DOI:
10.1039/D3QI02588H
(Review Article)
Inorg. Chem. Front., 2024,
11, 1668-1682
Recent progress on modulating luminescence thermal quenching properties of Bi3+-activated phosphors
Received
15th December 2023
, Accepted 16th January 2024
First published on 18th January 2024
Abstract
The luminescence thermal quenching properties of phosphor materials have been a huge challenge for their wide application. Therefore, how to modulate the thermal quenching property of phosphor materials has become a research hotspot nowadays. The luminescence thermal quenching behavior of rare earth and transition metal ions has been widely researched in the past decades. In recent years, bismuth as a novel, nontoxic, and inexpensive activator ion has attracted much attention from researchers. However, Bi3+-activated phosphors always suffer from horrible thermal quenching effects. In this contribution, many Bi3+-activated phosphors with excellent antithermal quenching are summarized in detail, and four design strategies for modulating the thermal quenching properties of Bi3+-activated phosphors are proposed: (1) defect engineering; (2) structural modulation; (3) lattice structure rigidity; and (4) energy transfer. In addition, the challenges and opportunities for the wide application of Bi3+-activated phosphors are presented. This review provides a reference for the design and development of novel antithermal quenching Bi3+-activated phosphors.
1. Introduction
Phosphor-converted light-emitting diodes (pc-LEDs) are extensively utilized in solid-state lighting, backlight displays, and biological imaging due to their low energy consumption, high brightness, and rapid response time.1–3 However, the luminescence thermal quenching properties of phosphors have been a significant challenge for the broad application of pc-LEDs.4,5 Bi3+-activated phosphors are widely used in pc-LEDs owing to the fact that they are non-toxic, inexpensive, and can be synthesized directly under air conditions without the need for a reducing environment.6 However, Bi3+-activated phosphors always suffer from horrible thermal quenching (TQ) effects. It is a great challenge to improve the antithermal quenching characteristics of Bi3+-activated phosphors for their applications in pc-LEDs and even in high power and laser LEDs.7,8
In the past few years, several reviews have briefly introduced several important design strategies to reduce the thermal quenching performance of inorganic luminescent materials, including component design such as defects, crystal structure, and crystallinity of materials, and composite structure design such as laminate structure, surface coating and glass process.9 A large number of studies have shown that the thermal quenching phenomenon of the Bi3+ 3P1 → 1S0 transition is closely related to the cross-relaxation between the excited state 3P1 and ground state 1S0 levels of Bi3+ ions and thermal ionization at high temperatures.10 In general, the greater the energy level difference between the 3P1 energy level of the Bi3+ ion and its intersection point with the 1S0 energy level (cross-relaxation point), the less likely it is to experience cross-relaxation, and the better the antithermal quenching.11 At the same time, the greater the energy difference between the 3P1 energy level of the Bi3+ ion and the conduction band of the matrix, the less likely it is that thermal ionization will occur, and it is easier to suppress the thermal quenching phenomenon and reduce the non-radiative transition process.12 Accordingly, four strategies, namely defect engineering, structural modulation, lattice structure rigidity, and energy transfer, to modulate the thermal quenching characteristics of Bi3+-activated phosphors are summarized and presented for developing more Bi3+-activated antithermal quenching pc-LEDs.
2. Luminescence thermal quenching properties of Bi3+-activated phosphors
The electron configuration of the Bi3+ ion is [(Xe)4f145d10]6s2, with a ground state of 1S0. The excited states originating from the 6s6p configuration are the 3P0, 3P1, 3P2 and 1P1 states, arranged in ascending order of energy.13 Transitions from 1S0 to 3P1, 3P2 and 1P1 are represented as A-, B- and C-bands in spectroscopy, as shown in Fig. 1a. The transitions from 1S0 to 3P0 and 3P2 remain entirely spin-forbidden, irrespective of the unique configuration. The energy levels of 3P1 and 1P1 are mixed by spin–orbit coupling. Therefore, only 1S0 → 3P1 and 1S0 → 1P1 transitions are expected to have reasonable absorption intensity.14 In most matrices, the A-band is located in the ultraviolet region, while the C-band is located in the vacuum ultraviolet (VUV) region, constituting the broad absorption band of the Bi3+ ion.
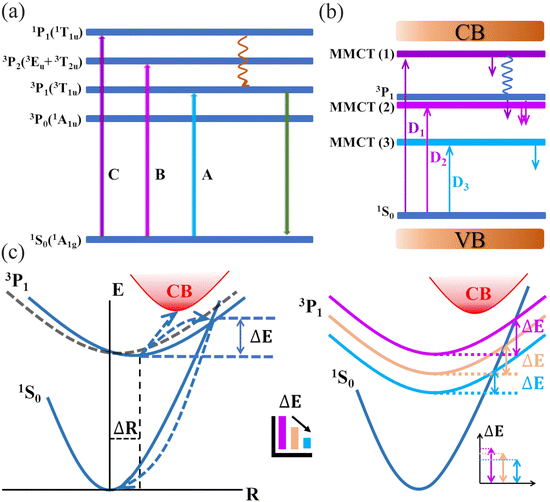 |
| Fig. 1 (a) Energy level scheme of Bi3+ ion. (b) Different configurations of energy levels leading to luminescence in Bi3+-activated phosphors. (c) Thermal quenching mechanisms of Bi3+ ion. | |
In addition, the metal-to-metal charge transfer (MMCT) process can occur between the Bi3+ ion and the transition metal ions with d0 or d10 configurations, such as Ti4+, V5+, Nb5+, Ta5+, Mo6+, and W6+ in oxide-type host materials, which involves transferring an electron from the Bi3+ ion to the host metal cations (Mn+) (M = Ti, V, Nb, Ta, Mo). According to the electronic configuration of Mn+, the transition is expressed as Bi3+(6s2)/Mn+(d0) → Bi4+(6s1)/M(n−1)+(d1) or Bi3+(6s2)/Mn+(d10) → Bi4+(6s1)/M(n−1)+(s1).13 Boutinaud developed a preliminary empirical model to predict the MMCT emission in some Bi3+-doped d0 transition metal oxides (titanates, vanadates, niobates, etc.), and proposed three types of MMCT emission,15 as shown in Fig. 1b. (1) The MMCT energy is less than the 3P1 energy level. In this case, the MMCT state is directly excited and emitted. The 3P1 → 1S0 transition is not excluded. (2) 3P1 → 1S0 and MMCT transition have roughly the same energy. In this case, both the MMCT state and 3P1 state should emit, and the relative intensity will be different according to different temperatures. (3) The MMCT energy is greater than the 3P1 → 1S0 transition. In this case, the 3P1 state is directly 1S0 → 3P1 excited and 3P1 → 1S0 emitted. Excitation of the MMCT state is still possible and after absorbed energy transfer the state can be MMCT emission or 3P1 → 1S0 emission.
In general, the Bi3+ thermal quenching phenomenon is closely related to the thermal ionization processes and cross-relaxation between 3P1 (excited state) and 1S0 (ground state) energy levels at high temperatures,10 as shown in Fig. 1c. The cross-relaxation process occurs when the temperature is high enough that an electron located at 3P1 can overcome the energy barrier (ΔE), reach the intersection of the 3P1 and 1S0 energy levels, and then return to 1S0. The larger the ΔR (the horizontal distance between the two parabolas 3P1 and 1S0) and ΔE values are, the more difficult it is for the electrons located in the 3P1 energy level to reach 1S0 by non-radiative transitions during the warming process, and the better the antithermal quenching performance of Bi3+ is.16 The process of thermal ionization is the transition of electrons in the excited state during warming to the conduction band of the host, which is lost by a non-radiative transition.12
3. Bi3+-activated phosphor thermal quenching modulation strategy
Most of the current pc-LED phosphor materials consist of rare earth or transition metal activator ions and inorganic hosts. The activator ions are principally Eu2+, Ce3+, and Yb2+ represented by 5d–4f electron transitions;17,18 Eu3+, Tb3+, and Pr3+ represented by 4f–4f electron transitions;19,20 and Mn2+, Mn4+, and Cr3+ represented by 3d–3d electron transitions.21 Except for the 4f–4f electron transition, the luminescence properties of the 5d–4f and 3d–3d electron transitions are extremely susceptible to the influence of the surrounding environment. The main group element Bi3+ with 6p–6s electron transition is very sensitive to changes in the local lattice environment, and its emission can be dramatically regulated from blue → green → yellow → red depending on the radius, electronegativity, and change in charge of the surrounding ions.13 These new environmentally friendly non-toxic, inexpensive, wide-emission Bi3+-activated phosphors with full visible spectrum modulation have great application advantages for solid-state lighting. However, Bi3+-activated phosphors always suffer from horrible thermal quenching effects. Accordingly, we propose four strategies to section the thermal quenching properties of Bi3+-activated phosphors, as shown in Fig. 2: (1) defect engineering; (2) structural modulation; (3) lattice structure rigidity; and (4) energy transfer.
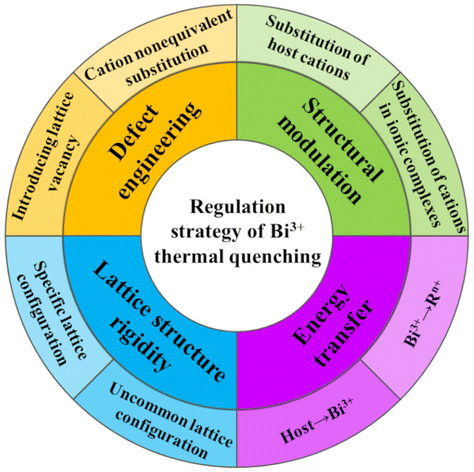 |
| Fig. 2 Bi3+-activated phosphor thermal quenching modulation strategy. | |
3.1. Defect engineering
As key parameters in modulating the thermal quenching properties of fluorescent materials, defects have two kinds of effects on phosphors. On the one hand, forming a suitable defect energy level, which can compensate the emission of luminescent ions in the process of heating and improve their antithermal quenching properties.22 On the other hand, due to the complexity of defects introduced in the synthesis process, some defects on the surface of phosphor particles will lead to the loss of activator energy. Removing Ca cation vacancy defects in Ca3(PO4)2:0.07Ce3+,0.07M (M = Li+, Na+, and K+) phosphors by using an anti-defect engineering strategy with the introduction of Li+, Na+, and K+ ions has been proposed by Pan et al.23 The photoluminescence emission (PL) intensity recorded at 150 °C in these Ca3(PO4)2:Ce3+,Li+, Ca3(PO4)2:Ce3+,Na+ and Ca3(PO4)2:Ce3+,K+ phosphors maintained 78.33%, 87.92% and 69.67% of the value at 25 °C, compared with 45.81% in the Ca3(PO4)2:Ce3+. At the same time, too high a concentration of defects will destroy the host lattice structure, which reduces the stability of the host structure and antithermal quenching properties of activator ions.6
Fig. 3a shows a schematic diagram of energy transfer from a typical defect energy level to the Bi3+ excitation energy level in a fluorescent material. In the low temperature range, some electrons are induced to be trapped and stored in the trap energy level by introducing defects in the fluorescent material as the trap energy level. After thermal stimulation, the trapped electrons transfer from the trap energy level to the excited state energy level of the luminescent ion through the host conduction band, thus realizing the luminescence process.24 Therefore, zero or even negative quenching occurs when the trap energy level captures and releases electrons in dynamic equilibrium.25 An effective energy transfer occurs from the trap to the luminescence center, formally providing additional excitation energy for the luminescent ions, resulting in stronger luminescence. Therefore, the amount, type and depth of defects become the key to the anomalous thermal quenching properties. In addition to selecting hosts with inherent defects,26 defects can be introduced by cation nonequivalent substitution and introducing lattice vacancies.
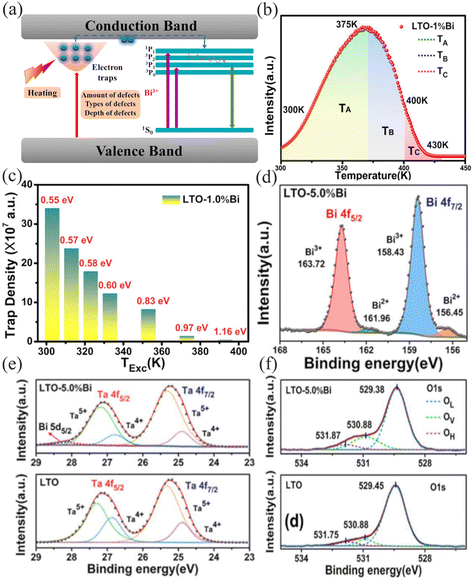 |
| Fig. 3 (a) Defect engineering modulation mechanism of the thermal quenching performance of Bi3+-activated phosphors. (b) The trap distribution in the LiTaO3:1.0%Bi3+ phosphor. (c) Trap depth distribution in the LiTaO3:1.0%Bi3+ phosphor. XPS spectra at the binding energy range for Bi 4f (d), Ta 4f (e), and O 1s (f). (b–f: Reproduced with permission.27 Copyright 2020 Elsevier B.V.). | |
3.1.1 Cation nonequivalent substitution.
Cation nonequivalent substitution is the most dominant way to introduce defects. Nonequivalent substitution is prone to occur when the radii of the ions inside the lattice are similar. On the one hand, higher valence ions replacing lower valence ions create positive defects, while the reverse process forms negative defects. On the other hand, their unequal substitution will cause the non-conservation of charge in the lattice, which as a result will induce the generation of defects with opposite charge. Tang et al. replaced the lower valence K+ ions with higher valence Eu2+ ions and the higher valence Hf4+ ions with lower valence Sc3+ ions in the K2HfSi3O9 host to form the positive defect
and negative defect Sc′Hf, respectively. Meanwhile positive defect
and negative defect Sc′Hf induced the formation of oppositely charged V′K and
, respectively, to maintain electrical neutrality.28 Wei et al. used higher valence Ce3+ ions to replace the lower valence Ca2+ in the Li2CaSi2N4 host to form positive defects
and further induced negative defects V′′Ca.29 LiTaO3:xBi3+ phosphors synthesized by Hu et al. produced BiLi2+ and TaLi4+ positive defects and induced
negative defects by the substitution of higher valence Bi3+ and Ta5+ for lower valence Li+.27 Recording a thermoluminescence (TL) spectrum is the most direct way to determine the number of electrons contained in the trap and the depth of the trap. As shown in Fig. 3b, the LiTaO3:0.01Bi3+ TL curve is mainly composed of three parts: TA, TB, and TC. The depth of the trap can be roughly estimated by using eqn (1):30where Tm represents the maximum value of the peak temperature, while E stands for the trap depth. The calculated trap depths from low to high are 0.55–0.60 eV (TA), 0.83–0.97 eV (TB) and 1.16 eV (TC), as shown in Fig. 3c. The combination of the pyroelectric spectra and the calculated trap densities allows us to determine that the shallower traps TA and TB store more electrons compared to the deep trap TC. Combined XPS analysis of the binding energies of the Ta 4f, Bi 4f, and O 1s orbitals in the matrix and LiTaO3:0.05Bi3+ identified three traps TA, TB, and TC as belonging to the
, BiLi2+ and TaLi4+ defects, respectively, and are shown in Fig. 3d–f.
3.1.2 Introducing lattice vacancies.
By directly reducing the ions in the lattice one can directly introduce lattice vacancies thus forming defects. However, the absence of ions in the lattice causes a decrease in the rigidity of the crystal structure. Therefore, the luminescence of activator ions is only favored by the introduction of a specific number of vacancies in a specific substrate. Wei et al.6 introduced Zn vacancy defects in Ba2ZnGe2O7:Bi3+ phosphors using an appropriate Zn deficiency strategy and determined that a 5% reduction in Zn is most favorable for thermoluminescence compensation (Fig. 4e). Accordingly, the thermally induced emission compensation mainly originates from the auto-oxidation behavior of Bi2+ ions in Zn vacancies and the presence of oxygen vacancy defects. Oxygen vacancies are induced by the charge imbalance caused by Zn vacancies and Bi3+ ions replacing Ba2+ ions, as shown in Fig. 4a. The presence of oxygen vacancies, Zn vacancies and Bi2+ ions can be confirmed by X-ray photoelectron spectroscopy (XPS) and the position of characteristic peaks of electron paramagnetic resonance (EPR) spectra as shown in Fig. 4b and c. The use of thermoluminescence (TL) spectroscopy confirms the maximum thermoluminescence emission of the 95% Zn sample (Fig. 4d). The emission integral intensities of Ba2Zn0.95Ge2O7:Bi3+ phosphors at 150, 200 and 250 °C reached 138%, 148% and 134% of those at room temperature, respectively (Fig. 4e).
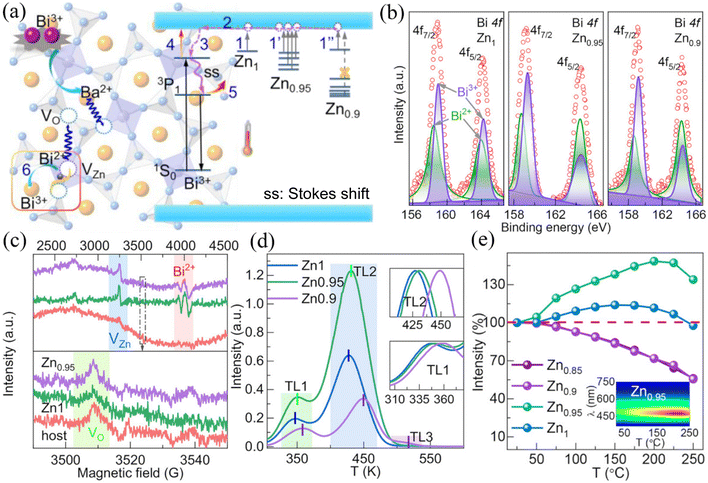 |
| Fig. 4 (a) Schematic mechanism for anti-TQ property in Ba2ZnGe2O7:Bi3+ and Ba2Zn0.95Ge2O7:Bi3+. (b) XPS spectra of Bi 4f for Ba2ZnGe2O7:Bi3+ (marked as Zn1), Ba2Zn0.95Ge2O7:Bi3+ (marked as Zn0.95), and Ba2Zn0.9Ge2O7:Bi3+ (marked as Zn0.9) phosphors. (c) EPR spectra for the Ba2ZnGe2O7 host, and Ba2ZnGe2O7:Bi3+ and Ba2Zn0.95Ge2O7:Bi3+ phosphors. (d) TL curves of Ba2ZnGe2O7:Bi3+, Ba2Zn0.95Ge2O7:Bi3+, and Ba2Zn0.9Ge2O7:Bi3+ phosphors; the insets are the magnified curves of peaks TL1 and TL2 (TL1, TL2, and TL3 represent the traps, respectively). (e) The integrated intensity of Ba2Zn1−xGe2O7:Bi3+ (0 ≤x ≤ 0.15); the inset plots the temperature-dependent PL spectrum of the Ba2Zn0.95Ge2O7:Bi3+ phosphors (a–e: Reproduced with permission.6 Copyright 2020 Wiley-VCH GmbH.). | |
3.2. Structural modulation
Variations in lattice structure are primarily due to variations in the atoms that compose the interior of the lattice. Since the anions that constitute the host directly affect the properties of the material (such as oxides, nitrides, halides), the formation of solid solutions by anionic substitution is not possible due to the vast differences in synthesis and preparation methods. Therefore, crystal structure modulation is generally performed by cation substitution in specific types of hosts.31 According to the differences in the constituent lattice cations, substitution can be mainly categorized into that of host cations and cations in ionic complexes. Once the crystal structure is determined, the luminescence thermal quenching properties of the phosphor can be strongly influenced by the chemical substitution of cations in the host cations and cations in ionic complexes.32 As shown in Fig. 5, the environment around Bi3+ is affected by the chemical substitution of the host cations and/or cations in ionic complexes, which affects the nephelauxetic effect and crystal-field splitting of Bi3+.13,33 The energy gap between the ground state 1S0 and the excited state 3P1 is altered, which affects the luminescence thermal quenching properties of Bi3+. In particular, due to the alteration of the ions constituting the host conduction bands, not only the thermal ionization process is affected, but also the MMCT process is generated in a specific substrate.
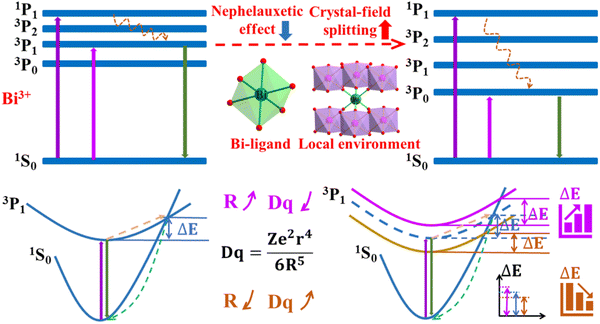 |
| Fig. 5 Structural modulation mechanism of the thermal quenching performance of Bi3+-activated phosphors. | |
3.2.1 Substitution of host cations.
The host cation is the Bi3+ substitution of cations in the matrix after doping with Bi3+. In general, the crystal-field splitting strength (Dq) can be evaluated by using eqn (2):13 |  | (2) |
where Z represents the charge and valence of the anion, e denotes the electron charge, r stands for the wave-function radius, and R signifies the bond length between the central cation and its ligands. Accordingly, the degree of Bi3+ crystal-field splitting is mainly related to the bond length R between Bi3+ and its ligand. If the two different host cations substituted in the lattice have the same coordination number and can form the same space group, the bond length R between Bi3+ and its ligand is proportional to the difference in radius between the two substituted ions. Generally, the larger the radius of the host cation, the longer the bond length between it and the ligand, and the longer the average bond length between Bi3+ and its ligand. As the bond length R between Bi3+ and its ligand increases, the crystal field splitting of Bi3+ decreases, and when the energy level difference between the ground and excited states increases, the activation energy of thermal quenching increases and the antithermal quenching property of Bi3+ increases. In the YVO4:Bi3+ phosphors prepared by Kang et al.,34 the small radius of Sc3+ was substituted by the large radius of Y3+ in an octa-coordinated environment. The bond length between Bi3+ and the ligand O increased, the crystal field splitting of Bi3+ decreased, the energy gap between the 1S0 and 3P1 energy levels increased, the Bi3+ emission was significantly blue-shifted as shown in Fig. 6a, and the Bi3+ emission showed a significantly linear dependence on the increase of Y3+ substitution, as shown in Fig. 6b. The energy gap between the 1S0 and 3P1 energy levels increased, the activation energy of thermal quenching increased, and the antithermal quenching property of Bi3+ increased.
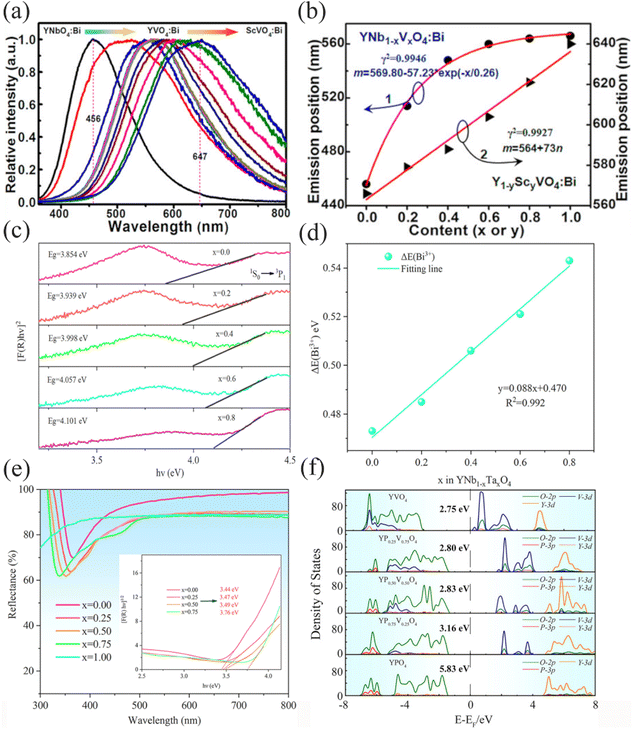 |
| Fig. 6 (a) Normalized emission spectra of (Y1−y,Scy) (Nb1−x,Vx)O4:Bi compounds. The Bi-doping content is 0.01 mol%, and the sequence for x and y values is 0.0, 0.2, 0.4, 0.6, 0.8, and 1.0. (b) Dependence of the emission band position on the V/Nb and Sc/Y ratios. The fitted curves (red) are based on the equation m = a + A exp(−n/k) for Y(Nb1−x,Vx)O4:Bi compounds (curve 1) and m = a + kn for (Y1−y,Scy)VO4:Bi compounds (curve 2), respectively. (c) The energy-gap value of Ynb1−xTaxO4:0.005Bi3+ calculated according to the Kubelka–Munk absorption function (x = 0.0–0.8). (d) Dependence of the thermal quenching activation energy (ΔE) on the Nb/Ta ratios. The fit line is shown in green. (e) Diffuse reflectance (DR) spectra of YV1−xPxO4:Bi3+ (x = 0.00–1.00). The inset is the band-gap value of YV1−xPxO4:Bi3+ calculated according to the Kubelka–Munk absorption function. (f) Density of states in bulk YV1−xPxO4 (x = 0.00–1.00) derived from DFT calculations. (a and b: Reproduced with permission.34 Copyright 2016, American Chemical Society. c and d: Reproduced with permission.11 Copyright 2021, American Chemical Society. e and f: Reproduced with permission.35 Copyright 2022, American Chemical Society.) | |
3.2.2 Substitution of cations in ionic complexes.
Substitution of cations in ionic complexes occurs at metal cation sites in the matrix other than the host cation. The crystal environment around Bi3+ can be indirectly affected by changing the cations in ionic complexes. The changes in ionic radius, electronegativity, and charge of cations in ionic complexes affect the ligand of Bi3+, which in turn affects the bond length between Bi3+ and the ligand. As shown in eqn (2), the larger the bond length R between Bi3+ and its ligand, the smaller the crystal field splitting of Bi3+, the larger the energy level difference between the ground state 1S0 and the excited state 3P1, the larger the activation energy of thermal quenching, and the better the antithermal quenching property of Bi3+. Because of the uncertainty of the effect of cation substitution on the surroundings of Bi3+ ions in ionic complexes, this structural change cannot be determined simply by the number of substituted ionic ligands and ionic radii, but rather by the direct determination of the energy gap between the altered Bi3+ excited state and the ground state. The relative positions of the excited and ground state energy levels of Bi3+ can be obtained not only by processing the diffuse reflectance spectra with the Kubelka–Munk formula (as shown in eqn (3)
36 and (4)
37), but it is also proportional to the activation energy of the thermal quenching ΔE (as shown in the Arrhenius formula of eqn (5)
38): | [F(R∞)hν]n = A(hν − Eg) | (3) |
where F(R∞) represents the Kubelka–Munk function, and n is determined by whether the host absorption signifies an indirectly (n = 1/2) or directly (n = 2) allowed transition. A represents the proportionality constant, and hν stands for the energy per photon. The Kubelka–Munk function F(R∞) can be expressed as eqn (4): | 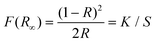 | (4) |
where R denotes the reflection coefficient, K represents the absorption coefficient, and S stands for the scattering parameter. The Arrhenius formula is: | 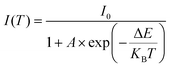 | (5) |
where ΔE signifies the activation energy intensity, I0 is the initial emission intensity measured at a specific temperature, I(T) represents the emission intensity at any temperature, T is the temperature corresponding to I(T), and A and KB are constants. KB is referred to as the Boltzmann constant, with a known value of 8.629 × 10−5 eV K−1. Guo's group substituted Ta for Nb in YNbO4:Bi3+ phosphors.11 When the ratio of Nb5+/Ta5+ changes from 1/0 to 0.2/0.8, the energy gap of Bi3+ increases from 3.854 eV to 4.101 eV (Fig. 6c). Simultaneously, the thermal quenching activation energy of Bi3+ increases from 0.473 eV to 0.543 eV (Fig. 6d). Consequently, the emission integral intensity at 150 °C relative to room temperature increases from 45% to 90%. Since some cations in ionic complexes with d0 electronic configurations are an important part of the composition of the matrix conduction bands and the MMCT transitions. The substitution of cations in ionic complexes severely affects the size of the matrix energy gap and the position of the MMCT band, which in turn affects the thermal quenching property of Bi3+ by influencing the type of emission and the thermal ionization process. When Guo's group used Y0.99V1−xPxO4:0.01Bi3+ as a model phosphor,35 the Bi3+ band gap increased from 3.44 to 3.76 eV (Fig. 6e), and the host YV1−xPxO4 widened its band gap from 2.75 to 3.16 eV (Fig. 6f), and this led to a decrease in electron-transfer potential energy (ΔET) and an increase in thermal quenching activation energy (ΔE). Consequently, the relative emission intensity increased from 0.06 to 0.64 at 303–523 K.
3.3. Lattice structure rigidity
The lattice structure rigidity of the host is closely related to the thermal quenching property of the activator ions. A frame with good structural rigidity can significantly reduce the vibration of the lattice during warming and thus reduce the loss of emission. The atoms in the lattice with excellent rigid structure are not easily displaced during the temperature rise, and the lattice is still able to maintain a relatively good structural rigidity, as shown in Fig. 7a. In contrast, owing to the enhancement of thermal vibration due to warming, the atoms of the lattice with poor structural rigidity are significantly shifted and the rigidity of the lattice is significantly reduced, as shown in Fig. 7b. The Debye temperature (θD) calculated by experiment and density functional theory (DFT) can be used as a key parameter to measure the rigidity of the crystal structure.39,40 The high Debye temperature of fluorescent material corresponds to low lattice vibration frequency and small Stokes shift, which often reduce the possibility of non-radiative transition. Therefore, the Debye temperature can help in finding and screening host materials with relatively good antithermal quenching performance. The Debye temperature (θD) can be obtained from the quasi-harmonic Debye model and can be calculated from eqn (6) and (7):41 | 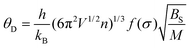 | (6) |
| 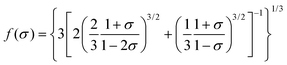 | (7) |
where kB and h represent the simplified Boltzmann constant and Planck constant, respectively; M represents the relative molecular mass of the primitive cell; Bs is the thermal insulator elastic modulus of the crystal; n is the number of atoms contained in each primitive cell; V represents the volume of the primitive cell; and σ is the Poisson's ratio.
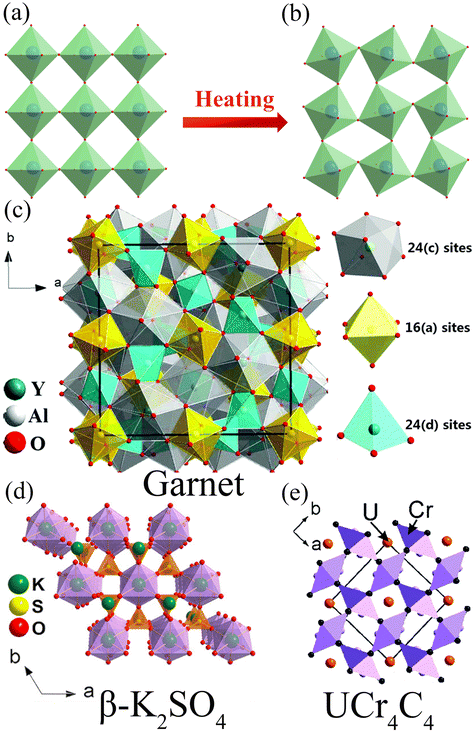 |
| Fig. 7 Crystal structure diagrams of structures with good (a) and poor (b) structure rigidity relative to the original crystal structure during the heating process. (c) Garnet structure model. (d) β-K2SO4 structure mode. (e) UCr4C4 structure model. (c: Reproduced with permission.43 Copyright 2017 Royal Society of Chemistry. d: Reproduced with permission.44 Copyright 2018, American Chemical Society. e: Reproduced with permission.45 Copyright 2016 Royal Society of Chemistry.) | |
3.3.1 Specific lattice configuration.
Materials with high structural rigidity and excellent lattice symmetry can reduce the lattice vibration frequency, inhibit the non-radiative transition process of ions, and reduce the phonon loss. Brgoch et al. pointed out that lattices with high polyhedral connectivity in fluorescent materials can effectively limit the freedom of vibration and reduce the nonradiative relaxation process involving phonons, which ensures that such fluorescent materials usually have good thermal stability.42 Therefore, compared with some common fluorescent materials, garnet-type, β-K2SO4-type and UCr4C4-type phosphors usually have better structural rigidity, which is more conducive to the thermal stability of phosphors.
As shown in Fig. 7c, the garnet structure belongs to the cubic system, and the space group is Ia3d.43 The general formula is A3B2C3O12, where A, B and C are cations located in different symmetrical positions. The atom A occupies the 24(c) lattice of an 8-coordinated dodecahedron, the atom B occupies the 16(a) lattice of a 6-coordinated octahedron, and the atom C occupies the 24(d) lattice of a 4-coordinated tetrahedron. Each octahedron is connected with six tetrahedra, and each tetrahedron is connected with four octahedra through a common angle. The aluminate phosphor Y3Al2Ga3O12:Bi3+ with garnet configuration synthesized by Zheng et al. still maintains 90% room temperature emission intensity at 420 K.46 Ye et al. synthesized the germanate Ca3Lu2Ge3O12:Bi3+ phosphor with garnet configuration, which still maintains 75% room temperature emission intensity at 423 K.47 The germanate Ca4ZrGe3O12:Bi3+ phosphor with garnet configuration synthesized by Jiang et al. still maintains 80% room temperature emission intensity at 423 K.48
As shown in Fig. 7d, the prototype of the β-K2SO4 mineral structure belongs to an orthorhombic system, and the space group is Pnam.44 Two typical types include an orthosilicate A2SiO4 type (A = Sr, Ba) and phosphate ABPO4 type (A is a monovalent cation such as Na+, K+ while B is a divalent cation such as Ca2+, Sr2+, Ba2+). The K2BaCa(PO4)2:Bi3+ phosphor with β-K2SO4 configuration synthesized by Yang et al. still maintains 90% room temperature emission intensity at 423 K.49
The structural prototype of a UCr4C4 mineral belongs to the tetragonal system, with the space group of I4/m, where Cr and C are connected to form CrC4 tetrahedra, which are connected to form the skeleton, with U ions filling the space between the tetrahedra,50 as shown in Fig. 7e. The general formula of the compound can be written as Me(A,B)4X4, where Me is an alkali metal or alkaline earth metal ion, and A and B are coordination ions. [AX4] and [BX4] tetrahedra form the vierer ring in the direction of [001] through the common edge or common vertex connection. Me ions are in the formed ring structure, and their lattice sites are highly symmetrical and the density k = (AB/X) = 1, so they have strong structural rigidity. Wu et al. synthesized the Ba2ZnGe2O7:Bi3+ phosphor with UCr4C4 type structure, which still maintains 98% room temperature emission intensity at 473 K.51
3.3.2 Uncommon lattice configuration.
In addition, there are some special structural matrices with high polyhedral connectivity and lattice symmetry, such as the Sr3Y(BO3)3:Bi3+ phosphor synthesized by Wu et al., which has high polyhedral connectivity and high rigidity and still maintains 80% room temperature emission intensity at 423 K.52 The Ca6BaP4O17:0.5%Bi3+ phosphor synthesized by Liu et al. has high structural rigidity (θD = 632 K) and maintains 99% room temperature emission integrated intensity at 423 K.53
3.4. Energy transfer
In addition to energy transfer from defect energy levels to Bi3+, energy transfer from the host and other sensitizer ions to Bi3+ is possible. Nevertheless, owing to the higher energy levels associated with Bi3+, the 1S0 → 1P1 and 1S0 → 3P1 absorptions are located in the near ultraviolet (NUV) region. Constructing energy transfers from other sensitizer ions to Bi3+ is challenging work. Up-conversion is one possible approach, such as the energy transfer of Pr3+ → Bi3+ through the special 4f5d energy level of Pr3+ in YBO3:Pr3+,Bi3+ phosphors observed by Zhao et al.54 Unfortunately, few investigations have focused on the thermal quenching properties of Bi3+. Accordingly, we focus on the energy transfer from the host → Bi3+ and Bi3+ → Rn+ to modulate the thermal quenching properties of Bi3+, as shown in Fig. 8a.
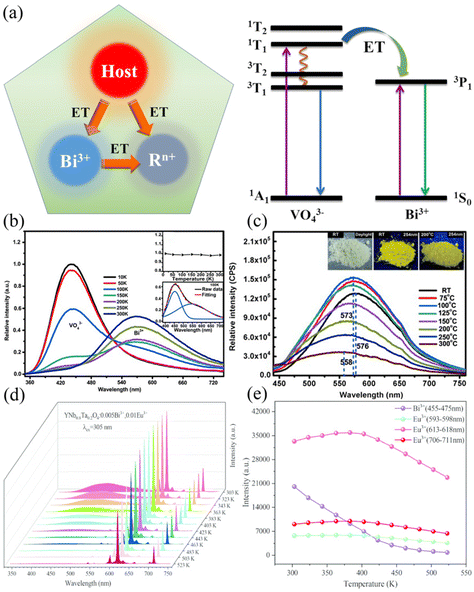 |
| Fig. 8 (a) Energy transfer modulation mechanism of the thermal quenching performance of Bi3+-activated phosphors. (b) Emission spectra of Lu0.98VO4:2.0%Bi3+ under 265 nm excitation at 10–300 K. Inset above: the dependence of the relative emission intensity of Lu0.98VO4:2.0%Bi3+ on temperature upon excitation at 380 nm; inset below: the Gaussian peak deconvolution of the emission spectrum at 100 K. (c) Emission spectra (λex = 385 nm) of Lu0.995VO4:0.5%Bi3+ measured at 298–573 K. Insets show photographs of Lu0.98VO4:2.0%Bi3+ exposed to daylight at 298 K (left), 254 nm light at 298 K (middle), and 254 nm light at 473 K (right). (d) PL spectra of YNb0.8Ta0.2O4:0.005Bi3+,0.01Eu3+ at various temperatures. (e) The integrated emission intensity of Bi3+ and Eu3+ at various temperatures. (b and c: Reproduced with permission.56 Copyright 2014 Wiley-VCH Verlag GmbH & Co. KGaA, Weinheim. d and e: Reproduced with permission.59 Copyright 2021 Royal Society of Chemistry.). | |
3.4.1 Host → Bi3+.
The host → Bi3+ energy transfer is the most dominant way to enhance the antithermal quenching property of Bi3+. Anionic groups in the host tend to have more pronounced absorption in the near-UV area (such as VO43−, NbO43−, TaO43−, MoO66−, WO66−, etc.). In specific hosts, the emission of anionic groups can be directly observed at room temperature and these hosts are referred to as self-activated hosts, such as LuVO4,55,56 ScVO4,55 and CaWO4.57 In most cases, the host emission is already quenched at room temperature and can only be observed at ultracold temperatures, such as in LiTaO3.58 As shown in Fig. 8b, Kang et al.56 investigated thermal quenching of the Lu0.98VO4:2.0%Bi3+ phosphor during the low temperature process from 10 to 300 K. With an increase of temperature under 265 nm excitation, the emission of VO43− is weakened, the emission of activator Bi3+ is enhanced, the energy transfer of VO43− → Bi3+ is significantly strengthened, and the antithermal quenching property of Bi3+ is greatly improved. As shown in Fig. 8c, Kang et al.56 also tested the thermal quenching of the Lu0.98VO4:0.5%Bi3+ phosphor during the process from room temperature to 573 K. Under the 385 nm excitation of VO43−, Bi3+ showed good antithermal quenching properties, and maintained more than 80% of the initial intensity at 423 K. Subsequent studies by Peng's group also confirmed that the efficiency of energy transfer from host → Bi3+ is mainly related to the concentration of the activator Bi3+ and the energy gap between the two energy levels.56,57
3.4.2 Bi3+ → Rn+.
Considering that Bi3+ 1S0 → 1P1 and 1S0 → 3P1 absorptions are located in the NUV region, Bi3+ can be used as a good sensitizer ion to transfer energy to other rare earth or transition metal ions Rn+ (Rn+ = Eu3+, Sm3+, Tb3+, Dy3+, Mn2+, Mn4+, etc.) in most cases.13 Accordingly, this strategy of compensating for other activator ions by sacrificing the specific emission of Bi3+ provides ideas for the design and development of highly sensitive dual luminescence fluorescence temperature probes based on fluorescence intensity ratio (FIR).60,61 The efficiency of energy transfer is an essential factor in limiting the sensitivity of temperature measurement by this type of fluorescent temperature probe. As shown in Fig. 8d and e, Guo's group realized the energy transfer of Bi3+ → Eu3+ by double doping Bi3+ and Eu3+ in the YNb0.8Ta0.2O4 host.59 Since the energy transfer efficiencies are different, the emission of Bi3+ and Eu3+ (613–618 nm) was selected for FIR temperature measurement to have the maximum relative sensitivity.
4. Summary and outlook
In this review, four strategies to modulate the luminescence thermal quenching properties of Bi3+-activated phosphors are presented: (1) defect engineering; (2) structural modulation; (3) lattice structure rigidity; and (4) energy transfer. Moreover, the specific measurements and theoretical basis for modulating the luminescence thermal quenching properties of phosphors are detailed with Bi3+ as an example, which can be widely used for other ion-activated fluorescent materials. The four strategies for modulating the luminescence thermal quenching properties of Bi3+-activated phosphors are not completely independent of each other. We can either integrate these four strategies to design and develop phosphors with good antithermal quenching properties, or investigate the primary factors influencing the luminescence thermal quenching of a specific fluorescent material. In addition, examples of antithermal quenching and the corresponding mechanism for representative Bi3+-activated phosphors are summarized as shown in Table 1, which provides a reference for finding and developing high-quality antithermal quenching phosphors for applications in lighting and displays.
Table 1 Comparison of antithermal quenching and the corresponding mechanism for representative Bi3+-activated phosphor materials
Composition |
λ
ex/nm |
λ
em/nm |
I@T (K) |
Mechanism |
Ref. |
Ca3Sc2Si3O12:0.06Bi3+ |
365 |
447 |
103.6%@423 |
ET between the traps and Bi3+ |
62
|
BaSc2Ge3O10:0.005Bi3+ |
305 |
370 |
107%/93%@353/473 |
ET between the traps and Bi3+ |
63
|
LaGd2SbO7:0.03Bi3+ |
350 |
520 |
135.2%@423 |
ET between the traps and Bi3+ |
64
|
Ba2Zn0.95Ge2O7:0.005Bi3+ |
346 |
500 |
138%/134%@423/523 |
ET between the traps and Bi3+ |
6
|
KGaGeO4:0.01Bi3+ |
365 |
497 |
207%/351%@393/513 |
ET between the traps and Bi3+ |
65
|
Ba2Y0.94AlO5:0.06Bi3+ |
324 |
574 |
100%@560 |
ET between the traps and Bi3+ |
66
|
La0.5ScO3:0.5Bi3+ |
350 |
420 |
128.3%@573 |
ET between the traps and Bi3+ |
67
|
BaGa2O4:0.02Bi3+ |
310 |
638 |
93.6%@473 |
ET between the traps and Bi3+ |
68
|
|
325 |
638 |
88.1%@473 |
ET between the traps and Bi3+ |
68
|
|
365 |
638 |
174.0%@473 |
ET between the traps and Bi3+ |
68
|
Cs2.98Zn6B9O21:0.02Bi3+ |
332 |
436 |
100%/77%@423/523 |
ET between the traps and Bi3+ |
69
|
SrSc2O4:0.05Bi3+ |
295 |
650 |
110%@423 |
ET between the traps and Bi3+ |
70
|
Ba1.5Sr0.5Ga4O8:0.01Bi3+ |
325 |
627 |
97%@423 |
Structural modulation |
71
|
YNb0.2Ta0.8O4:0.005Bi3+ |
297 |
470 |
90%@423 |
Structural modulation |
11
|
CaBaGa4O8:0.007Bi3+ |
340 |
530 |
73%@423 |
Highly rigid structure |
72
|
Ba2ZnGe2O7:0.03Bi3+ |
362 |
520 |
98%@473 |
Highly rigid structure |
51
|
Ba3Sc4O9:0.016Bi3+ |
330 |
508 |
62.2%@423 |
Highly rigid structure |
73
|
|
377 |
538 |
68.1%@423 |
Highly rigid structure |
73
|
K2BaCa(PO4)2:0.01Bi3+ |
340 |
460 |
95%/68%@373/473 |
Highly rigid structure |
49
|
Na2Y2B2O7:0.005Bi3+ |
380 |
415 |
76.47%@420 |
Highly rigid structure |
74
|
Ca3.92ZrGe3O12:0.08Bi3+ |
370 |
435 |
64%@483 |
Highly rigid structure |
48
|
Sr2.55Y(BO3)3:0.45Bi3+ |
370 |
420 |
79.99%@420 |
Highly rigid structure |
52
|
Ba2Lu5B5O17:0.01Bi3+ |
370 |
408 |
70%@423 |
Highly rigid structure |
75
|
K2MgGeO4:0.01Bi3+ |
335 |
614 |
85%@393 |
Highly rigid structure |
76
|
Ca5.995BaP4O17:0.005Bi3+ |
355 |
398 |
99%@423 |
Highly rigid structure |
53
|
Lu0.99VO4:0.01Bi3+ |
385 |
573 |
187.8%/50%@373/573 |
Host → Bi3+ |
56
|
Although there have been some approaches to address the strong thermal quenching behavior of Bi3+-activated phosphors, there is a lack of systematic research on high-quality Bi3+-activated phosphors with anti-thermal quenching. The challenges and perspectives for the wide application of Bi3+-activated phosphors are summarized as follows:
(1) At present, although the changes in the microstructures of the crystals have been confirmed by some characterization methods, which explained the improvement of the thermal quenching properties of the activator ion Bi3+, it is still a challenge to improve and adjust the crystal structure regularly to regulate the phosphor thermal quenching properties.
(2) There is a lack of evaluation and application systems for the thermal quenching properties of phosphors. How to regularly adjust the thermal quenching characteristics of phosphors to satisfy the needs of practical applications is an urgent problem to be solved.
(3) With the development of theoretical calculations, there is some significance in the validation of calculating the parameters related to the thermal quenching characteristics of fluorescent materials, such as the Debye temperature and the energy gap of fluorescent materials, although, through the density functional theory (DFT), we are often faced with the problems of insufficient accuracy of theoretical calculations and inconsistency between experimental phenomena and theoretical calculation data.
(4) Many Bi3+-activated phosphors are still in the research stage and need to be further optimized and improved to be suitable for practical applications.
Author contributions
Xiang Lv: methodology, validation, formal analysis, investigation, data curation, writing – original draft, and writing – review & editing. Ran Xiao: methodology and investigation. Jianxia Liu: methodology and investigation. Chunwei Yang: methodology and investigation. Yanmei Xin: methodology and investigation. Ning Guo: conceptualization, methodology, resources, supervision, and funding acquisition.
Conflicts of interest
There are no conflicts of interest to declare.
Acknowledgements
This work is financially supported by the National Natural Science Foundation of China (grant no. 21401130), the Opening Research Fund of the State Key Laboratory of Rare Earth Resource Utilization, the Changchun Institute of Applied Chemistry, and the Chinese Academy of Sciences (RERU2014005).
References
- S. Piao, Y. Wang and G. Zhu,
et al., Structural design and evolution of a novel Bi3+-doped narrow-band emission blue phosphor with excellent photoluminescence performance for wide color gamut wLED, J. Mater. Chem. C, 2021, 9, 14777–14787 RSC.
- G. Wei, Z. Wang and R. Li,
et al., Enhancement of Near-Infrared Phosphor Luminescence Properties via Construction of Stable and Compact Energy Transfer Paths, Adv. Opt. Mater., 2022, 10, 202201076 Search PubMed.
- J. Qiao, J. Zhao and Q. Liu,
et al., Recent advances in solid-state LED phosphors with thermally stable luminescence, J. Rare Earths, 2019, 37, 565–572 CrossRef CAS.
- G. Liu, M. S. Molokeev and Z. Xia, Structural Rigidity Control toward Cr3+-Based Broadband Near-Infrared Luminescence with Enhanced Thermal Stability, Chem. Mater., 2022, 34, 1376–1384 CrossRef CAS.
- X. Zhang, L. Zhang and B. Sun,
et al., Abnormal Thermal Quenching Effect of High Power Density Excited Fluorescent Materials, Chin. J. Lumin., 2021, 42, 1458–1481 CAS.
- Y. Wei, H. Yang and Z. Gao,
et al., Anti–Thermal–Quenching Bi3+ Luminescence in a Cyan–Emitting Ba2ZnGe2O7:Bi Phosphor Based on Zinc Vacancy, Laser Photonics Rev., 2020, 15, 202000048 Search PubMed.
- J. Zhou, Y. Wang and Y. Chen,
et al., Single-Crystal Red Phosphors and Their Core-Shell Structure for Improved Water-Resistance for Laser Diodes Applications, Angew. Chem., Int. Ed., 2021, 60, 3940–3945 CrossRef CAS PubMed.
- Z. Yang, T. de Boer and P. M. Braun,
et al., Thermally stable red-emitting oxide ceramics for laser lighting, Adv. Mater., 2023, 35, 2301837 CrossRef CAS PubMed.
- P. Dang, W. Wang and H. Lian,
et al., How to Obtain Anti–Thermal–Quenching Inorganic Luminescent Materials for Light–Emitting Diode Applications, Adv. Opt. Mater., 2022, 10, 202102287 Search PubMed.
- M. Amachraa, Z. Wang and C. Chen,
et al., Predicting Thermal Quenching in Inorganic Phosphors, Chem. Mater., 2020, 32, 6256–6265 CrossRef CAS.
- Q. Ma, N. Guo and J. Wang,
et al., Constructing a Model for Tuning the Thermal Quenching Properties of Bismuth-Doped Phosphors by Energy-Gap Modulation, J. Phys. Chem. C, 2021, 125, 20717–20726 CrossRef CAS.
- C. Wang, S. Zhang and Q. Bai,
et al., Luminescent thermal behavior of Eu3+ in K5Bi(MoxW1−xO4)4 phosphors, Opt. Mater., 2022, 134, 113211 CrossRef CAS.
- P. Dang, D. Liu and G. Li,
et al., Recent Advances in Bismuth Ion–Doped Phosphor Materials: Structure Design, Tunable Photoluminescence Properties, and Application in White LEDs, Adv. Opt. Mater., 2020, 8, 201901993 Search PubMed.
- Y. Wang, N. Guo and B. Shao,
et al., Adjustable photoluminescence of Bi3+ and Eu3+ in solid solution constructed by isostructural end components through composition and excitation-driven strategy, Chem. Eng. J., 2021, 421, 127735 CrossRef CAS.
- P. Boutinaud, Revisiting the spectroscopy of the Bi3+ ion in oxide compounds, Inorg. Chem., 2013, 52, 6028–6038 CrossRef CAS PubMed.
- G. Liu and Z. Xia, Modulation of Thermally Stable Photoluminescence in Cr3+-Based Near-Infrared Phosphors, J. Phys. Chem. Lett., 2022, 13, 5001–5008 CrossRef CAS PubMed.
- S. Xia and C. Duan, The Simple Model and Its Application to Interpretation and Assignment of 4f-5d Transition Spectra of Rare Earth Ions in Solids, Chin. J. Lumin., 2006, 27, 154–158 CAS.
- J. Qiao and Z. Xia, Progress in Eu2+ Doped Inorganic near Infrared Phosphors, J. Chin. Soc. Rare Earths, 2023, 41, 25–38 Search PubMed.
- S. S. Zhou, G. C. Jiang and X. T. Wei,
et al., Pr3+-Doped β-NaYF4 for Temperature Sensing with Fluorescence Intensity Ratio Technique, J. Nanosci. Nanotechnol., 2014, 14, 3739–3742 CrossRef CAS PubMed.
- X. Zhou, S. Jiang and G. Xiang,
et al., Tunable emission color of Li2SrSiO4:Tb3+ due to cross–relaxation process and optical thermometry investigation, J. Am. Ceram. Soc., 2018, 101, 3076–3085 CrossRef CAS.
- L. Li, H. Li and Z. Wu,
et al.,
Novel double-perovskite SrLaLiTeO6:Mn4+ far-red phosphor with superior thermal stability for indoor plant growth LED, J. Lumin., 2021, 238, 118286 CrossRef CAS.
- Z. Qiao, X. Wang and C. Heng,
et al., Exploring Intrinsic Electron-Trapping Centers for Persistent Luminescence in Bi3+-Doped LiREGeO4 (RE = Y, Sc, Lu): Mechanistic Origin from First-Principles Calculations, Inorg. Chem., 2021, 60, 16604–16613 CrossRef CAS PubMed.
- X. Pan, L. Mei and Y. Zhuang,
et al., Anti-Defect engineering toward high luminescent efficiency in whitlockite phosphors, Chem. Eng. J., 2022, 434, 134652 CrossRef CAS.
- X. Lv, N. Guo and R. Xiao,
et al., Tuning the thermal quenching properties of Ga3+-modified LiTaO3:Bi3+ phosphor through defect engineering strategy, J. Lumin., 2023, 255, 119609 CrossRef CAS.
- X. Ji, J. Zhang and Y. Li,
et al., Improving Quantum Efficiency and Thermal Stability in Blue-Emitting Ba2−xSrxSiO4:Ce3+ Phosphor via Solid Solution, Chem. Mater., 2018, 30, 5137–5147 CrossRef CAS.
- Q. Ran, Y. Zhang and J. Yang,
et al., White-light defect emission and enhanced photoluminescence efficiency in a 0D indium-based metal halide, J. Mater. Chem. C, 2022, 10, 1999–2007 RSC.
- R. Hu, Y. Zhang and Y. Zhao,
et al., UV–Vis-NIR broadband-photostimulated luminescence of LiTaO3:Bi3+ long-persistent phosphor and the optical storage properties, Chem. Eng. J., 2020, 392, 124807 CrossRef CAS.
- Z. Tang, G. Zhang and Y. Wang, Design and Development of a Bluish-Green Luminescent Material (K2HfSi3O9:Eu2+) with Robust Thermal Stability for White Light-Emitting Diodes, ACS Photonics, 2018, 5, 3801–3813 CrossRef CAS.
- Q. Wei, J. Ding and Y. Wang, A novel tunable extra-broad yellow-emitting nitride phosphor with zero-thermal-quenching property, Chem. Eng. J., 2020, 386, 124004 CrossRef CAS.
- Y. H. Kim, P. Arunkumar and B. Y. Kim,
et al., A zero-thermal-quenching phosphor, Nat. Mater., 2017, 16, 543–550 CrossRef CAS PubMed.
- J. Chan, L. Cao and Z. Xu,
et al., Cation substitution induced highly symmetric crystal structure in cyan-green-emitting Ca2La1−xLuxHf2Al3O12:Ce3+ solid-solution phosphors with enhanced photoluminescence emission and thermal stability: Toward full-visible-spectrum white LEDs, Mater. Today Phys., 2023, 35, 101130 CrossRef CAS.
- X. Lv, N. Guo and W. Lv,
et al., Regulating Luminescence Thermal Quenching of Praseodymium-Doped Niobo-Tantalate Phosphor through Intervalence Charge Transfer Band Displacement, Inorg. Chem., 2023, 62, 15747–15756 CrossRef CAS PubMed.
- P. Dorenbos, A Review on How Lanthanide Impurity Levels Change with Chemistry and Structure of Inorganic Compounds, ECS J. Solid State Sci. Technol., 2013, 2, R3001–R3011 CrossRef CAS.
- F. Kang, H. Zhang and L. Wondraczek,
et al., Band-Gap Modulation in Single Bi3+-Doped Yttrium–Scandium–Niobium Vanadates for Color Tuning over the Whole Visible Spectrum, Chem. Mater., 2016, 28, 2692–2703 CrossRef CAS.
- Q. Ma, Q. Zhang and M. Yang,
et al., Thermal Quenching Mechanism of Metal-Metal Charge Transfer State Transition Luminescence Based on Double-Band-Gap Modulation, Inorg. Chem., 2022, 61, 9823–9831 CrossRef CAS PubMed.
- C. Yang, N. Guo and S. Qu,
et al., Design of anti-thermal quenching Pr3+-doped niobate phosphors based on a charge transfer and intervalence charge transfer band excitation-driven strategy, Inorg. Chem. Front., 2023, 10, 4808–4818 RSC.
- X. Li, R. Hu and X. Wang,
et al., Intense mechanoluminescence and photostimulated luminescence with less afterglow in Pr3+/Gd3+ co-doped LiTaO3 phosphors, J. Lumin., 2021, 238, 118222 CrossRef CAS.
- R. Xiao, N. Guo and C. Jia,
et al., Adjustment of Bi3+ Luminescence and Thermal Quenching Properties by B’-Site Ion Substitution Strategy in Double Perovskite CaLaMgSb/TaO6:Bi3+ Phosphor, Inorg. Chem., 2023, 62, 9120–9129 CrossRef CAS PubMed.
- N. C. George, J. Brgoch and A. J. Pell,
et al., Correlating Local Compositions and Structures with the Macroscopic Optical Properties of Ce3+-Doped CaSc2O4, an Efficient Green-Emitting Phosphor, Chem. Mater., 2017, 29, 3538–3546 CrossRef CAS.
- K. A. Denault, J. Brgoch and S. D. Kloss,
et al., Average and local structure, debye temperature, and structural rigidity in some oxide compounds related to phosphor hosts, ACS Appl. Mater. Interfaces, 2015, 7, 7264–7272 CrossRef CAS PubMed.
- J. H. Tian, X. W. Sun and T. Song,
et al., Phase transition and high-pressure thermodynamic properties of CdN derived from first-principles and quasi-harmonic Debye model, Comput. Theor. Chem., 2017, 1120, 91–95 CrossRef CAS.
- J. Brgoch, C. K. Borg and K. A. Denault,
et al., An efficient, thermally stable cerium-based silicate phosphor for solid state white lighting, Inorg. Chem., 2013, 52, 8010–8016 CrossRef CAS PubMed.
- Z. Xia and A. Meijerink, Ce3+-Doped garnet phosphors: composition modification, luminescence properties and applications, Chem. Soc. Rev., 2017, 46, 275–299 RSC.
- J. Qiao, L. Ning and M. S. Molokeev,
et al., Eu2+ Site Preferences in the Mixed Cation K2BaCa(PO4)2 and Thermally Stable Luminescence, J. Am. Chem. Soc., 2018, 140, 9730–9736 CrossRef CAS PubMed.
- Z. Xia, Z. Xu and M. Chen,
et al., Recent developments in the new inorganic solid-state LED phosphors, Dalton Trans., 2016, 45, 11214–11232 RSC.
- C. Zheng, P. Xiong and M. Peng,
et al., Discovery of a novel rare-earth free narrow-band blue-emitting phosphor Y3Al2Ga3O12:Bi3+ with strong NUV excitation for LCD LED backlights, J. Mater. Chem. C, 2020, 8, 13668–13675 RSC.
- S. Ye, H. Liu and Y. Wang,
et al., Design of a Bismuth-Activated Narrow-Band Cyan Phosphor for Use in White Light Emitting Diodes and Field Emission Displays, ACS Sustainable Chem. Eng., 2020, 8, 18187–18195 CrossRef CAS.
- Z. Jiang, J. Gou and Y. Min,
et al., Crystal structure and luminescence properties of a novel non-rare-earth activated blue-emitting garnet phosphor Ca4ZrGe3O12: Bi3+ for n-UV pumped light-emitting diodes, J. Alloys Compd., 2017, 727, 63–68 CrossRef CAS.
- P. Yang, Z. Guo and Z. Sun,
et al., An insight of luminescence properties of Bi3+-activated K2BaCa(PO4)2 phosphors, Solid State Sci., 2019, 92, 1–5 CrossRef CAS.
- N. S. M. Viswanath, G. Krishnamurthy Grandhi and H. Tran Huu,
et al., Zero-thermal-quenching and improved chemical stability of a UCr4C4-type phosphor via crystal site engineering, Chem. Eng. J., 2021, 420, 127664 CrossRef CAS.
- Q. Wu, X. Chen and H. Chen,
et al., A novel pale–yellow Ba2ZnGe2O7:Bi3+ phosphor with site–selected excitation and small thermal quenching, J. Am. Ceram. Soc., 2019, 102, 6068–6076 CrossRef CAS.
- S. Wu, P. Xiong and X. Liu,
et al., Sr3Y(BO3)3:Bi3+ phosphor with excellent thermal stability and color tunability for near-ultraviolet white-light LEDs, J. Mater. Chem. C, 2021, 9, 3672–3681 RSC.
- L. Liu, P. Dai and D. Wen, Ultra-narrow-band luminescence with spectral width down to 32 nm in bismuth-activated phosphate phosphors with superior thermal stability, Mater. Today Chem., 2023, 30, 101494 CrossRef CAS.
- X. Zhao, J. Liu and Z. Guan,
et al., Upconverting ultraviolet light source with extended emission wavelengths based on Bi3+-activated phosphor, Opt. Commun., 2023, 549, 129948 CrossRef CAS.
- F. Kang, M. Peng and X. Yang,
et al., Broadly tuning Bi3+ emission via crystal field modulation in solid solution compounds (Y,Lu,Sc)VO4:Bi for ultraviolet converted white LEDs, J. Mater. Chem. C, 2014, 2, 6068–6076 RSC.
- F. Kang, M. Peng and Q. Zhang,
et al., Abnormal anti-quenching and controllable multi-transitions of Bi3+ luminescence by temperature in a yellow-emitting LuVO4:Bi3+ phosphor for UV-converted white LEDs, Chem. – Eur. J., 2014, 20, 11522–11530 CrossRef CAS PubMed.
- F. Kang and M. Peng, A new study on the energy transfer in the color-tunable phosphor CaWO4:Bi, Dalton Trans., 2014, 43, 277–284 RSC.
- T. Lyu and P. Dorenbos, Vacuum–Referred Binding Energies of Bismuth and Lanthanide Levels in LiTaO3 Perovskite: Toward Designing Energy Storage Phosphor for Anti–Counterfeiting, X–Ray Imaging, and Mechanoluminescence, Laser Photonics Rev., 2022, 16, 202200304 Search PubMed.
- Q. Ma, N. Guo and Y. Xin,
et al., Preparation of zero-thermal-quenching
tunable emission bismuth-containing phosphors through the topochemical design of ligand configuration, Inorg. Chem. Front., 2021, 8, 4072–4085 RSC.
- S. Zhou, Y. Cheng and J. Xu,
et al., Design of Ratiometric Dual-Emitting Mechanoluminescence: Lanthanide/Transition-Metal Combination Strategy, Laser Photonics Rev., 2022, 16, 202100666 Search PubMed.
- H. Suo, X. Zhao and Z. Zhang,
et al., Rational Design of Ratiometric Luminescence Thermometry Based on Thermally Coupled Levels for Bioapplications, Laser Photonics Rev., 2020, 15, 202000319 Search PubMed.
- G. Li, Q. Yang and R. Wang,
et al., Cation defects succeed in triggering super stability of narrow-band blue-emitting phosphors Ca3Sc2Si3O12: Bi3+ for backlighting display application, Ceram. Int., 2023, 49, 28159–28166 CrossRef CAS.
- H. Li, R. Pang and L. Jiang,
et al., Developing Thermally Stable Defect-Assisted Phosphors of BaSc2Ge3O10:Bi3+ for Pc-UV-LEDs Applications, Inorg. Chem., 2023, 62, 11645–11653 CrossRef CAS PubMed.
- P. Gao, Q. Li and C. Zhou,
et al., High-Efficiency Continuous-Luminescence-Controllable Performance and Antithermal Quenching in Bi3+-Activated Phosphors, Inorg. Chem., 2022, 61, 13104–13114 CrossRef CAS PubMed.
- C. Wang, Y. Cai and H. Zhang,
et al., Variation from Zero to Negative Thermal Quenching of Phosphor with Assistance of Defect States, Inorg. Chem., 2021, 60, 19365–19372 CrossRef CAS PubMed.
- Z. Liao, L. Qiu and X. Wei,
et al., Study on luminescence properties of Bi3+ doped Ba2YAlO5: A wide-band yellow emitting phosphor with excellent thermal stability, J. Alloys Compd., 2023, 938, 168648 CrossRef CAS.
- H. Yang, P. Li and X. Fu,
et al., A novel blue emitting phosphor LaScO3:Bi3+ for white LEDs: high quantum efficiency, high color purity and excellent thermal stability, Mater. Today Chem., 2022, 26, 101050 CrossRef CAS.
- H. Li, J. Cai and R. Pang,
et al., A strategy for developing thermal-quenching-resistant emission and super-long persistent luminescence in BaGa2O4:Bi3+, J. Mater. Chem. C, 2019, 7, 13088–13096 RSC.
- M. Guan, W. Wang and W. Yan,
et al., Novel narrow-band blue-emitting Cs3Zn6B9O21:Bi3+phosphor with superior thermal stability, CrystEngComm, 2020, 22, 5792–5798 RSC.
- Y. Wei, C. Heng and H. Yang,
et al., Light-Induced Charge Transfer to Achieve Deep-Red Emission in SrSc2O4:Bi toward Multiple Optical Applications, Chem. Mater., 2022, 34, 8831–8839 CrossRef CAS.
- P. Dang, D. Liu and X. Yun,
et al., Ultra-broadband cyan-to-orange emitting Ba1+xSr1−xGa4O8:Bi3+ phosphors: luminescence control and optical temperature sensing, J. Mater. Chem. C, 2020, 8, 1598–1607 RSC.
- J. Qin, P. Jiang and R. Cong,
et al., Exclusive confinement of Bi3+-activators in the triangular prism enabling efficient and thermally stable green emission in the tridymite-type phosphor CaBaGa4O8:Bi3+, Dalton Trans., 2023, 52, 11638–11648 RSC.
- Y. Wang, J. Ding and Y. Wang, Preparation and photoluminescence properties with the site–selected excitations of Bi3+–activated Ba3Sc4O9 phosphors, J. Am. Ceram. Soc., 2017, 100, 2612–2620 CrossRef CAS.
- S. Wu, P. Xiong and X. Liu,
et al., Bismuth activated high thermal stability blue-emitting phosphor Na2Y2B2O7:Bi used for near-UV white-light LEDs, J. Mater. Chem. C, 2020, 8, 16584–16592 RSC.
- R. Sun, C. Dou and F. You,
et al., Investigation on Ba2(Y1−x, Lux)5B5O17: y at.% Bi as a new type of N-UV excited high efficient blue phosphor for wLED, Opt. Mater., 2022, 129, 112542 CrossRef CAS.
- H. Li, R. Pang and G. Liu,
et al., Synthesis and Luminescence Properties of Bi3+-Activated K2MgGeO4: A Promising High-Brightness Orange-Emitting Phosphor for WLEDs Conversion, Inorg. Chem., 2018, 57, 12303–12311 CrossRef CAS PubMed.
|
This journal is © the Partner Organisations 2024 |
Click here to see how this site uses Cookies. View our privacy policy here.