DOI:
10.1039/D3QI01952G
(Research Article)
Inorg. Chem. Front., 2024,
11, 246-254
Revealing the role of a unique local structure in lanthanide-doped Cs2LiInCl6 in boosting visible and NIR-II luminescence†
Received
24th September 2023
, Accepted 9th November 2023
First published on 10th November 2023
Abstract
Local structure engineering is one of the most useful strategies for tunable down-shifting and upconversion emissions in halide double perovskites (DPs). However, the roles of the local structure, including local symmetry, coordination structure, and active sites, in these DPs remain largely unexplored. Herein, we studied the local structure-correlated up/down-conversion luminescence in Yb3+/Er3+/Ho3+ co/tri-doped Cs2LiInCl6 with a unique local structure, which is different from its typical Cs2NaInCl6 counterpart. At optimal concentrations, the emission intensities of Cs2LiInCl6:Yb3+,Er3+ were 6 and 110 times higher than those of Cs2NaInCl6 at 552 and 1540 nm, respectively. The evolution of the local site symmetry calculated by the first-principles density functional theory demonstrates that the lower local symmetry of Cs2LiInCl6:Yb3+,Er3+ is responsible for luminescence enhancement at a low-doping level of Er3+. In addition, we verified that the concentration quenching effect can be effectively suppressed by two independent In3+ sites in Cs2LiInCl6, leading to increased visible and near-infrared (NIR-II) emissions close to the optimal concentration with higher Er3+ contents. Moreover, time-resolved photoluminescence (PL) measurements and steady-state rate equations are used to thoroughly understand the mechanism underlying the local-structure-dependent PL properties. Our results demonstrate that both the local symmetry breaking and presence of multiple independent active sites are beneficial for luminescence enhancement, and accordingly provide meaningful guidance for designing highly efficient visible and NIR-II emitters.
Introduction
Lead-free halide double perovskites (DPs) as promising luminescent materials have received increased interest from researchers for potential applications in various materials science and biomedical fields owing to their low toxicity and high chemical-, thermal-, and photo-stability.1–8 However, the photoluminescence (PL) performance is very poor for most DPs in view of the indirect bandgaps or parity-forbidden transitions, limiting their further practical photonic and biological applications. Fortunately, DPs have the general formula A2M+M3+X6 (where A = Rb+ and Cs+; M+ = Ag+ and Na+; M3+ = In3+ and Bi3+; and X = Cl− and Br−) that provides abundant opportunities for the material composition adjustment. For example, Luo et al. used Na+ for the partial substitution of Ag+ in Cs2AgInCl6, resulting in the break of the parity-forbidden transition.1 Similarly, Pei et al. found the Na+-induced breakdown of the local site symmetry in Cs2(Na/Ag)BiCl6 leading to an obviously improved near-infrared (NIR) luminescence.9 In particular, lanthanide (Ln) elements are generally suitable for incorporation into DPs for favorable optical properties owing to the same valence state as that of In3+ or Bi3+, proper octahedral coordination environment, and special ladder-like 4f electronic states. Consequently, DP hosts with Ln-ion-doping often display enhanced visible or NIR emissions with sharp peaks.10–13
The second near-infrared (NIR-II, 1000–1700 nm) spectral region has shown great application prospects in NIR-based lighting devices, biosensing, optical fiber communication, high-resolution imaging, information storage, etc.14–18 Among Ln ions, Er3+ has a good spectral response of approximately 1540 nm with a relatively large absorption cross-section and is therefore commonly used as either an NIR-II sensitizer in up-conversion (UC) materials or NIR-II emitting center in down-conversion (DC) systems. Unfortunately, the photoluminescence quantum yields (PLQY) of Er-doping UC/DC materials are still low because of the lack of appropriate host materials or powerful design strategies. To date, one of the most useful strategies for tunable down-shifting and upconversion emissions is local structure engineering.13,19,20 For example, Er3+–Sb3+ co-doped Cs2NaInCl6 has been demonstrated as a potential host for 1540 nm emission by partially alleviating the parity-forbidden rule within 4f intraconfigurational transitions of Er3+.10 However, most of the previous works proved that the visible and NIR-II emission enhancement cannot only be attributed to the change of the local structure as the synergistic effect of the improved crystallization also works.1,9,10,21 The role of the local structure alone, such as local symmetry, coordination structure, and interionic distance, in these DPs, remains largely unexplored. Therefore, it requires in-depth corroboration of the exact effect of the local structure on rational modulation of both visible and NIR emissions in the UC/DC systems.
In this work, we have, for the first time, synthesized lead-free Yb3+/Er3+/Ho3+ co/tri-doped Cs2LiInCl6 double perovskite with a unique local structure, including low unit cell symmetry (trigonal), two independent In3+ active sites, proper In3+ separation distance, and large crystal-field strength. By comparing with the conventional Cs2NaInCl6 counterpart, we provide direct evidence that local symmetry breaking and the presence of multiple active sites are two priority factors for emission enhancement and elucidate the local-structure-correlated up/down-conversion luminescence in terms of emission intensity, transition selectivity, wavelength shift, and lifetime.
Results and discussion
We selected Cs2LiInCl6, a double perovskite, as a prototype to uncover the role of local structure, because of its different local structure compared with the commonly used DPs, such as Cs2AgInCl6, Cs2NaBiCl6, and Cs2NaInCl6 (in our study for comparison). Meanwhile, other factors, including phonon energy, crystallization quality, and crystal size, can be excluded, because they are very similar for both Cs2NaInCl6 and Cs2LiInCl6. Both Cs2LiInCl6 and Cs2NaInCl6 DPs were synthesized using a solid-state method (Fig. S1†). As shown in Fig. 1a, the unique local structure of Cs2LiInCl6 DP has two points: one is the trigonal-phase crystallographic structure compared with the cubic-phase structure of Cs2NaInCl6, and the second is that Cs2LiInCl6 has two independent In3+ active sites (In1 and In2) with a lower site symmetry of D3d, whereas Cs2NaInCl6 has only one In3+ active site with the highest site symmetry of Oh.6 The distances of In1–In1 (or In2–In2) and In1–In2 are 7.3184 and 7.3712 Å, respectively, confirming that the two In sites are not crystallographically equivalent. The difference could allow us to thoroughly understand the mechanism underlying the local-structure-dependent PL properties. As a proof of concept, Yb3+/Er3+/Ho3+ ions were doped into the host for both upconversion luminescence and NIR emissions. As shown in the X-ray diffraction (XRD) patterns in Fig. 1b, it was found that almost all the main peaks match well with the standard pattern of Cs2LiInCl6 (PDF#79-0775), indicating the retaining trigonal phase. We then carefully compared the XRD results with the standard pattern of Cs2LiInCl6, and found that there are some impurity peaks belonging to Cs2InCl5(H2O), due to the easy hygroscopicity of Li-based materials.22 Fortunately, such impurities have no impact on our results. In addition, the peak positions shifted toward a smaller angle, as a result of lattice expansion by the substitution of In3+ by Yb3+, Er3+, and/or Ho3+ with larger ionic radii according to Bragg's law. In a Yb–Er coupled system, Yb3+ is widely used as the sensitizer for 980 nm light, and enables efficient energy transfer to Er3+ (Fig. 1c). Therefore, green and 1540 nm NIR-II emissions can be obtained simultaneously under 980 nm excitation. The scanning electron microscopy (SEM) images show that the products are micro-sized crystals with uniform distribution of all the elements of Cs, In, Cl, Yb, Er, and Ho using energy dispersive X-ray spectroscopy (EDS mappings in Fig. 1d). The actual doping content of lanthanide ions in Cs2LiInCl6 was determined by EDS and the induced coupled plasma optical emission spectrometry, as shown in Table S1 and Fig. S2.†
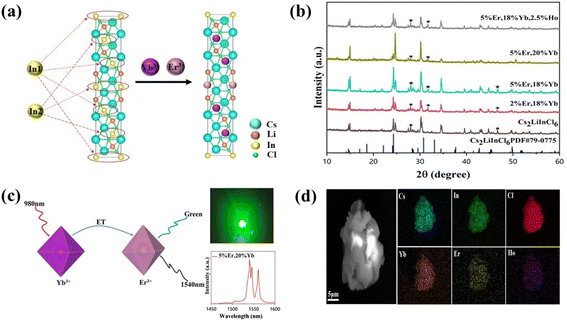 |
| Fig. 1 (a) The crystalline structure of trigonal-phase Cs2LiInCl6 with two independent In3+ active sites: In1 and In2. (b) XRD patterns of Cs2LiInCl6 and Yb3+/Er3+/Ho3+ co/tri-doped Cs2LiInCl6 DPs. * are the impurity peaks of Cs2InCl5(H2O). (c) A schematic illustration of the energy transfer process from Yb3+ to Er3+ for the green UC emission and 1540 nm DC luminescence. (d) The SEM image and EDS distribution of the elements in Yb3+/Er3+/Ho3+ tri-doped Cs2LiInCl6. | |
For a better understanding of the local environment, Raman spectroscopic measurements were performed. According to the factor group analysis (Raman and hyper-Raman scattering database on the Bilbao Crystallographic Server, cryst.ehu.es), the following number of Raman modes can be found for Cs2LiInCl6, ΓRaman = 7A1g + 9Eg. Although all these modes are Raman-active, there are only three main Raman modes, as shown in Fig. 2a. Comparing with Cs2NaInCl6 DP,6,10 the lowest frequency mode (∼174 cm−1) of pure Cs2LiInCl6 belongs to the Eg mode, due to the asymmetric stretching of In–Cl bonds in the octahedral cages, and the other two frequency modes of 289 and 321 cm−1 can be attributed to the A1g mode related to the octahedrons. After Yb/Er co-doping, both A1g and Eg modes were slightly shifted to longer wavenumbers due to the sublattice distortion of the octahedrons. The UV-vis absorption spectra show that the host absorption band edge located at 235 nm is almost unchanged after doping with lanthanide ions (Fig. 2b). Some new strong absorption in the 270–550 nm could be associated with the presence of Yb and Er. The optical band gaps of Cs2LiInCl6 and Cs2NaInCl6 DPs were evaluated to be 4.7 and 4.9 eV, respectively (Fig. S3†). The calculated electronic bandgaps were approximately 3.2 and 2.9 eV (Fig. S4†), respectively, which were underestimated using the first-principles method. Thermogravimetric analysis (TGA) was also conducted for both pure and Yb/Er co-doped Cs2LiInCl6 samples. As shown in Fig. 2c, there were two stages of mass loss, 100 °C and 540 to 560 °C. The mass change at 100 °C should be ascribed to the desorption of physically or chemically absorbed H2O, because of the easy hydration of Li-based materials. The mass loss in the high-temperature range indicates that the samples begin to decompose gradually. These results indicate that the thermal stability of Cs2LiInCl6 DP is as high as those of other halide double perovskites. Fig. 2d shows the UC and NIR emission spectra of Cs2LiInCl6 DP with different doping concentrations under 980 nm excitation. Three emission peaks, located at 526, 552, and 669 nm, can be assigned to 2H11/2, 4S3/2, and 4F9/2 to 4I15/2 transitions of Er3+. The short-wave infrared emission shows multiple peak features at 1540, 1546, and 1561 nm with ultra-narrowband full width at half maximum (FWHM) of 6 nm, corresponding to the 4I13/2 → 4I15/2 transition of Er3+.
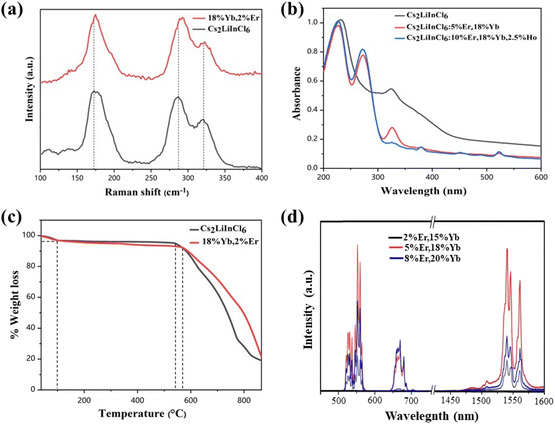 |
| Fig. 2 (a) Raman spectra of Cs2LiInCl6 and Cs2LiInCl6:18%Yb3+,2%Er3+. (b) Absorbance spectra of Cs2LiInCl6, Cs2LiInCl6:18%Yb3+,5%Er3+ and Cs2LiInCl6:18%Yb3+,10%Er3+,2.5%Ho3+. (c) TGA results of Cs2LiInCl6 and Cs2LiInCl6:18%Yb3+,2%Er3+. (d) PL spectra of Cs2LiInCl6:15%Yb3+,2%Er3+, Cs2LiInCl6:18%Yb3+,5%Er3+, and Cs2LiInCl6:20%Yb3+,8%Er3+. | |
In principle, a high dopant concentration will definitely benefit the overall brightness of UC and NIR emissions. However, the concentration quenching effect of lanthanide ions always limits their optimal concentration.13,23 To determine the optimal concentrations of Cs2NaInCl6 and Cs2LiInCl6 DPs, the emission intensities of both visible and NIR regions were measured as a function of the total doping content (% Yb3+ + % Er3+). The comparison can be clearly seen in Fig. 3a and b. At a relatively low doping level of Er3+ (blue region at 552 nm), the optimal concentration of Cs2NaInCl6 DP was 18% Yb3+ + 2% Er3+, while the intensity of Cs2LiInCl6 was 3 times stronger than that of Cs2NaInCl6. At a high doping concentration of Er3+ (yellow region), the concentration quenching effect inevitably occurred, leading to decreased emission intensity for Cs2NaInCl6. Whereas the trend was different for Cs2LiInCl6, the UC luminescence can be amplified continuously until reaching the maximum intensity at 18% Yb3+ + 5% Er3+. At this time, Cs2LiInCl6 DP was nearly 6 times brighter than Cs2NaInCl6 DP with the highest concentration of Er3+. The DC emissions at 1540 nm showed similar trends for both Cs2NaInCl6 and Cs2LiInCl6 DPs. The optimal concentration of Cs2NaInCl6 was 15% Yb3+ + 5% Er3+, in comparison, with a higher optimal concentration for Cs2LiInCl6 was 15% Yb3+ + 10% Er3+ (Fig. 3b). Meanwhile, the enhancement factors were 90 times and 110 times at these two concentrations, respectively. Fig. 3c and d show the compared PL spectra of the UC and NIR emissions of Cs2NaInCl6 and Cs2LiInCl6 DPs at the corresponding optimal concentrations of Cs2LiInCl6. The origin of luminescence enhancement is highly desirable for study in detail. Besides, the larger optimal concentration for Cs2LiInCl6 indicates that the concentration quenching effect can be effectively suppressed, thus resulting in further increased emission, which deserves to be explored further for designing highly efficient DP-based emitters.
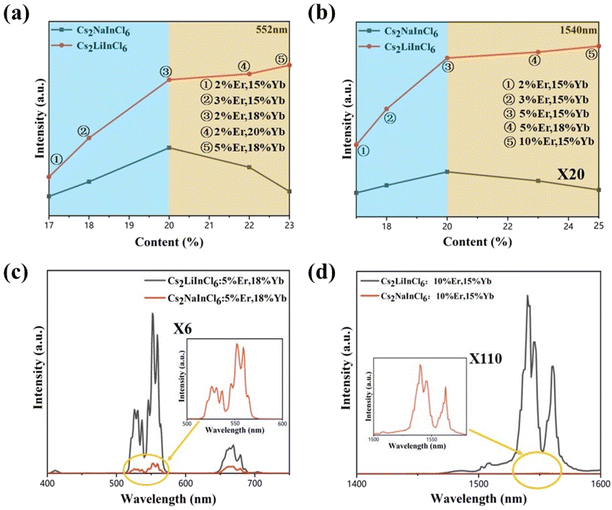 |
| Fig. 3 (a) The UC emission intensity of Cs2NaInCl6 and Cs2LiInCl6 DPs as a function of the total doping content. (b) The DC emission intensity of Cs2NaInCl6 and Cs2LiInCl6 DPs as a function of the total doping content. (c) and (d) PL spectra of Cs2NaInCl6 and Cs2LiInCl6 DPs at the corresponding optimal concentrations of Cs2LiInCl6. | |
To explore the difference in the luminescence enhancement between Cs2NaInCl6 and Cs2LiInCl6 DPs, we first used first-principles density functional theory (DFT) calculations to track the evolution of local site symmetry at different doping concentrations (Fig. 4). At a low doping concentration of Er3+, when Yb3+ and Er3+ ions are doped into the In lattice of Cs2NaInCl6, the local site symmetry will be reduced from Oh to D2h, whereas the local site symmetry of Cs2LiInCl6 will descend from D3d to C3v. According to the branching rules,24 Cs2LiInCl6 should have a lower symmetry than that of Cs2NaInCl6. On the basis of the Judd–Ofelt (J–O) theory,25 the probability of spontaneous radiative electric dipole (ED) transitions can be expressed as:
| 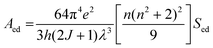 | (1) |
where
e is the electron charge,
λ is the mean wavelength,
n is the refractive index, and
h is the Planck's constant. The electric-dipole line strength
Sed is given by
eqn (2):
|  | (2) |
where
Ωt (
t = 2, 4, 6) are J–O intensity parameters, which depend on the local environment and symmetry around the Ln
3+ ions. That means breaking the local site symmetry is a useful strategy to increase the ED transitions of rare-earth ions. Several previous studies have demonstrated that the lower symmetry usually contributes to the larger
Ωt,
26–28 and therefore leads to high probabilities of radiative ED transitions, 552 nm visible and 1540 nm NIR-II emissions in our case. Hence, considering the lower local site symmetry of Cs
2LiInCl
6, it is not surprising that the superior luminescence intensity can be obtained for Cs
2LiInCl
6. The increased distortion of Yb
3+ and Er
3+ sites in Cs
2LiInCl
6 should cause the observed luminescence enhancement in both low-doping and high-doping levels of Er
3+, as seen in the blue and yellow regions in
Fig. 3a and b. At a high doping level, namely close to the optimal concentration of Cs
2LiInCl
6, the local site symmetry of Cs
2LiInCl
6 maintained the same as
C3v, whereas the symmetry of Cs
2NaInCl
6 will increase from
D2h to
D4h. Consequently, the effect of the site symmetry still works, and much larger enhancement factors for NIR-II emission in Cs
2LiInCl
6 mean that local symmetry breaking can bring a greater impact on NIR emission than visible luminescence.
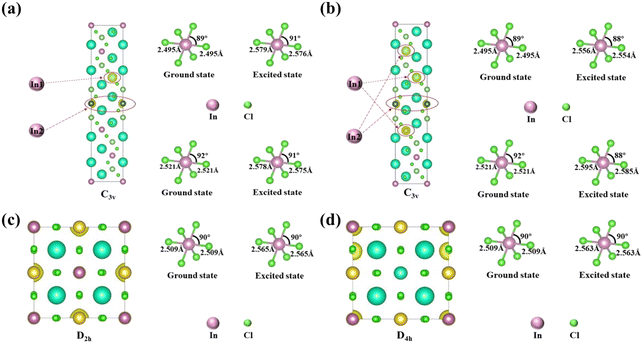 |
| Fig. 4 (a and b) The calculated local site symmetry and sublattice distortions of Cs2LiInCl6:Yb3+,Er3+ at the low and high doping levels, respectively. (c and d) The calculated local site symmetry and sublattice distortions of Cs2NaInCl6: Yb3+,Er3+ at the low and high doping levels, respectively. | |
The PL decay curves of Cs2LiInCl6:18%Yb3+,5%Er3+, and Cs2NaInCl6:18%Yb3+,5%Er3+ for both visible and NIR emissions were then measured (Fig. 5a and b). It is interesting that Cs2LiInCl6:18%Yb3+,5%Er3+ has a shortened green emission lifetime (2.73 ms) compared to its Cs2NaInCl6 counterpart (4.23 ms). The PL decay of Cs2NaInCl6:18%Yb3+,5%Er3+ can be fitted by three components: a fast lifetime of τ1: 0.67 ms, a middle lifetime of τ2: 2.54 ms, and a slow lifetime of τ3: 8.55 ms (Table S2†); while the PL decay of Cs2LiInCl6:18%Yb3+,5%Er3+ is fitted by a mono-exponential function with a slow lifetime of 2.73 ms (Fig. 5a). The measured lifetime (τ) in the PL decay curves is connected to the radiative (τr) and nonradiative lifetimes (τnr) from the relation 1/τ = 1/τr + 1/τnr. On one hand, distortions of the local symmetry would facilitate electronic transitions, and increase the radiative transition rate (kr = 1/τr); on the other hand, the improved crystallization results in a reduction of the number of defects for less nonradiative relaxation, leading to a prolonged emission lifetime (τnr). Thus, if the main contribution of emission enhancement comes from the improved crystallization, the measured average τ should be longer for Cs2LiInCl6 than Cs2NaInCl6. Instead, Cs2LiInCl6 showed a shortened lifetime, indicating that the radiative transition rate kr plays a predominant role in luminescence, directly suggesting that the main contribution of the emission enhancement comes from the breaking of local symmetry. The shortened slow lifetime of Cs2LiInCl6:18%Yb3+,5%Er3+ is also consistent with DFT results, due to lower local symmetry. Based on the fitting results, we also infer that the cross-relaxation process can be effectively suppressed in Cs2LiInCl6 compared to Cs2NaInCl6 with high-doping concentrations of Er3+. Furthermore, we investigated the decay lifetimes of the 4I13/2 state (1540 nm) under 980 nm excitation. The 4I13/2 lifetime of Cs2LiInCl6:18%Yb3+,5%Er3+ is also fitted by a mono-exponential function, which is much longer than that of the Cs2NaInCl6 counterpart (Fig. 5b and Table S3†), indicating that the nonradiative decay processes are largely reduced.
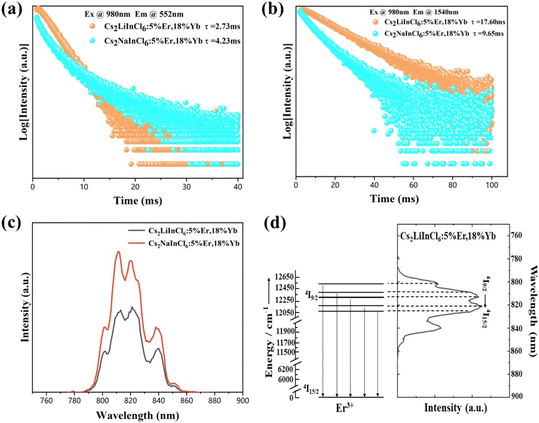 |
| Fig. 5 (a and b) The PL decay curves of Cs2LiInCl6:18%Yb3+,5%Er3+ and Cs2NaInCl6:18%Yb3+,5%Er3+ for both visible and NIR emissions under 980 nm excitation. (c) High-resolution PL spectra of Cs2LiInCl6:18%Yb3+,5%Er3+ and Cs2NaInCl6:18%Yb3+,5%Er3+ for the 4I9/2 → 4I15/2 transition of Er3+. (d) The energy level splitting of Cs2LiInCl6:18%Yb3+,5%Er3+ from the 4I9/2 crystal field. | |
Basically, the emission wavelength of Ln3+ shows almost no shift, because of the shield of the 4f energy levels by exterior shells. However, as shown in Fig. 5c, both Cs2LiInCl6 and Cs2NaInCl6 DPs have the same crystal-field strength, and the center wavelength of Cs2LiInCl6 slightly shifts to 820 nm compared with 813 nm of Cs2NaInCl6. The emission band at approximately 800 nm belongs to the 4I9/2 → 4I15/2 transition of Er3+,29 and the 4I9/2 level can split into 5 stark levels as long as the crystal field symmetry is lower than the cubic symmetry.30 The large difference in the 4I9/2 energy level between Cs2LiInCl6 DP and commercial NaYF4 nanoparticles (808 nm) supports that the 4I9/2 intermediate state could be a good indicator of the crystal-field environment. Fig. 5d exhibits the energy level splitting of Cs2LiInCl6:18%Yb3+,5%Er3+ from the 4I9/2 crystal field. The crystal-field strength of Er3+ from the 4I9/2 crystal field was calculated to be 400 cm−1 for Cs2LiInCl6 and Cs2NaInCl6 (Fig. S5†), demonstrating that the 4I9/2 crystal field is insensitive to the variation in local symmetry.31 On the basis of a similar coordination environment, the wavelength shift in the 4I9/2 state should be attributed to the population redistribution among the crystal-field-split manifolds with thermal fluctuations. Meanwhile, Cs2LiInCl6 shows excellent transition selectivity under 1550 nm excitation. A record green-to-red ratio of up to 281 can be seen in Fig. S6† for Cs2LiInCl6:18%Yb3+,10%Er3+,2.5%Ho3+, suggesting that Cs2LiInCl6 is a good candidate for single-band UC applications.32 Fig. S6 and S7† prove that the high green-to-red ratio is closely related to the excitation wavelength because of different UC populating mechanisms, further confirming the weak electron–phonon coupling in Cs2LiInCl6.
To obtain a deep insight into the boosting mechanism, pump-power-dependent PL measurements were performed for visible and NIR emissions. As shown in Fig. 6a, the slopes are fitted to 2.49, 2.32, and 3.10 for 2H11/2, 4S3/2, and 4F9/2 emissions, respectively, following the two-photon and three-photon conversion mechanisms. The general UC mechanism under 980 nm excitation is depicted in Fig. 6b. The three-photon process could increase the cross-relaxation (CR) rate at a high doping level of Er3+, leading to an intensified output of red emission. Considering that the 4I9/2 state is closely related to other excited states of Er3+, it is desirable to investigate the relationship of this emission band with the cross-relaxation process.33–35 A set of rate equations were established based on the UC process, as shown in Fig. 6b:
|  | (3) |
|  | (4) |
|  | (5) |
where
N0,
N1,
N2, and
N3 are the population densities of
4I
15/2,
4I
13/2,
4I
11/2, and
4I
9/2 states of Er
3+, respectively.
β1,
β2, and
β3 are the non-radiative rates of
4I
13/2,
4I
11/2, and
4I
9/2 states of Er
3+.
A1,
A2, and
A3 are the radiative rates of
4I
13/2,
4I
11/2, and
4I
9/2 levels of Er
3+, respectively.
T2 denotes the possible thermal pumping rate from the
4I
11/2 to
4I
9/2 state.
W1,
W2, and
W3 are the energy transfer rates from the
2F
5/2 state of Yb
3+ to the
4F
9/2,
4I
11/2, and
4F
5/2 levels of Er
3+.
C1 represents the cross-relaxation process of
4I
13/2 +
4I
13/2 →
4I
15/2 +
4I
9/2 (CR3). The CR4 process can be omitted due to less contribution of
4I
9/2 →
4S
3/2. In
eqn (5), the term
T2N2 is commonly ignored at room temperature. In such case, the following expression is obtained:
| 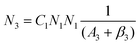 | (6) |
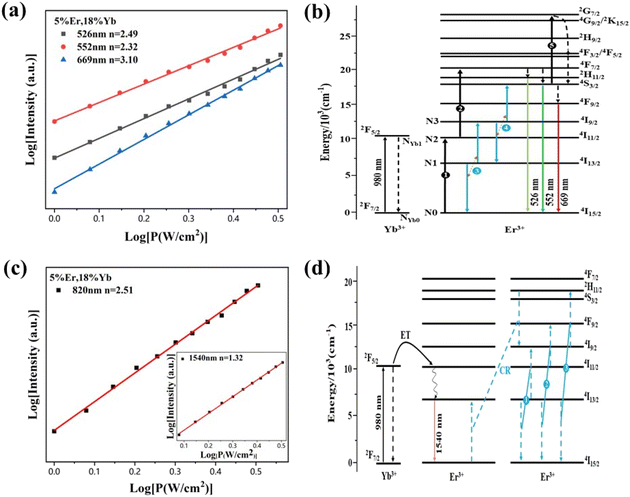 |
| Fig. 6 (a) The power density dependence of Er3+ emissions of 2H11/2 (526 nm), 4S3/2 (552 nm), and 4F9/2 (669 nm) for Cs2LiInCl6:18%Yb3+,5%Er3+. (b) UC emission mechanism of Cs2LiInCl6:Yb3+,Er3+ under 980 nm excitation. (c) The power density dependence of the Er3+ emission of 4I9/2 (820 nm) for Cs2LiInCl6:18%Yb3+,5%Er3+. The inset shows the power density dependence of 1540 nm emission under 980 nm excitation. (d) The NIR emission mechanism of Cs2LiInCl6: Yb3+,Er3+ under 980 nm excitation. | |
From eqn (6), we can observe that N3∞N12∞P2, indicating that the two NIR photons at 980 nm are needed to populate the 4I9/2 excited state, which is in accordance with the pump-power dependent PL result in Fig. 6c. As a result of the intrinsic low phonon energy (320 cm−1) and weak electron–phonon coupling for Cs2LiInCl6 DP, much weaker non-radiative transition from 4I9/2 → 4I13/2 can be neglected (β3), the intensity of the 4I9/2 state (I820) can be derived from eqn (6):
We then obtain I820∞C1. As the intensity of Cs2NaInCl6 is 3-fold higher than that of Cs2LiInCl6 (Fig. 5c), we can conclude that the CR rate of Cs2LiInCl6 is 3 times weaker than the Cs2NaInCl6 counterpart, demonstrating that the concentration quenching effect can be effectively suppressed for Cs2LiInCl6 at a high-doping level of Er3+. We infer that the distribution of Er3+ is statistical onto the two In3+ sites, and the distance of two adjacent Er3+ ions can be directly increased in Cs2LiInCl6, blocking the CR process at a high doping level of Er3+ (Fig. S8†).36–40 Thus, the higher tolerance of Er3+ content in Cs2LiInCl6 is definitely beneficial to the UC emission, consistent with the results in Fig. 3a. The NIR-II emission originated from an energy transfer from Yb3+ to Er3+ because the emission intensity of 4I13/2 shows a linear relationship with the pump power of 980 nm laser (the inset of Fig. 6c).1 Together with the PL decay and PL results shown in Fig. 3b and 5b, respectively, we suppose that the Er3+–Er3+ energy transfer is the main nonradiative channel, and the presence of the two independent In3+ sites in Cs2LiInCl6 can decrease undesirable nonradiative energy transfer (CR1, CR2, and CR3 in Fig. 6d), accounting for the obvious NIR-II luminescence enhancement.
Conclusions
In summary, we provided a comprehensive understanding of the exact effect of local structure on both down-shifting and upconversion emissions in double perovskites by means of the successful synthesis of Cs2LiInCl6. Taking advantage of its unique local structure, including low local symmetry (trigonal), two In3+ active sites, and large crystal-field strength, Cs2LiInCl6:Yb3+ and Er3+ show 6-fold and 110-fold emission enhancement compared with Cs2NaInCl6 counterpart at 552 and 1540 nm, respectively. Experimental results and theoretical calculations directly demonstrate that local symmetry breaking and the presence of multiple independent active sites are very important for luminescence enhancement. Our results provide a novel optical design through the engineering of local structures for desired luminescent properties of double perovskites in both visible and NIR-II regions.
Conflicts of interest
There are no conflicts to declare.
Acknowledgements
The work was financially supported by the National Natural Science Foundation of China-Yunnan Joint Fund (U1902222), the National Natural Science Foundation of China (12064021 and 51862020), and the Foundation of Yunnan Province (202001AT070037, 202101AT070104 and 202101AT070097), and Yunnan Major Scientific and Technological Projects (grant no. 202202AG050016). The authors would like to thank Kang Jiao from Shiyanjia Lab (https://www.shiyanjia.com) for the SEM analysis.
References
- J. Luo, X. Wang, S. Li, J. Liu, Y. Guo, G. Niu, L. Yao, Y. Fu, L. Gao, Q. Dong, C. Zhao, M. Leng, F. Ma, W. Liang, L. Wang, S. Jin, J. Han, L. Zhang, J. Etheridge, J. Wang, Y. Yan, E. H. Sargent and J. Tang, Efficient and stable emission of warm-white light from lead-free halide double perovskites, Nature, 2018, 563, 541–545 CrossRef CAS PubMed.
- P. Saghy, A. M. Brown, C. Chu, L. C. Dube, W. Zheng, J. R. Robinson and O. Chen, Lanthanide double perovskite nanocrystals with emissions covering the UV-C to NIR spectral range, Adv. Opt. Mater., 2023, 11, 2300277 CrossRef CAS.
- Y. Liu, A. Nag, L. Manna and Z. Xia, Lead-free double perovskite Cs2AgInCl6, Angew. Chem., Int. Ed., 2021, 60, 11592–11603 CrossRef CAS PubMed.
- X. Xu, P. Han, D. Zheng, K. Du, C. Li, F. Liu, R. Zhang and K. Han, Boosting the upconversion and near-infrared emission via alloying Bi3+ in Cs2NaErCl6 double perovskite, Laser Photonics Rev., 2022, 16, 2200318 CrossRef CAS.
- S. Jin, R. Li, H. Huang, N. Jiang, J. Lin, S. Wang, Y. Zheng, X. Chen and D. Chen, Compact ultrabroadband light-emitting diodes based on lanthanide-doped lead-free double perovskites, Light: Sci. Appl., 2022, 11, 52 CrossRef CAS PubMed.
- Z. Rao, Z. Li, X. Zhao and X. Gong, Targeted high-precision up-converting thermometer platform over multiple temperature zones with Er3+, Mater. Horiz., 2023, 10, 1816–1824 RSC.
- F. Igbari, Z.-K. Wang and L.-S. Liao, Progress of lead-free halide double perovskites, Adv. Energy Mater., 2019, 9, 1803150 CrossRef.
- P. Han, X. Zhang, C. Luo, W. Zhou, S. Yang, J. Zhao, W. Deng and K. Han, Manganese-doped, lead-free double perovskite nanocrystals for bright orange-red emission, ACS Cent. Sci., 2020, 6, 566–572 CrossRef CAS PubMed.
- Y. Pei, D. Tu, C. Li, S. Han, Z. Xie, F. Wen, L. Wang and X. Chen, Boosting near-infrared luminescence of lanthanide in Cs2AgBiCl6 double perovskites via breakdown of the local site symmetry, Angew. Chem., Int. Ed., 2022, 61, e202205276 CrossRef CAS PubMed.
- S. Saikia, A. Joshi, H. Arfin, S. Badola, S. Saha and A. Nag, Sb3+–Er3+-codoped Cs2NaInCl6 for emitting blue and short-wave infrared radiation, Angew. Chem., Int. Ed., 2022, 61, e202201628 CrossRef CAS PubMed.
- Y. Shi, X. Zhang, X. Wang and Y. Zhang, Pure green upconversion from a multicolor downshifting perovskite crystal, Adv. Opt. Mater., 2023, 11, 2202704 CrossRef CAS.
- M. N. Tran, I. J. Cleveland, J. R. Geniesse and E. S. Aydil, High photoluminescence quantum yield near-infrared emission from a lead-free ytterbium-doped double perovskite, Mater. Horiz., 2022, 9, 2191–2197 RSC.
- H. Arfin, J. Kaur, T. Sheikh, S. Chakraborty and A. Nag, Bi3+-Er3+ and Bi3+-Yb3+ codoped Cs2AgInCl6 double perovskite near-infrared emitters, Angew. Chem., 2020, 132, 11403–11407 CrossRef.
- B. Zhou, L. Yan, J. Huang, X. Liu, L. Tao and Q. Zhang, NIR-II-responsive photon upconversion through energy migration in an ytterbium sublattice, Nat. Photonics, 2020, 14, 760–766 CrossRef CAS.
- X. Cheng, Y. Pan, Z. Yuan, X. Wang, W. Su, L. Yin, X. Xie and L. Huang, Er3+ sensitized photon upconversion nanocrystals, Adv. Funct. Mater., 2018, 28, 1800208 CrossRef.
- X. Feng, L. Lin, R. Duan, J. Qiu and S. Zhou, Transition metal ion activated near-infrared luminescent materials, Prog. Mater. Sci., 2022, 129, 100973 CrossRef CAS.
- G. Chen, T. Y. Ohulchanskyy, A. Kachynski, H. Agren and P. N. Prasad, Intense visible and near-infrared upconversion photoluminescence in colloidal LiYF4:Er3+ nanocrystals under excitation at 1490 nm, ACS Nano, 2011, 5, 4981–4986 CrossRef CAS PubMed.
- X. Lei, R. Li, D. Tu, X. Shang, Y. Liu, W. You, C. Sun, F. Zhang and X. Chen, intense near-infrared-II luminescence from NaCeF4: Er/Yb nanoprobes for in vitro bioassay and in vivo bioimaging, Chem. Sci., 2018, 9, 4682–4688 RSC.
- H. Dong, L.-D. Sun and C.-H. Yan, Local structure engineering in lanthanide-doped nanocrystals for tunable upconversion emissions, J. Am. Chem. Soc., 2021, 143, 20546–20561 CrossRef CAS PubMed.
- H. Fu, P. Peng, R. Li, C. Liu, Y. Liu, F. Jiang, M. Hong and X. Chen, A general strategy for tailoring upconversion luminescence in lanthanide-doped inorganic nanocrystals through local structure engineering, Nanoscale, 2018, 10, 9353–9359 RSC.
- D. Avram, B. Cojocaru, I. Tiseanu, M. Florea and C. Tiseanu, Down-/up-conversion emission enhancement by Li addition: improved crystallization or local structure distortion, J. Phys. Chem. C, 2017, 121, 14274–14284 CrossRef CAS.
- Q. Ge, R. Zheng, J. Lin and H. Chen, Cs2InCl5(H2O): A moisture-stable defective double halide perovskite analogue with broadband emission, Mater. Lett., 2020, 277, 128280 CrossRef CAS.
- L. Yan, B. Zhou, N. Song, X. Liu, J. Huang, T. Wang, L. Tao and Q. Zhang, Self-sensitization induced upconversion of Er3+ in core–shell nanoparticles, Nanoscale, 2018, 10, 17949–17957 RSC.
- D. Tu, Y. Liu, H. Zhu, R. Li, L. Liu and X. Chen, Breakdown of crystallographic site symmetry in lanthanide-doped NaYF4 crystals, Angew. Chem., Int. Ed., 2013, 52, 1128–1133 CrossRef CAS PubMed.
- B. R. Judd, Optical absorption intensities of rare-earth ions, Phys. Rev., 1962, 127, 750–761 CrossRef CAS.
- J. Hao, Y. Zhang and X. Wei, Electric-induced enhancement and modulation of upconversion photoluminescence in epitaxial BaTiO3: Yb/Er thin films, Angew. Chem., Int. Ed., 2011, 50, 6876–6880 CrossRef CAS PubMed.
- Y. Wang, X. Wang, Y. Mao and J. A. Dorman, Impact of Sc3+-modified local site symmetries on Er3+ ion upconversion luminescence in Y2O3 nanoparticles, J. Phys. Chem. C, 2022, 126, 11715–11722 CrossRef CAS PubMed.
- S. Tanabe, T. Ohyagi, N. Soga and T. Hanada, Compositional dependence of Judd-Ofelt parameters of Er3+ ions in alkali-metal borate glasses, Phys. Rev. B: Condens. Matter Mater. Phys., 1992, 46, 3305–3310 CrossRef CAS PubMed.
- E. M. Chan, E. S. Levy and B. E. Cohen, Rationally designed energy transfer in upconverting nanoparticles, Adv. Mater., 2015, 27, 5753–5761 CrossRef CAS PubMed.
- P. A. Loiko, E. V. Vilejshikova, N. M. Khaidukov, M. N. Brekhovskikh, X. Mateos, M. Aguilo and K. V. Yumashev, Judd–Ofelt modeling, stimulated-emission cross-sections and non-radiative relaxation in Er3+: K2YF5 crystals, J. Lumin., 2016, 180, 103–110 CrossRef CAS.
- A. Li, D. Xu, H. Lin, L. Yao, S. Yang, Y. Shao, Y. Zhang and Z. Chen, A novel anion doing strategy to enhance upconversion luminescence in NaGd(MoO4)2:Yb3+/Er3+ nanophosphors, Phys. Chem. Chem. Phys., 2017, 19, 15693–15700 RSC.
- L. Lei, J. Zhang and S. Xu, The progress of single-band upconversion nanomaterials, RSC Adv., 2016, 6, 81076–81084 RSC.
- L. Li, F. Qin, Y. Zhou, J. Mao and Z. Zhang, Origin of the giant thermal enhancement of the Er3+ ion's 4I9/2-4I15/2 photoluminescence, Spectrochim. Acta, Part A, 2020, 229, 117862 CrossRef CAS PubMed.
- X. Yin, W. Xu, G. Zhu, Y. Ji, Q. Xiao, X. Dong, M. He, B. Cao, N. Zhou, X. Luo, L. Guo and B. Dong, Towards highly efficient NIR II response upconversion phosphor enabled by long lifetimes of Er3+, Nat. Commun., 2022, 13, 6549 CrossRef CAS PubMed.
- L. Peng, F. Qin, C. Wang, L. Li and Z. Zhang, A high-precision thermometry strategy by replacing the infrared with visible light for detection, Opt. Lett., 2023, 48, 4061–4064 CrossRef CAS PubMed.
- C. Ma, X. Xu, F. Wang, Z. Zhou, D. Liu, J. Zhao, M. Guan, C. I. Lang and D. Jin, Optical sensitizer concentration in single upconversion nanocrystals, Nano Lett., 2017, 17, 2858–2864 CrossRef CAS PubMed.
- A. Aebischer, M. Hostettler, J. Hauser, K. Kramer, T. Weber, H. U. Gudel and H.-B. Burgi, Structural and spectroscopic characterization of active sites in a family of light-emitting sodium lanthanide tetrafluorides, Angew. Chem., Int. Ed., 2006, 45, 2802–2806 CrossRef CAS PubMed.
- Y.-X. Yang, Z. Xu, S.-L. Zhao, Z.-Q. Liang, W. Zhu and J.-J. Zhang, Shape controllable synthesis and enhanced upconversion photoluminescence of β-NaYF4:Yb3+ and Er3+ nanocrystals by introducing Mg2+, Chin. Phys. B, 2017, 26, 087801 CrossRef.
- E. M. Chan, D. J. Gargas, P. J. Schuck and D. J. Milliron, Concentrating and recycling energy in lanthanide codopants for efficient and spectrally pure emission: the case of NaYF4: Er3+/Tm3+ upconversion nanocrystals, J. Phys. Chem. B, 2012, 116, 10561–10570 CrossRef CAS PubMed.
- A. N. C. Neto, R. T. Moura and O. L. Malta, On the mechanisms of non-radiative energy transfer between lanthanide ions: centrosymmetric systems, J. Lumin., 2019, 210, 342–347 CrossRef.
|
This journal is © the Partner Organisations 2024 |
Click here to see how this site uses Cookies. View our privacy policy here.