DOI:
10.1039/D3QI01864D
(Research Article)
Inorg. Chem. Front., 2024,
11, 278-285
Shape-controlled synthesis and self-assembly of highly uniform upconverting calcium fluoride nanocrystals†
Received
15th September 2023
, Accepted 9th November 2023
First published on 13th November 2023
Abstract
Herein, the size- and shape-controlled synthesis of nearly-monodisperse, lanthanide-doped calcium fluoride (CaF2) nanocrystals (NCs) is reported. The sizes and shapes of CaF2 NCs are controlled by tailoring the reaction conditions, such as the concentration of lithium fluoride precursors, reaction time and temperature, and the procedure for adding the calcium trifluoroacetate precursors in the reaction mixture. Highly uniform CaF2 NCs are synthesized with several morphologies, such as nanospheres, truncated octahedra, nanoplates, and nanowires. The shape-controlled CaF2 NCs self-assemble into NC superlattices with long-range orientational and positional order forming crystalline and liquid crystalline structures. The near-infrared-to-visible upconversion luminescence properties are investigated by varying the types of dopants as well as the sizes and shapes of the CaF2 NCs.
Introduction
Lanthanide-based luminescent nanocrystals (NCs) offer immense potential in many emerging applications, such as biomedical imaging,1,2 optical sensors,3,4 spectral converters for photovoltaics5,6 and agriculture,7 and displays.8 Lanthanides are f-block elements with partially-filled and well shielded f-orbitals. Their ions are responsible for the characteristic atomic optical absorption and emission spectra, the long, several microsecond excited-state lifetimes, and the magnetic properties of the NCs. Optically-active, lanthanide-doped NCs show unique optical properties, such as upconversion luminescence (UC),9 quantum cutting,10 and downshifting11 are observed as energy is transferred between excited-state atomic levels in the dopants.12 The composition and crystal structure of the host material plays a critical role in influencing the NC luminescence properties.13,14 Utilization of lanthanide materials in large-scale industrial applications requires the development of host materials using inexpensive and environmentally friendly elements, while maintaining their desired physical and chemical properties.
To date, various synthetic procedures for the preparation of lanthanide-based NCs have been reported, which include hydro- and solvo-thermal syntheses,15,16 as well as co-precipitation17 and thermal decomposition methods.18,19 Among these, thermal decomposition has been widely investigated to afford highly uniform NCs, which has also been utilized for the synthesis of semiconducting quantum dots20 and metal oxide NCs.21,22 The thermolysis of organometallic precursors at high temperature enables the formation of uniform NCs with controlled crystal structures. The sizes, shapes, and compositions of the NCs can be easily tailored by controlling the reaction parameters, such as time, temperature, precursor ratio, and temperature ramping time. In lanthanide-doped NCs, the size23,24 and shape25 of hosts, distance between dopants,26,27 and the spatial position of dopants28 can be tailored during the synthesis, allowing their UC properties to be precisely controlled. Therefore, the development of a synthetic method to precisely control the sizes and morphologies of lanthanide-doped NCs is critical to optimizing the UC properties.
Several types of host materials, such as sodium lanthanide fluorides,14,29–31 lanthanide oxides,32–35 lanthanide fluorides,18,36 lanthanide oxyfluorides,37,38 and alkali earth fluorides,39,40 have been reported for lanthanide-doped NCs. Among these, CaF2 is a promising candidate host material, as the rare earth elements common in other host compositions are substituted by inexpensive and abundant calcium. CaF2 is an inert and non-hygroscopic material showing high chemical and thermal resistance. In addition, CaF2 shows broadband transmittance in the ultraviolet, visible, near-infrared (NIR), and even mid-IR regions. CaF2 is a biocompatible material exhibiting low toxicity, which allows its application in biomedicine.41 Lanthanide-doped CaF2 is used as a scintillating material for the detection of gamma rays and charged particles,42,43 allowing the utilization of rare-earth-doped NCs as spectral converters for high-energy radiation in bio-imaging applications44 and radiation detection.45,46 Although many literature reports have described the synthesis of uniform CaF2 NCs,16,47,48 the development of a synthetic procedure to precisely control the sizes and shapes of highly uniform lanthanide-doped CaF2 NCs in a reproducible manner is still challenging.
Herein, a size- and shape-controlled synthesis of upconverting CaF2 NCs is reported. Nearly-monodisperse CaF2 NCs are synthesized via the high-temperature thermal decomposition of calcium trifluoroacetate (CaTFA2) precursors in a mixture of lithium fluoride (LiF), oleic acid (OA), and 1-octadecene (ODE). The addition of LiF in the reaction mixture and the control of the relative ratio of OA to ODE affords CaF2 NCs with tunable morphologies including nanospheres (NSs), truncated octahedra (NOs), nanoplates (NPs), and nanowires (NWs). Highly uniform CaF2 NCs self-assemble into close-packed superlattices over large areas via liquid–air interfacial assembly. CaF2 NWs self-assemble into liquid-crystalline superlattices with long-range orientational and positional order. The optical properties of upconverting CaF2 NCs are investigated. First, the NIR-to-visible two-photon upconversion luminescence is studied using Er3+/Yb3+, Tm3+/Yb3+, and Tm3+/Er3+/Yb3+-doped CaF2 NCs, which exhibit characteristic blue, green, red, and NIR emission peaks upon excitation at 980 nm depending on the types of dopants. Next, the UC properties of the Er3+/Yb3+-doped CaF2 NCs with varying sizes and shapes are investigated using highly uniform CaF2 NCs.
Results and discussion
The CaF2 NCs were synthesized by adding CaTFA2 and LiF to a 50
:
50 (v/v) OA/ODE mixture. The CaTFA2 precursors were synthesized by dissolving CaCO3 in trifluoroacetic acid at room temperature, followed by solvent evaporation. The reaction solution was preheated at 125 °C under vacuum for 1 h to remove water and volatile solvents. The reaction mixture was then heated to 300 °C and maintained at this reaction temperature for 30 min. During the synthesis, LiF does not completely dissolve in the solvent mixtures and non-polar solvent, whereas CaTFA2 and the CaF2 NCS show complete dissolution. The residual LiF was thus removed by dissolving the CaF2 NCs in a non-polar solvent, such as hexane, toluene, or chloroform, followed by centrifugation.
Fig. 1a and b respectively show representative low- and high-magnification transmission electron microscopy (TEM) images of Er3+,Yb3+-co-doped CaF2 NCs synthesized at 300 °C; a 1
:
3 molar ratio of CaTFA2 to LiF was used in this reaction. The low-magnification TEM image indicates the high uniformity in size and shape of the CaF2 NCs achieved by this method. The high-magnification TEM image shows the NCs have pseudo-spherical morphologies. Fig. 1c–f show high-resolution TEM (HRTEM) images of single CaF2 NCs. The single-crystal structures are observed from the fringe interference patterns of the isolated NCs. The HRTEM images show CaF2 NCs oriented along the [110] and [112] zone axes, respectively, which are further validated by the fast Fourier transforms (FFTs) of the HRTEM data (Fig. 1d and f) and simulated electron diffraction patterns (Fig. S1†). The average size and size distribution of the NCs are obtained from the small-angle X-ray scattering (SAXS) data (Fig. 1g). The simulated small-angle X-ray patterns are well-matched to the experimental results with an input parameter of 9.2 ± 0.8 nm diameter, which represents the high size uniformity of the CaF2 NCs. The powder X-ray diffraction data reveals the face-centered cubic crystal structures of the CaF2 NCs (JCPDS No. 87-0971, space group: Fm3m).
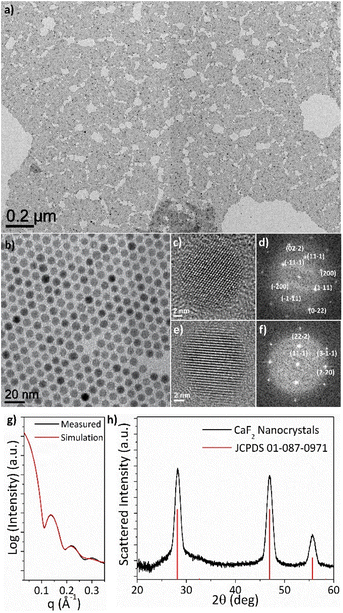 |
| Fig. 1 (a) Low-magnification and (b) high-magnification transmission electron microscopy (TEM) images of upconverting CaF2:2%Er3+,20%Yb3+ NCs. High-resolution TEM (HRTEM) images of an isolated CaF2 nanocrystal (NC) along the (c) [110] and (e) [112] projections, and (d and f) fast Fourier transform of the respective HRTEM images. (g) Small-angle X-ray scattering data with simulated pattern and (h) wide-angle X-ray diffraction spectrum of Er3+,Yb3+-co-doped CaF2 NCs. | |
The sizes of the CaF2 NCs are easily tuned by changing the reaction temperature. When the reaction is performed at a temperature of 320 °C, the average size of the CaF2 NCs increases to 10.8 ± 0.8 nm in diameter (Fig. S2†). The sizes of the CaF2 NCs are increased further by modifying the method for adding the metal precursors in the reaction solution. When the CaTFA2 precursor in the OA and ODE solution is slowly injected into the solution mixtures containing LiF at 320 °C, truncated octahedral CaF2 NCs are obtained as the final product (Fig. 2). For the reaction, two reaction vessels are prepared with LiF on one flask and CaTFA2 precursors in OA and ODE (50
:
50 vol%) on the other side. Both reaction mixtures are degassed at 125 °C for one hour to remove water and volatile solvent. After degassing, the temperature of the reactor containing LiF in 40 mL of the OA/ODE mixture is increased to 320 °C, and the solution containing CaTFA2 in 20 mL of the solvent mixture is slowly added at 1.33 mL min−1 using a syringe pump. The solution with the CaTFA2 precursor is pumped into the flask over 15 min, which is then held for additional 15 min for the further growth of NCs. Fig. 2 shows TEM images of the truncated CaF2 octahedra at low and high magnification to show their uniformity and the top and side views of the truncated CaF2 octahedral NCs (Fig. 2b and c). The tip-to-tip distance of the truncated CaF2 octahedra is approximately 25.3 ± 2.6 nm, as calculated by statistical analysis from TEM images. During the slow injection of the metal precursors, few nuclei are formed in the reaction mixture during the early stage of injection, and the growth of the NCs occurs from these nuclei at a high temperature, which results in the formation of larger NCs.49
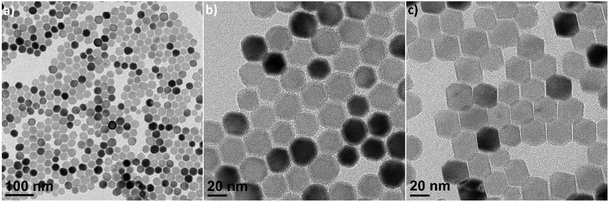 |
| Fig. 2 TEM images of CaF2 NCs showing truncated octahedral morphologies. (a) Low-magnification, (b) top-view, and (c) side-view images of the truncated octahedra. | |
Synthesis of the NCs without the addition of LiF in the reaction mixture yields CaF2 nanoplates with rectangular plate-like morphologies, as reported in prior literature.40 The morphologies are further tuned by changing the relative ratio of OA to ODE added into the reaction mixture. When the relative amount of OA to ODE is decreased from 50 vol% to 1.6 vol%, CaF2 grows in a uniaxial direction to form NWs with high aspect ratios. As shown in Fig. 3c, the widths of the NWs are ≤3 nm and the lengths are up to a micrometer. Some NWs are thin in the middle, while a plate-like shape is observed at the end of the NWs with approximately 10 nm widths (Fig. 3d and S3†). During the synthesis of the CaF2 NWs, carried out at 280 °C, the viscosity of the reaction solution increases 15 min into the reaction, which may indicate the formation of the CaF2 NWs.50,51 When the reaction time or reaction temperature is increased, the NWs dissociate into smaller NWs and nanorods, eventually forming two-dimensional nanoplate morphologies (Fig. S4†). These results indicate that the kinetically stable NWs are formed at the beginning, which eventually transform into nanoplates to minimize the surface energy by decreasing the relative surface area. With the same solvent ratio of OA to ODE (1.6 vol% OA), when the reaction is performed with LiF salts, isotropic spherical NCs are obtained as the final product, indicating that LiF induces the isotropic growth of CaF2 NCs.
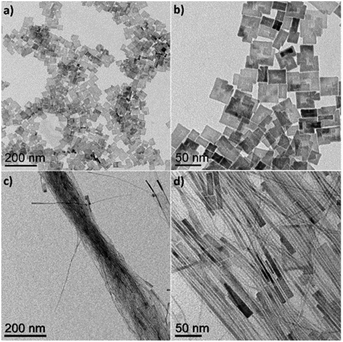 |
| Fig. 3 TEM images of (a and b) CaF2 nanoplates and (c and d) CaF2 nanowires. | |
The self-assembly of the colloidal NCs into ordered nanostructures allows the utilization of their unique size-, shape-, and orientation-dependent properties, which is of considerable interest for photonic,52 plasmonic,53 electronic,54 and electrochemical applications.55 We used liquid interfacial assembly to organize superlattices of CaF2 NCs with varying shapes. Liquid interfacial assembly is a facile, robust, and reproducible technique to fabricate uniform single and binary superlattices from isotropic and anisotropic NC building blocks.56–58 First, we self-assembled the spherical CaF2 NCs as shown in Fig. 1a and b. TEM images reveal that CaF2 NC superlattices self-assembled over a large area (Fig. 4a). The sharp, hexagonal small-angle selected area electron diffraction (SAED) pattern reveals the long-range ordering of two-dimensional close-packed hexagonal arrays of CaF2 NCs stacked into bilayer superlattices. The wide-angle SAED pattern of the self-assembled superlattice also shows relatively intense single-crystal-like diffraction spots with six-fold symmetry (Fig. 4b, inset). This is attributed to the preferential alignment and perpendicular orientation of the [111] axis of all NCs on the substrate, owing to the faceting of the CaF2 NCs.
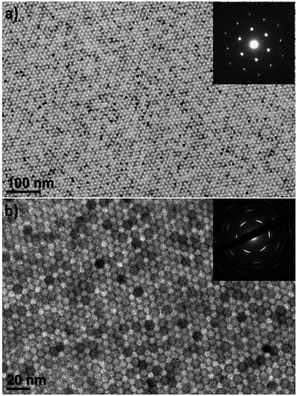 |
| Fig. 4 Assemblies of CaF2 NCs forming (a) bilayer and (b) trilayer superlattices. Inset images show the (a) small-angle and (b) wide-angle SAED patterns, exhibiting the CaF2 lattice in the [111] plane. | |
The upconverting CaF2 NWs, as exemplified for Er3+,Yb3+-co-doped CaF2 NWs, self-assemble into superlattices with liquid crystalline order (Fig. 5). The domain sizes of the superlattices are up to a micrometer in scale with the preservation of long-range orientational order. The single-crystalline SAED patterns indicate that the NWs are oriented in the same crystallographic direction, not only along their long axes, but also their short axes. The SAED patterns show two sets of diffraction patterns. The NWs are projected from the [110] zone axis in the cubic crystal lattices, resulting in an angle of 54.7° between the diffraction spots of the (100) and (111) planes (Fig. S5†). Another set of diffraction data corresponding to the (110) plane with four-fold symmetry is also seen in the SAED pattern of the self-assembled structures, representing the diffraction spots projected along the [100] zone axis. This indicates that the cross-sections of the NWs are faceted with (100) and (110) planes, allowing the preferential orientation of NWs along either the (100) or (110) plane parallel to the substrate, as shown in Fig. S5.†
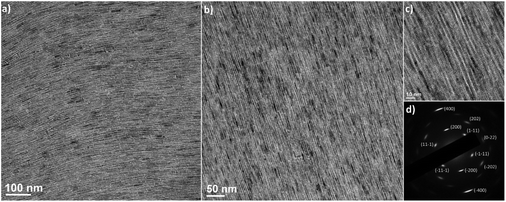 |
| Fig. 5 (a and b) Low-magnification and (c) high-magnification TEM images of liquid-crystalline superlattices of Er3+,Yb3+-co-doped CaF2 NWs. (d) The wide-angle electron diffraction data. | |
The UC properties of the lanthanide-doped CaF2 NCs are investigated by incorporating optically active lanthanide ions in the CaF2 NC host. First, the two-photon UC properties are characterized in Er3+/Yb3+, Tm3+/Yb3+, and Tm3+/Er3+/Yb3+-doped CaF2 NCs. Fig. 6a and b show a photograph and the photoluminescence (PL) spectra for dispersions of the upconverting CaF2 NCs illuminated with 980 nm radiation. Upon excitation at 980 nm, blue, orange, and red emissions are observed for CaF2 doped with Tm3+/Yb3+, Er3+/Yb3+, and Tm3+/Er3+/Yb3+, respectively. The 0.2%Tm3+/20%Yb3+-doped CaF2 NCs show characteristic blue and NIR peaks at 480 and 800 nm. The weak blue emission is attributed to the 1D2→3F4 and 1G4→2H6 transitions, and the NIR emission at 800 nm corresponds to the radiative transition from the 3H4→3H6 energy levels of the Tm3+ dopants. With the 2%Er3+/20%Yb3+ dopants, green (521 and 539 nm) and red (656 nm) peaks are observed, which are the characteristic emission lines of Er3+ dopants for 2H11/2→4I15/2 and 4S3/2→4I15/2 (green) and 4F9/2→4I15/2 (red) transitions. Triply-doped CaF2 NCs with 0.5%Tm3+/2%Er3+/20%Yb3+ ions exhibit emission peaks at 480, 539, 656, and 800 nm, which are attributed to emission from both Tm3+ and Er3+. In addition, the relative intensity of NIR peaks (800 nm) to visible peaks (480, 539, 656 nm) increases with an increase in the relative ratio of Tm3+ in the compositions of the dopants (Fig. S6†), attributed to the relaxation from higher flevels in Er3+ to lower 3H4-levels in Tm3+ with increasing concentration of Tm3+ ions in the host materials.
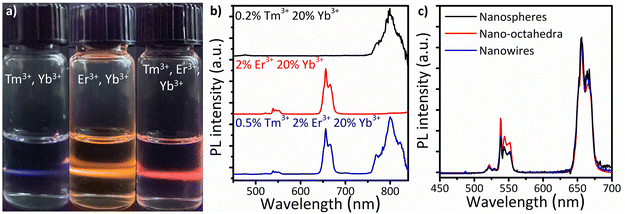 |
| Fig. 6 (a) Photograph of upconverting CaF2 doped with 0.2%Tm3+/20%Yb3+ (left), 2%Er3+/20%Yb3+ (middle), and 0.5%Tm3+/2%Er3+/20%Yb3+ (right) NCs under 980 nm irradiation. UC spectra of CaF2 NCs (b) with varying dopant compositions and (c) different sizes and shapes. | |
In addition, the size and shape dependence of the UC properties is demonstrated using CaF2 NSs, NOs, and NWs. Fig. S7† shows the TEM images of the CaF2 NCs used for the UC measurements. The sizes of CaF2 NSs and NOs are measured to be 12.1 ± 1.1 nm and 17.3 ± 1.2 nm, respectively, exhibiting high size uniformity. All NCs are doped with 2%Er3+ and 20%Yb3+ ions as experimentally confirmed by energy dispersive X-ray spectroscopy in the scanning transmission electron microscope (Table S1†). The larger-sized CaF2 NOs exhibit the highest UC intensity, whereas the CaF2 NWs show the weakest UC intensities under identical measurement conditions (Fig. 6c). This difference might be attributed to the surface-to-volume ratio of CaF2 NCs, where smaller-sized NCs exhibit a larger surface area, resulting in a higher population of surface ions. The surface lanthanide ions can potentially introduce defects, inducing nonradiative quenching, subsequently reducing the UC intensities.59Fig. 6c describes the UC spectra of CaF2 NSs, NOs, and NWs normalized to the red emission peaks at 656 nm. The green-to-red intensity ratio in the UC spectra is influenced by several factors, such as the particle size,23 shape,60 the excitation power density,61 and solvents.62 When other factors are kept constant, the green-to-red ratio decreases with decreasing NC size owing to the larger surface-to-volume ratio.63 Non-radiative relaxation is mediated by nanocrystal phonons, the vibration of surface ligands, and surface defects.64 Consequently, the large surface-to-volume ratio of smaller-sized CaF2 NSs enhances multiphonon relaxation between f levels, favoring the population of the 4F9/2 state over 2H11/2 and 4S3 states.59 In contrast, the green-to-red intensity ratio of CaF2 NWs is nearly identical to that of NSs. Shan et al. reported shape effects on the green-to-red intensity ratio of hexagonal-phase Er3+,Yb3+-doped β-phase (hexagonal) NaGdF4 NCs, attributed to the crystal anisotropy between the a-axis and c-axis in β-phase NaGdF4 structures, influencing the UC intensities and green-to-red intensity ratio. In contrast, the green-to-red intensity ratio of Er3+ in CaF2 NCs was relatively insensitive to the shape compared to the β-phase NaGdF4 attributed to the highly symmetric crystal structure of the CaF2 hosts.
Conclusions
In summary, the shape-controlled synthesis and self-assembly of luminescent CaF2 NCs are described. Highly uniform CaF2 NCs are synthesized via high-temperature thermal decomposition. The shapes of the CaF2 NCs are tunable by changing the reaction conditions, such as the addition of LiF salts, method of precursor injection, and relative ratio of OA to ODE. In the presence of LiF, highly uniform pseudo-spherical CaF2 NCs are obtained, and their sizes can be further tuned by changing the reaction temperature. The CaF2 NCs with truncated octahedral shapes are synthesized by slowly injecting the metal precursor solution at high temperature. In the absence of LiF, the shapes of CaF2 NCs are tailored from square nanoplates to NWs, depending on the relative ratio of OA to ODE. CaF2 NCs are self-assembled into ordered arrays via liquid interfacial self-assembly with a long-range order over a large area. The UC luminescence properties are investigated using Er3+/Yb3+, Tm3+/Yb3+, and Tm3+/Er3+/Yb3+-doped CaF2 NCs as well as Er3+/Yb3+-doped CaF2 NCs with different sizes and shapes. The uniformity of the sizes and shapes of CaF2 NCs comprising the inexpensive and abundant calcium can allow the utilization of upconverting NCs for large-scale applications.
Author contributions
Taejong Paik: conceptualization, methodology, investigation, funding acquisition, supervision, writing – original draft, and writing – review & editing. Nicholas J. Greybush: methodology, investigation, data curation, and formal analysis. Ho Young Woo: investigation, data curation, and formal analysis. Seong Vin Hong: data curation and formal analysis. Seung Hyeon Kim: data curation and formal analysis. Jennifer D. Lee: data curation and formal analysis. Cherie R. Kagan: conceptualization, funding acquisition, supervision, and writing – review & editing. Christopher B. Murray: conceptualization, funding acquisition, supervision, and writing – review & editing.
Conflicts of interest
There are no conflicts to declare.
Acknowledgements
This research was supported by the Creative Materials Discovery Program through the National Research Foundation of Korea (NRF) grants funded by the Korean government (2018M3D1A1059001, 2021M3H2A1038042, 2021M3H4A3A01062960, 2021R1A2C1013604, and 2022R1A4A2000776). C. R. K. thanks for the support of Stephen J. Angello Professorship. C. B. M. is grateful for the support of Richard Perry University Professorship.
References
- F. Wang and X. Liu, Recent Advances in the Chemistry of Lanthanide-Doped Upconversion Nanocrystals, Chem. Soc. Rev., 2009, 38, 976–989 RSC.
- M. Nyk, R. Kumar, T. Y. Ohulchanskyy, E. J. Bergey and P. N. Prasad, High Contrast in Vitro and in Vivo Photoluminescence Bioimaging Using Near Infrared to Near Infrared Up-Conversion in Tm3+ and Yb3+ Doped Fluoride Nanophosphors, Nano Lett., 2008, 8, 3834–3838 CrossRef CAS PubMed.
- L. Wang, R. Yan, Z. Huo, L. Wang, J. Zeng, J. Bao, X. Wang, Q. Peng and Y. Li, Fluorescence Resonant Energy Transfer Biosensor Based on Upconversion-Luminescent Nanoparticles, Angew. Chem., Int. Ed., 2005, 44, 6054–6057 CrossRef CAS PubMed.
- F. Vetrone, R. Naccache, A. Zamarrón, A. J. de la Fuente, F. Sanz-Rodríguez, L. M. Maestro, E. M. Rodriguez, D. Jaque, J. G. Solé and J. A. Capobianco, Temperature Sensing Using Fluorescent Nanothermometers, ACS Nano, 2010, 4, 3254–3258 CrossRef CAS PubMed.
- A. Shalav, B. S. Richards and M. A. Green, Luminescent Layers for Enhanced Silicon Solar Cell Performance: Up-Conversion, Sol. Energy Mater. Sol. Cells, 2007, 91, 829–842 CrossRef CAS.
- X. Huang, S. Han, W. Huang and X. Liu, Enhancing Solar Cell Efficiency: the Search for Luminescent Materials as Spectral Converters, Chem. Soc. Rev., 2013, 42, 173 RSC.
- L. Shen and X. Yin, Solar spectral management for natural photosynthesis: from photonics designs to potential applications, Nano Converg., 2022, 9, 36 CrossRef CAS PubMed.
- E. Downing, L. Hesselink, J. Ralston and R. Macfarlane, A Three-Color, Solid-State, Three-Dimensional Display, Science, 1996, 273, 1185–1189 CrossRef CAS.
- S. Heer, K. Kömpe, H.-U. Güdel and M. Haase, Highly Efficient Multicolour Upconversion Emission in Transparent Colloids of Lanthanide-Doped NaYF4 Nanocrystals, Adv. Mater., 2004, 16, 2102–2105 CrossRef CAS.
- B. M. van der Ende, L. Aarts and A. Meijerink, Near–Infrared Quantum Cutting for Photovoltaics, Adv. Mater., 2009, 21, 3073–3077 CrossRef CAS.
- Y. Zhong, Z. Ma, S. Zhu, J. Yue, M. Zhang, A. L. Antaris, J. Yuan, R. Cui, H. Wan, Y. Zhou, W. Wang, N. F. Huang, J. Luo, Z. Hu and H. Dai, Boosting the Down-Shifting Luminescence of Rare-Earth Nanocrystals for Biological Imaging Beyond 1500 nm, Nat. Commun., 2017, 8, 737 CrossRef PubMed.
- F. Auzel, Upconversion and Anti-Stokes Processes with f and d Ions in Solids, Chem. Rev., 2004, 104, 139–174 CrossRef CAS PubMed.
- S. Heer, K. Kömpe, H.-U. Güdel and M. Haase, Crystal Phase Control of Luminescing α-NaGdF4:Eu3+and β-NaGdF4:Eu3+ Nanocrystals, Adv. Mater., 2004, 16, 2101–2105 CrossRef.
- F. Wang, Y. Han, C. S. Lim, Y. Lu, J. Wang, J. Xu, H. Chen, C. Zhang, M. Hong and X. Liu, Simultaneous Phase and Size Control of Upconversion Nanocrystals through Lanthanide Doping, Nature, 2010, 463, 1061–1065 CrossRef CAS PubMed.
- X. Wang, J. Zhuang, Q. Peng and Y. Li, A General Strategy for Nanocrystal Synthesis, Nature, 2005, 437, 121–124 CrossRef CAS PubMed.
- G. Wang, Q. Peng and Y. Li, Upconversion Luminescence of Monodisperse CaF2:Yb3+/Er3+ Nanocrystals, J. Am. Chem. Soc., 2009, 131, 14200–14201 CrossRef CAS PubMed.
- N. Martin, P. Boutinaud, R. Mahiou, J.-C. Cousseins and M. Bouderbala, Preparation
of Fluorides at 80 °C in the NaF-(Y, Yb, Pr)F3 System, J. Mater. Chem., 1999, 9, 125–128 RSC.
- Y.-W. Zhang, X. Sun, R. Si, L.-P. You and C.-H. Yan, Single-Crystalline and Monodisperse LaF3 Triangular Nanoplates from a Single-Source Precursor, J. Am. Chem. Soc., 2005, 127, 3260–3261 CrossRef CAS PubMed.
- J.-C. Boyer, F. Vetrone, L. A. Cuccia and J. A. Capobianco, Synthesis of Colloidal Upconverting NaYF4 Nanocrystals Doped With Er3+,Yb3+ and Tm3+,Yb3+ via Thermal Decomposition of Lanthanide Trifluoroacetate Precursors, J. Am. Chem. Soc., 2006, 128, 7444–7445 CrossRef CAS PubMed.
- C. B. Murray, C. R. Kagan and M. G. Bawendi, Synthesis and Characterization of Monodisperse Nanocrystals and Close-packed Nanocrystal Assemblies, Annu. Rev. Mater. Sci., 2000, 30, 545–610 CrossRef CAS.
- J. Park, K. An, Y. Hwang, J.-G. Park, H.-J. Noh, J.-Y. Kim, J.-H. Park, N.-M. Hwang and T. Hyeon, Ultra-Large-Scale Synthesis of Monodisperse Nanocrystals, Nat. Mater., 2004, 3, 891–895 CrossRef CAS PubMed.
- J. Kwon, W. J. Choi, U. Jeong, W. Jung, I. Hwang, K. H. Park, S. G. Ko, S. M. Park, N. A. Kotov and J. Yeom, Recent advances in chiral nanomaterials with unique electric and magnetic properties, Nano Converg., 2022, 9, 32 CrossRef CAS PubMed.
- D. Yuan, M. C. Tan, R. E. Riman and G. M. Chow, Comprehensive Study on the Size Effects of the Optical Properties of NaYF4:Yb,Er Nanocrystals, J. Phys. Chem. C, 2013, 117, 13297–13304 CrossRef CAS.
- A. D. Ostrowski, E. M. Chan, D. J. Gargas, E. M. Katz, G. Han, P. J. Schuck, D. J. Milliron and B. E. Cohen, Controlled Synthesis and Single-Particle Imaging of Bright, Sub-10 nm Lanthanide-Doped Upconverting Nanocrystals, ACS Nano, 2012, 6, 2686–2692 CrossRef CAS PubMed.
- A. Sánchez-Iglesias, M. Grzelczak, J. Pérez-Juste and L. M. Liz-Marzán, Binary Self-Assembly of Gold Nanowires with Nanospheres and Nanorods, Angew. Chem., Int. Ed., 2010, 49, 9985–9989 CrossRef PubMed.
- Y. Lu, J. Zhao, R. Zhang, Y. Liu, D. Liu, E. M. Goldys, X. Yang, P. Xi, A. Sunna, J. Lu, Y. Shi, R. C. Leif, Y. Huo, J. Shen, J. A. Piper, J. P. Robinson and D. Jin, Tunable Lifetime Multiplexing Using Luminescent Nanocrystals, Nat. Photonics, 2014, 8, 32–36 CrossRef CAS.
- J. Wang, R. Deng, M. A. MacDonald, B. Chen, J. Yuan, F. Wang, D. Chi, T. S. A. Hor, P. Zhang, G. Liu, Y. Han and X. Liu, Enhancing Multiphoton Upconversion Through Energy Clustering at Sublattice Level, Nat. Mater., 2014, 13, 157–162 CrossRef CAS PubMed.
- F. Wang, R. Deng, J. Wang, Q. Wang, Y. Han, H. Zhu, X. Chen and X. Liu, Tuning Upconversion Through Energy Migration in Core–Shell Nanoparticles, Nat. Mater., 2011, 10, 968–973 CrossRef CAS PubMed.
- H.-X. Mai, Y.-W. Zhang, R. Si, Z.-G. Yan, L.-d. Sun, L.-P. You and C.-H. Yan, High-Quality Sodium Rare-Earth Fluoride Nanocrystals: Controlled Synthesis and Optical Properties, J. Am. Chem. Soc., 2006, 128, 6426–6436 CrossRef CAS PubMed.
- J.-C. Boyer, L. A. Cuccia and J. A. Capobianco, Synthesis of Colloidal Upconverting NaYF4:Er3+/Yb3+ and Tm3+/Yb3+ Monodisperse Nanocrystals, Nano Lett., 2007, 7, 847–852 CrossRef CAS PubMed.
- X. Ye, J. E. Collins, Y. Kang, J. Chen, D. T. N. Chen, A. G. Yodh and C. B. Murray, Morphologically Controlled Synthesis of Colloidal Upconversion Nanophosphors and Their Shape-Directed Self-Assembly, Proc. Natl. Acad. Sci. U. S. A., 2010, 107, 22430–22435 CrossRef CAS PubMed.
- R. Si, Y.-W. Zhang, L.-P. You and C.-H. Yan, Rare-Earth Oxide Nanopolyhedra, Nanoplates, and Nanodisks, Angew. Chem., Int. Ed., 2005, 44, 3256–3260 CrossRef CAS PubMed.
- Z. Huo, C.-K. Tsung, W. Huang, M. Fardy, R. Yan, X. Zhang, Y. Li and P. Yang, Self-organized ultrathin oxide nanocrystals, Nano Lett., 2009, 9, 1260–1264 CrossRef CAS PubMed.
- T. Paik, T. R. Gordon, A. M. Prantner, H. Yun and C. B. Murray, Designing Tripodal and Triangular Gadolinium Oxide Nanoplates and Self-Assembled Nanofibrils as Potential Multimodal Bioimaging Probes, ACS Nano, 2013, 7, 2850–2859 CrossRef CAS PubMed.
- Y. C. Cao, Synthesis of Square Gadolinium-Oxide Nanoplates, J. Am. Chem. Soc., 2004, 126, 7456–7457 CrossRef CAS PubMed.
- T. Paik, D.-K. Ko, T. R. Gordon, V. Doan-Nguyen and C. B. Murray, Studies of Liquid Crystalline Self-Assembly of GdF3 Nanoplates by In-Plane, Out-of-Plane SAXS, ACS Nano, 2011, 5, 8322–8330 CrossRef CAS PubMed.
- Y.-P. Du, Y.-W. Zhang, Z.-G. Yan, L.-D. Sun and C.-H. Yan, Highly Luminescent Self-Organized Sub-2 nm EuOF Nanowires, J. Am. Chem. Soc., 2009, 131, 16364–16365 CrossRef CAS PubMed.
- X. Sun, Y.-W. Zhang, Y.-P. Du, Z.-G. Yan, R. Si, L.-P. You and C.-H. Yan, From Trifluoroacetate Complex Precursors to Monodisperse Rare-Earth Fluoride and Oxyfluoride Nanocrystals with Diverse Shapes through Controlled Fluorination in Solution Phase, Chem. – Eur. J., 2006, 13, 2320–2322 CrossRef PubMed.
- Z. Quan, D. Yang, P. Yang, X. Zhang, H. Lian, X. Liu and J. Lin, Uniform Colloidal Alkaline Earth Metal Fluoride Nanocrystals: Nonhydrolytic Synthesis and Luminescence Properties, Inorg. Chem., 2008, 47, 9509–9517 CrossRef CAS PubMed.
- Y.-P. Du, X. Sun, Y.-W. Zhang, Z.-G. Yan, L.-D. Sun and C.-H. Yan, Uniform Alkaline Earth Fluoride Nanocrystals with Diverse Shapes Grown from Thermolysis of Metal Trifluoroacetates in Hot Surfactant Solutions, Cryst. Growth Des., 2009, 9, 2013–2019 CrossRef CAS.
- N.-N. Dong, M. Pedroni, F. Piccinelli, G. Conti, A. Sbarbati, J. E. Ramírez-Hernández, L. M. Maestro, M. C. I.-d. l. Cruz, F. Sanz-Rodriguez, A. Juarranz, F. Chen, F. Vetrone, J. A. Capobianco, J. G. Solé, M. Bettinelli, D. Jaque and A. Speghini, NIR-to-NIR Two-Photon Excited CaF2: Tm3+,Yb3+ Nanoparticles: Multifunctional Nanoprobes for Highly Penetrating Fluorescence Bio-Imaging, ACS Nano, 2011, 5, 8665–8671 CrossRef CAS PubMed.
- M. J. Weber, Inorganic Scintillators: Today and Tomorrow, J. Lumin., 2002, 100, 35–45 CrossRef CAS.
- M. Nikl, Scintillation Detectors for X-Rays, Meas. Sci. Technol., 2006, 17, R37–R54 CrossRef CAS.
- C. Sun, G. Pratx, C. M. Carpenter, H. Liu, Z. Cheng, S. S. Gambhir and L. Xing, Synthesis and Radioluminescence of PEGylated Eu3+-doped Nanophosphors as Bioimaging Probes, Adv. Mater., 2011, 23, H195–H199 CAS.
- C. W. E. v. Eijk, Inorganic Scintillators in Medical Imaging, Phys. Med. Biol., 2002, 47, R85–R106 CrossRef PubMed.
- C. M. Carpenter, C. Sun, G. Pratx, R. Rao and L. Xing, Hybrid X-Ray/Optical Luminescence Imaging: Characterization of Experimental Conditions, Med. Phys., 2010, 37, 4011–4018 CrossRef CAS PubMed.
- W. Zheng, S. Zhou, Z. Chen, P. Hu, Y. Liu, D. Tu, H. Zhu, R. Li, M. Huang and X. Chen, Sub-10 nm Lanthanide-Doped CaF2 Nanoprobes for Time-Resolved Luminescent Biodetection, Angew. Chem., 2013, 125, 6803–6808 CrossRef.
- G. Chen, J. Shen, T. Y. Ohulchanskyy, N. J. Patel, A. Kutikov, Z. Li, J. Song, R. K. Pandey, H. Ågren, P. N. Prasad and G. Han, (α-NaYbF4:Tm3+)/CaF2 Core/Shell Nanoparticles with Efficient Near-Infrared to Near-Infrared Upconversion for High-Contrast Deep Tissue Bioimaging, ACS Nano, 2012, 6, 8280–8287 CrossRef CAS PubMed.
- C. Boukouvala, J. Daniel and E. Ringe, Approaches to modelling the shape of nanocrystals, Nano Converg., 2021, 8, 1–15 CrossRef PubMed.
- S. Brunauer, L. S. Deming, W. E. Deming and E. Teller, On a Theory of the van der Waals Adsorption of Gases, J. Am. Chem. Soc., 1940, 62, 1723–1732 CrossRef CAS.
- H. Liu, Q. Gong, Y. Yue, L. Guo and X. Wang, Sub-1 nm Nanowire Based Superlattice Showing High Strength and Low Modulus, J. Am. Chem. Soc., 2017, 139, 8579–8585 CrossRef CAS PubMed.
- B. Abécassis, M. D. Tessier, P. Davidson and B. Dubertret, Self-Assembly of CdSe Nanoplatelets into Giant Micrometer-Scale Needles Emitting Polarized Light, Nano Lett., 2014, 14, 710–715 CrossRef PubMed.
- R. A. Alvarez-Puebla, A. Agarwal, P. Manna, B. P. Khanal, P. Aldeanueva-Potel, E. Carbó-Argibay, N. Pazos-Pérez, L. Vigderman, E. R. Zubarev, N. A. Kotov and L. M. Liz-Marzán, Gold Nanorods 3D-Supercrystals as Surface Enhanced Raman Scattering Spectroscopy Substrates for the Rapid Detection of Scrambled Prions, Proc. Natl. Acad. Sci. U. S. A., 2011, 108, 8157–8161 CrossRef CAS PubMed.
- K.-J. Wu, K.-C. Chu, C.-Y. Chao, Y.-F. Chen, C.-W. Lai, C.-C. Kang, C.-Y. Chen and P.-T. Chou, CdS Nanorods Imbedded in Liquid Crystal Cells for Smart Optoelectronic Devices, Nano Lett., 2007, 7, 1908–1913 CrossRef CAS.
- Y. Yang, B. Wang, X. Shen, L. Yao, L. Wang, X. Chen, S. Xie, T. Li, J. Hu, D. Yang and A. Dong, Scalable Assembly of Crystalline Binary Nanocrystal Superparticles and Their Enhanced Magnetic and Electrochemical Properties, J. Am. Chem. Soc., 2018, 140, 15038–15047 CrossRef CAS PubMed.
- A. Dong, J. Chen, P. M. Vora, J. M. Kikkawa and C. B. Murray, Binary Nanocrystal Superlattice Membranes Self-Assembled at the Liquid–Air Interface, Nature, 2010, 466, 474–477 CrossRef CAS PubMed.
- T. Paik and C. B. Murray, Shape-Directed Binary Assembly of Anisotropic Nanoplates: A Nanocrystal Puzzle with Shape-Complementary Building Blocks, Nano Lett., 2013, 13, 2952–2956 CrossRef CAS PubMed.
- T. Paik, B. T. Diroll, C. R. Kagan and C. B. Murray, Binary and Ternary Superlattices Self-Assembled from Colloidal Nanodisks and Nanorods, J. Am. Chem. Soc., 2015, 137, 6662–6669 CrossRef CAS PubMed.
- G. Tessitore, G. A. Mandl, M. G. Brik, W. Park and J. A. Capobianco, Recent insights into upconverting nanoparticles: spectroscopy, modeling, and routes to improved luminescence, Nanoscale, 2019, 11, 12015–12029 RSC.
- J. Shan, M. Uddi, R. Wei, N. Yao and Y. Ju, The Hidden Effects of Particle Shape and Criteria for Evaluating the Upconversion Luminescence of the Lanthanide Doped Nanophosphors, J. Phys. Chem. C, 2010, 114, 2452–2461 CrossRef CAS.
- M. Kraft, C. Würth, V. Muhr, T. Hirsch and U. Resch-Genger, Particle-size-dependent upconversion luminescence of NaYF4:Yb,Er nanoparticles in organic solvents and water at different
excitation power densities, Nano Res., 2018, 11, 6360–6374 CrossRef CAS.
- C. Würth, M. Kaiser, S. Wilhelm, B. Grauel, T. Hirsch and U. Resch-Genger, Excitation power dependent population pathways and absolute quantum yields of upconversion nanoparticles in different solvents, Nanoscale, 2017, 9, 4283–4294 RSC.
- J. Zhao, Z. Lu, Y. Yin, C. McRae, J. A. Piper, J. M. Dawes, D. Jin and E. M. Goldys, Upconversion luminescence with tunable lifetime in NaYF4:Yb,Er nanocrystals: role of nanocrystal size, Nanoscale, 2013, 5, 944–952 RSC.
- F. Wang, J. Wang and X. Liu, Direct Evidence of a Surface Quenching Effect on Size-Dependent Luminescence of Upconversion Nanoparticles, Angew. Chem., Int. Ed., 2010, 49, 7456–7460 CrossRef CAS PubMed.
|
This journal is © the Partner Organisations 2024 |
Click here to see how this site uses Cookies. View our privacy policy here.