates that ingeniously regulating the heteroatomic atom in Ir(III) phosphors is an effective solution to achieve deep-red-emitting emitters and their highly efficient OLEDs.
Experimental section
General synthesis of the cyclometalated and ancillary ligands
Mixture of toluene and ethanol (2
:
1, v
:
v) (30 mL), Pd(PPh3)4 (0.04 equiv.), benzo[b]thiophen-2-boric acid (1.0 equiv.), or 4-chloro-quinazoline (1.0 equiv.), and sodium carbonate aqueous solution (2 M, 20 mL) was stirred at 85 °C for 24 h under a nitrogen atmosphere. After cooling to room temperature, the mixture was extracted with CH2Cl2 and water three times. The organic layer was dealt with anhydrous Na2SO4. The solvent was separated under vacuum and the residual was purified with column chromatography on silica gel to give the desired compounds.
Ligand iqbt (yield, 36.0%).
1H NMR (CDCl3, 500 MHz): δδ (ppm) 8.62 (t, J = 4.0 Hz, 2H), 7.94 7.88 (m, 3H), 7.86 (s, 1H), 7.74 (td, J1 = 7.0 Hz, J2 = 1.0 Hz, 1H), 7.68–7.65 (m, 2H), 7.45–7.39 (m, 2H).
Ligand qabt (yield, 49.4%).
1H NMR (CDCl3, 500 MHz): δ (ppm) 9.36 (s, 1H), 8.64 (d, J = 8.5 Hz, 1H), 8.21 (d, J = 8.5 Hz, 1H), 8.13 (s, 1H), 8.01–7.94 (m, 3H), 7.76 (t, J = 5.5 Hz, 1H), 7.49–7.44 (m, 2H).
Ligand IPO (Yield, 70%).
The reaction was prepared following the literature method. 1H NMR (CDCl3, 500 MHz): δ (ppm) 10.57 (s, 1H), 8.01–7.92 (m, 2H), 7.97 (s, 1H), 7.63–7.56 (m, 6H), 7.46 (td, J1 = 7.5 Hz, J2 = 2.5 Hz, 4H), 4.21 (s, 3H).
General synthesis for designed Ir(III) complexes
Under a nitrogen atmosphere, the mixture solution of ethylene glycol ether and distilled water (3
:
1, v
:
v) (24 mL), a cyclometalated ligand (2.2 equiv.), and IrCl3·3H2O (1.0 equiv.) was heated to 110 °C and stirred for 24 hours. After cooling to room temperature, the precipitated solids were filtered and washed with water, anhydrous ethanol, and ether in sequence, and vacuum dried to constant weight at 100 °C. The IPO ligand (1.03 equiv.), silver oxide (1.02 equiv.), and triethylamine (0.4 mL) were resolved in chlorobenzene (15 mL) and stirred for 15 min under a nitrogen atmosphere. Then, the corresponding μ-chloro-bridged dimer (0.46 equiv.) and extra silver oxide (2.33 equiv.) were added and heated to 100 °C. After cooling to ambient temperature, saturated sodium chloride aqueous solution and dichloromethane were added to extract three times. The crude product was then purified by column chromatography on a silica gel column.
Complex Ir(iqbt)2IPO (Yield, 38.0%).
1H NMR (DMSO-d6, 500 MHz): δ (ppm) 9.06 (d, J = 8.5 Hz, 1H), 9.01 (d, J = 8.5 Hz, 1H), 8.26 (d, 7.0 Hz, 1H), 8.01–7.96 (m, 3H), 7.92–7.90 (m, 3H), 7.88–7.81 (m, 4H), 7.69 (d, J = 8.0 Hz, 1H), 7.28 (td, J1 = 16.0 Hz, J2 = 5.5 Hz, 5H), 7.32–7.26 (m, 5H), 7.16–7.06 (m, 6H), 6.96–6.93 (m, 4H), 6.70 (q, J = 8.0 Hz, 1H), 6.62 (d, J = 10.5 Hz, 1H), 6.53 (q, J = 8.0 Hz, 1H), 6.40 (d, J = 8.0 Hz, 1H), 5.99 (d, J = 8.0 Hz, 1H), 2.98 (s, 3H). 13C NMR (125 MHz, d6-DMSO) δ (ppm) 194.14, 190.78, 174.37, 172.52, 162.77, 160.80, 154.58, 153.35, 153.30, 152.72, 152.67, 150.11, 150.04, 149.69, 149.53, 148.74, 148.67, 144.72, 144.62, 137.39, 137.35, 135.30, 135.15, 135.11, 130.43, 130.41, 127.69, 127.61, 127.48, 127.37, 125.97, 125.92, 125.13, 125.05, 125.02, 124.96, 124.81, 124.72, 124.66, 124.00, 123.56, 123.54, 122.74, 122.69, 120.83, 120.58, 114.16, 113.88, 111.72, 109.24, 109.10, 36.73 MS m/z: [M]+ calcd for C56H38IrN4OPS2, 1070.2; found, 1070.2. Elemental analysis calcd for C56H38IrN4OPS2: C, 62.85; H, 3.58; N, 5.24. Found: C, 62.02; N, 5.16; H, 3.43.
Complex Ir(qabt)2IPO (Yield, 15.0%).
1H NMR (DMSO-d6, 500 MHz): δ (ppm) 8.90–8.88 (m, 1H), 8.85 (d, J = 8.5 Hz, 1H), 8.46 (d, J = 16.0 Hz, 2H), 8.27 (d, J = 2.0 Hz, 1H), 8.09–8.01 (m, 8 Hz), 7.99–7.96 (m 1H), 7.85 (d, J = 8.5 Hz, 1H), 7.79 (d, J = 8.0 Hz 1H), 7.36 (d, J = 2.0 Hz, 1H), 7.29 (q, J = 8.0 Hz, 1H), 7.18–7.15 (m, 5H), 7.11 (q, J = 7.0 Hz, 1H), 6.99–6.92 (m, 3H), 6.73–6.69 (m, 2H), 6.45 (d, J = 2.5 Hz, 1H), 6.27 (d, J = 8.5 Hz, 1H), 3.0 (s, 3H). 13C NMR (125 MHz, d6-DMSO) δ (ppm) 192.09, 192.09, 188.65, 170.99, 170.47, 169.04, 157.53, 156.65, 150.94, 150.11, 150.04, 148.67, 148.41, 147.92, 145.87, 145.81, 145.73, 141.56, 141.48, 135.48, 135.30, 134.36, 134.19, 134.09, 133.56, 133.38, 133.27, 131.68, 131.11, 131.04, 129.37, 129.35, 129.28, 128.71, 128.56, 128.48, 128.39, 128.34, 128.09, 127.81, 127.73, 127.20, 126.26, 126.17, 124.96, 124.88, 124.54, 123.27, 123.23, 120.20, 120.15, 117.34, 112.31, 112.22, 36.55 MS m/z: [M]+ calcd for C54H36IrN6OPS2, 1072.2; found, 1072.2. Elemental analysis calcd for C54H36IrN6OPS2: C, 60.49; H, 3.38; N, 7.84. Found: C, 60.28; N, 8.00; H, 3.08.
Conflicts of interest
The authors declare no competing financial interests.
Acknowledgements
This work was supported by funding from the Joint Fund Project of the Natural Science Foundation of Jilin Province (YDZJ202101ZYTS063).
References
- M. A. Baldo, D. F. O'Brien, Y. You, A. Shoustikov, S. Sibley, M. E. Thompson and S. R. Forrest, Highly efficient phosphorescent emission from organic electroluminescent devices, Nature, 1998, 395, 151 CrossRef CAS.
- T. Fleetham, G. Li, L. Wen and J. Li, Efficient “Pure” Blue OLEDs Employing Tetradentate Pt Complexes with a Narrow Spectral Bandwidth, Adv. Mater., 2014, 26, 7116 CrossRef CAS.
- G. Hong, X. Gan, C. Leonhardt, Z. Zhang, J. Seibert, J. M. Busch and S. Brase, A Brief History of OLEDs-Emitter Development and Industry Milestones, Adv. Mater., 2021, 33, e2005630 CrossRef.
- P. Tao, X. Lü, G. Zhou and W.-Y. Wong, Asymmetric Tris-Heteroleptic Cyclometalated Phosphorescent Iridium(III) Complexes: An Emerging Class of Metallophosphors, Acc. Mater. Res., 2022, 3, 830 CrossRef CAS.
- Y. Zhang and J. Qiao, Near-infrared emitting iridium complexes: Molecular design, photophysical properties, and related applications, iScience, 2021, 24, 102858 CrossRef CAS.
- T. Zhang, Y. Xiao, H. Wang, S. Kong, R. Huang, V. K. M. Au, T. Yu and W. Huang, Highly Twisted Thermally Activated Delayed Fluorescence (TADF) Molecules and Their Applications in Organic Light-Emitting Diodes (OLEDs), Angew. Chem., Int. Ed., 2023, e202301896, DOI:10.1002/anie.202301896.
- J. Jayabharathi, V. Thanikachalam and S. Thilagavathy, Phosphorescent organic light-emitting devices: Iridium based emitter materials – An overview, Coord. Chem. Rev., 2023, 483, 215100 CrossRef CAS.
- G. Lu, Z. G. Wu, R. Wu, X. Cao, L. Zhou, Y. X. Zheng and C. Yang, Semitransparent Circularly Polarized Phosphorescent Organic Light–Emitting Diodes with External Quantum Efficiency over 30% and Dissymmetry Factor Close to 10–2, Adv. Funct. Mater., 2021, 31, 2102898 CrossRef CAS.
- M. Wu, N. Li, C. Shi, J. Song, R. Zeng, F. Li, Q. Li, A. Yuan and C. Yang, A narrowband red-emitting asymmetric iridium(III) complex featuring B- and N-embedded π-conjugation units: structure, photophysics and OLED application, Inorg. Chem. Front., 2023, 10, 1262 RSC.
- C. Ou, Y.-C. Qiu, C. Cao, H. Zhang, J. Qin, Z.-L. Tu, J. Shi and Z.-G. Wu, Modulating the peripheral large steric hindrance of iridium complexes for achieving narrowband emission and pure red OLEDs with an EQE up to 32.0%, Inorg. Chem. Front., 2023, 10, 1018 RSC.
- C. You, D. Liu, J. Yu, H. Tan, M. Zhu, B. Zhang, Y. Liu, Y. Wang and W. Zhu, Boosting Efficiency of Near–Infrared Emitting Iridium(III) Phosphors by Administrating Their π–π Conjugation Effect of Core–Shell Structure in Solution–Processed OLEDs, Adv. Opt. Mater., 2020, 8, 2000154 CrossRef CAS.
- M. Idris, S. C. Kapper, A. C. Tadle, T. Batagoda, D. S. M. Ravinson, O. Abimbola, P. I. Djurovich, J. Kim, C. Coburn, S. R. Forrest and M. E. Thompson, Blue Emissive fac/mer–Iridium(III) NHC Carbene Complexes and their Application in OLEDs, Adv. Opt. Mater., 2021, 9, 2001994 CrossRef CAS.
- Y. Sun, X. Yang, Z. Feng, B. Liu, D. Zhong, J. Zhang, G. Zhou and Z. Wu, Highly Efficient Deep-Red Organic Light-Emitting Devices Based on Asymmetric Iridium(III) Complexes with the Thianthrene 5,5,10,10-Tetraoxide Moiety, ACS Appl. Mater. Interfaces, 2019, 11, 26152 CrossRef CAS PubMed.
- X. Yang, X. Zhou, Y. X. Zhang, D. Li, C. Li, C. You, T. C. Chou, S. J. Su, P. T. Chou and Y. Chi, Blue Phosphorescence and Hyperluminescence
Generated from Imidazo[4,5-b]pyridin-2-ylidene-Based Iridium(III) Phosphors, Adv. Sci., 2022, 9, e2201150 CrossRef.
- C. Guo, S. Guo, Q. Lu, Z. Jiang, Y. Yang, W. Zhou, Q. Zeng, J. Liang, Y. Miao and Y. Liu, Solution-Processed Yellow Organic Light-Emitting Diodes Based on Two New Ionic Ir(III) Complexes, Molecules, 2022, 27, 2840 CrossRef CAS PubMed.
- H. Y. Park, A. Maheshwaran, C. K. Moon, H. Lee, S. S. Reddy, V. G. Sree, J. Yoon, J. W. Kim, J. H. Kwon, J. J. Kim and S. H. Jin, External Quantum Efficiency Exceeding 24% with CIE(y) Value of 0.08 using a Novel Carbene-Based Iridium Complex in Deep-Blue Phosphorescent Organic Light-Emitting Diodes, Adv. Mater., 2020, 32, e2002120 CrossRef.
- T. Sajoto, P. I. Djurovich, A. B. Tamayo, J. Oxgaard, W. A. Goddard III and M. E. Thompson, Temperature Dependence of Blue Phosphorescent Cyclometalated Ir(III) Complexes, J. Am. Chem. Soc., 2009, 131, 9813 CrossRef CAS.
- M. Bixon and J. Jortner, Intramolecular Radiationless Transitions, J. Chem. Phys., 2003, 48, 715 CrossRef.
- R. Englman and J. Jortner, The energy gap law for radiationless transitions in large molecules, Mol. Phys., 1970, 18, 145 CrossRef CAS.
- J. V. Caspar, E. M. Kober, B. P. Sullivan and T. J. Meyer, Application of the energy gap law to the decay of charge-transfer excited states, J. Am. Chem. Soc., 1982, 104, 630 CrossRef CAS.
- J. Qiao, L. Duan, L. Tang, L. He, L. Wang and Y. Qiu, High-efficiency orange to near-infrared emissions from bis-cyclometalated iridium complexes with phenyl-benzoquinoline isomers as ligands, J. Mater. Chem., 2009, 19, 6573 RSC.
- P. N. Lai, S. Yoon and T. S. Teets, Efficient near-infrared luminescence from bis-cyclometalated iridium(III) complexes with rigid quinoline-derived ancillary ligands, Chem. Commun., 2020, 56, 8754 RSC.
- C. You, D. Liu, L. Wang, W. Zheng, M. Li, P. Wang and W. Zhu, Rigid phenanthro[4,5-abc]phenazine-cored iridium(III) complexes for high-performance near-infrared emission at about 800 nm in solution-processed OLEDs, Chem. Eng. J., 2023, 452, 138956 CrossRef CAS.
- P. Rajakannu, H. S. Kim, W. Lee, A. Kumar, M. H. Lee and S. Yoo, Naphthalene Benzimidazole Based Neutral Ir(III) Emitters for Deep Red Organic Light-Emitting Diodes, Inorg. Chem., 2020, 59, 12461 CrossRef CAS.
- E. Kabir, Y. Wu, S. Sittel, B.-L. Nguyen and T. S. Teets, Improved deep-red phosphorescence in cyclometalated iridium complexes via ancillary ligand modification, Inorg. Chem. Front., 2020, 7, 1362 RSC.
- P. N. Lai, S. Yoon, Y. Wu and T. S. Teets, Effects of Ancillary Ligands on Deep Red to Near-Infrared Cyclometalated Iridium Complexes, ACS Org. Inorg. Au, 2022, 2, 236 CrossRef CAS.
- P. N. Lai, C. H. Brysacz, M. K. Alam, N. A. Ayoub, T. G. Gray, J. Bao and T. S. Teets, Highly Efficient Red-Emitting Bis-Cyclometalated Iridium Complexes, J. Am. Chem. Soc., 2018, 140, 10198 CrossRef CAS.
- Z. Chen, H. Zhang, D. Wen, W. Wu, Q. Zeng, S. Chen and W. Y. Wong, A simple and efficient approach toward deep-red to near-infrared-emitting iridium(III) complexes for organic light-emitting diodes with external quantum efficiencies of over 10, Chem. Sci., 2020, 11, 2342 RSC.
- S. Yoon and T. S. Teets, Red to near-infrared phosphorescent Ir(III) complexes with electron-rich chelating ligands, Chem. Commun., 2021, 57, 1975 RSC.
- H. Zhang, Y. Xu, Z. Chen, S. Chen, R. Liu and W. Y. Wong, Iridium(III) complexes with 1-phenylisoquinoline-4-carbonitrile units for efficient NIR organic light-emitting diodes, iScience, 2021, 24, 102911 CrossRef CAS PubMed.
- M. Z. Shafikov, A. V. Zaytsev, A. F. Suleymanova, F. Brandl, A. Kowalczyk, M. Gapinska, K. Kowalski, V. N. Kozhevnikov and R. Czerwieniec, Near Infrared Phosphorescent Dinuclear Ir(III) Complex Exhibiting Unusually Slow Intersystem Crossing and Dual Emissive Behavior, J. Phys. Chem. Lett., 2020, 11, 5849 CrossRef CAS.
- S. Kesarkar, W. Mroz, M. Penconi, M. Pasini, S. Destri, M. Cazzaniga, D. Ceresoli, P. R. Mussini, C. Baldoli, U. Giovanella and A. Bossi, Near-IR Emitting Iridium(III) Complexes with Heteroaromatic beta-Diketonate Ancillary Ligands for Efficient Solution-Processed OLEDs: Structure-Property Correlations, Angew. Chem., Int. Ed., 2016, 55, 2714 CrossRef CAS.
- M. Penconi, A. B. Kajjam, M.-C. Jung, M. Cazzaniga, C. Baldoli, D. Ceresoli, M. E. Thompson and A. Bossi, Advancing Near-Infrared Phosphorescence with Heteroleptic Iridium Complexes Bearing a Single Emitting Ligand: Properties and Organic Light-Emitting Diode Applications, Chem. Mater., 2022, 34, 574 CrossRef CAS.
- M. Nonoyama, Benzo[h]quinolin-10-yl-N Iridium(III) Complexes, Bull. Chem. Soc. Jpn., 1974, 47, 767–768 CrossRef CAS.
- G. N. Li, Y. Zou, Y. D. Yang, J. Liang, F. Cui, T. Zheng, H. Xie and Z. G. Niu, Deep-red phosphorescent iridium(III) complexes containing 1-(benzo[b] thiophen-2-yl) isoquinoline ligand: synthesis, photophysical and electrochemical properties and DFT calculations, J. Fluoresc., 2014, 24, 1545 CrossRef CAS.
- S. Hecht, N. Vladimirov and J. M. J. Fréchet, Encapsulation of Functional Moieties within Branched Star Polymers: Effect of Chain Length and Solvent on Site Isolation, J. Am. Chem. Soc., 2001, 123, 18–25 CrossRef CAS.
-
M. J. Frisch, G. W. Trucks, H. B. Schlegel, G. E. Scuseria, M. A. Robb, J. R. Cheeseman, G. Scalmani, V. Barone, G. A. Petersson, H. Nakatsuji, X. Li, M. Caricato, A. V. Marenich, J. Bloino, B. G. Janesko, R. Gomperts, B. Mennucci, H. P. Hratchian, J. V. Ortiz, A. F. Izmaylov, J. L. Sonnenberg, D. Williams-Young, F. Ding, F. Lipparini, F. Egidi, J. Goings, B. Peng, A. Petrone, T. Henderson, D. Ranasinghe, V. G. Zakrzewski, J. Gao, N. Rega, G. Zheng, W. Liang, M. Hada, M. Ehara, K. Toyota, R. Fukuda, J. Hasegawa, M. Ishida, T. Nakajima, Y. Honda, O. Kitao, H. Nakai, T. Vreven, K. Throssell, J. A. Montgomery Jr., J. E. Peralta, F. Ogliaro, M. J. Bearpark, J. J. Heyd, E. N. Brothers, K. N. Kudin, V. N. Staroverov, T. A. Keith, R. Kobayashi, J. Normand, K. Raghavachari, A. P. Rendell, J. C. Burant, S. S. Iyengar, J. Tomasi, M. Cossi, J. M. Millam, M. Klene, C. Adamo, R. Cammi, J. W. Ochterski, R. L. Martin, K. Morokuma, O. Farkas, J. B. Foresman and D. J. Fox, Gaussian 16, Revision C.01, Gaussian Inc., Wallingford CT, 2019 Search PubMed.
- A. Beeby, S. Bettington, I. D. W. Samuel and Z. Wang, Tuning the emission of cyclometalated iridium complexes by simple ligand modification, J. Mater. Chem., 2003, 13, 80–83 RSC.
- L. Zhang, Z.-P. Yan and Y.-X. Zheng, Efficient sky-blue OLEDs with extremely low efficiency roll-off based on stable iridium complexes with a bis(diphenylphorothioyl)amide ligand, Dalton Trans., 2019, 48, 9744–9750 RSC.
- X.-F. Ma, J.-C. Xia, Z.-P. Yan, X.-F. Luo, Z.-G. Wu, Y.-X. Zheng and W.-W. Zhang, Highly efficient green and red electroluminescence with an extremely low efficiency roll-off based on iridium(III) complexes containing a bis(diphenylphorothioyl)amide ancillary ligand, J. Mater. Chem. C, 2019, 7, 2570–2576 RSC.
Footnote |
† Electronic supplementary information (ESI) available: Structural characterization and general information, and crystallographic data. CCDC 2155915. For ESI and crystallographic data in CIF or other electronic format see DOI: https://doi.org/10.1039/d3qi01824e |
|
This journal is © the Partner Organisations 2024 |
Click here to see how this site uses Cookies. View our privacy policy here.