DOI:
10.1039/D4QI00231H
(Research Article)
Inorg. Chem. Front., 2024,
11, 2661-2671
Adhesion, stability, structural and electronic properties of perovskite/BaWO4 heterostructures: first-principles and experimental characterizations†
Received
24th January 2024
, Accepted 18th March 2024
First published on 18th March 2024
Abstract
The poor stability of lead halide perovskites poses a critical challenge for realizing potential applications. Constructing latticed-matched perovskite/BaWO4 heterostructures is highly advantageous for achieving enhanced stability. However, there is limited knowledge about the interfacial properties. Herein, we conducted combined first-principles and experimental investigations of the perovskite/BaWO4 heterostructures in this work. First, eight different interfacial configurations were constructed and investigated systematically. The influence of surface terminations on interfacial properties was examined through the adhesive strength and electronic structures. The results of adhesion work suggest that the MAPbBr3/BaWO4 configurations possess stronger interfacial interaction than the CsPbBr3/BaWO4 configurations. The formation of BaW/PbBr and BaW/MAPb configurations was more facile compared to their counterparts. The interfacial charge transfer direction, potential difference, and valence band edges of the perovskite/BaWO4 heterostructures were found to be significantly influenced by the surface termination of BaWO4. The absorption intensity of the perovskite/BaWO4 heterostructures is significantly affected by the surface termination of the perovskite. Finally, the CsPbBr3/BaWO4 heterostructure was fabricated and characterized, thereby validating the first-principles predictions. This study provides a fundamental contribution to establishing perovskite/BaWO4 heterostructures for the advancement of perovskite-based optoelectronic devices.
1. Introduction
During the past decade, lead bromide perovskites in the guise of colloidal nanocrystals (NCs), such as cesium lead bromide (CsPbBr3) and methylammonium lead bromide (MAPbBr3), have been extensively explored for diverse applications, such as light-emitting diodes (LEDs) and photodetectors, owing to their distinctive photophysical characteristics including tunable band gap, high absorption coefficient, strong defect tolerance, high quantum yield, low fabrication cost, and so on.1–5 Unfortunately, the excellent properties and exciting progress of lead bromide perovskite NCs are overshadowed by their limited operational lifetime under ambient conditions, which is severely hindering their real-world implementation and widespread adoption. The instability of perovskite NCs renders them vulnerable to environmental factors, which results in a series of detrimental effects, including structural alterations and phase transitions, reduced luminescence efficiency, and limited electron mobility.6–10 An efficient way to enhance the stability of perovskite NCs and preserve their colloidal stability and photoluminescence is to couple them with another material to form a heterostructure.11–16 Although perovskite NC heterostructures have been successfully designed to enhance their optical stability and emission intensity, their applications are still limited to a few combinations.17–22 The design of perovskite NC heterostructures in combination with lattice-matched materials is still a topic that requires further investigation.
Barium tungstate (BaWO4) with a scheelite structure is known for its high ionic/electronic conductivity and high thermal/chemical stability, and has potential applications in catalysis.23–26 Recently, the heterostructural growth of CsPbBr3 and MAPbBr3 NCs on a BaWO4 crystal was successfully demonstrated based on lattice matching.27,28 Compared to the pure perovskite, the perovskite/BaWO4 heterostructures exhibited enhanced luminescence intensity and sustained stability against UV-light radiation, heat, and diverse solvents. The BaWO4 crystal can effectively inhibit halogen migration in the perovskite, leading to tunable emissions with different compositions. This implies the great potential of combining barium tungstate with lead bromide perovskite in advanced anticounterfeiting and encryption applications. To date, the CsPbBr3/BaWO4 and MAPbBr3/BaWO4 heterostructures have been examined by experimental investigations.27,28 The interfacial characteristics of these heterostructures, which are directly determined by the arrangement of atoms and their interactions, are still not fully understood. Systematic and comparative investigations of the interfacial systems are therefore of great interest.29–33 In this study, we utilize the first-principles approach to explore the heterostructures of CsPbBr3/BaWO4 and MAPbBr3/BaWO4. Moreover, the composition and morphological characteristics were further verified by microscopic and spectroscopic tools. The interfacial behavior and mechanism of the heterostructure were investigated by combining theoretical and experimental approaches to assess the photocatalytic potential of these newly developed heterostructures.
2. Computational and experimental details
The first-principles density functional theory (DFT) calculations were carried out based on projector augmented wave (PAW) formalism34 within the Vienna ab initio simulation package (VASP, version 5.4.4).35–37 The exchange correlation effect was evaluated by means of the generalized gradient approximation (GGA) of the Perdew–Burke–Ernzerhof (PBE) functional.38 The kinetic plane wave energy cutoff was set to 450 eV with Gaussian smearing (σ = 0.1 eV).39 Brillouin-zone integration was done using the Monkhorst–Pack40k-point mesh with 0.02 Å−1 separation. The vacuum layer thickness was set to 15 Å to neglect the coupling between the images. The spurious interactions between periodic images were significantly reduced by using the dipole correction in the VASP (IDIPOL = 3). The DFT-D3 with the BJ-damping method41 was included to consider the van der Waals (vdW) interactions. The convergence criteria for energy and force were 10−6 eV and 0.005 eV Å−1, respectively. During the geometric optimizations, the bottom layers were constrained to the bulk geometry, while the remaining layers were fully relaxed. The VESTA (Visualization for Electronic and Structural Analysis) program42 was utilized to visualize the crystallographic, volumetric, and morphological data.
In this study, we adopted the cubic structure (space group Pm
m) for CsPbBr3, the monoclinic (space group P21/m) structure for MAPbBr3, and the tetragonal (space group I41/a) structure for BaWO4. The atomic structures and lattice parameters derived from first-principles simulations are provided in Fig. S1 and Table S1,† exhibiting excellent agreement with the corresponding experimental results.43–45 Following the rigorous lattice matching principle, the (200) in-plane lattice spacing in BaWO4 closely matched those of CsPbBr3 and MAPbBr3, serving as the foundation for constructing the perovskite/BaWO4 heterostructures. The perovskite (200) slabs were constructed employing a stoichiometric six-layer slab, while the BaWO4 (200) slab was generated using an eleven-layer symmetric slab. CsPbBr3 (or MAPbBr3) exhibits two potential surface terminations: the termination by Cs (or MA) cations, and the termination by Pb cations. In contrast, the BaWO4 slab features two distinct surface types: BaW- and O-terminated surfaces. By combining the perovskite and BaWO4 slabs, we generated a total of eight lattice-matched interfacial configurations, as depicted in Fig. 1. The lattice parameters and mean absolute strain values for the eight models are presented in Table S2.†
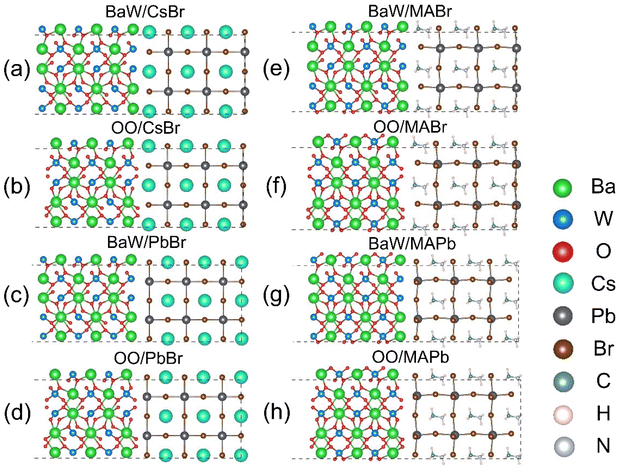 |
| Fig. 1 Schematic illustration of the perovskite/BaWO4 interfaces: (a) BaW/CsBr, (b) OO/CsBr, (c) BaW/PbBr, (d) OO/PbBr, (e) BaW/MABr, (f) OO/CsBr, (g) BaW/MAPb, and (h) OO/MAPb. | |
The CsPbBr3/BaWO4 heterostructure was prepared through an in situ synthesis thermal-assisted method. The stoichiometric proportions of Na2WO4·2H2O (1 mmol) and Ba(NO3)2 (1 mmol) were dissolved in a 20 ml aqueous solution and stirred in a 50 ml beaker, immediately resulting in a white BaWO4 precipitate. After centrifugation and drying, 145 mg of the precipitate was weighed and put together with PbBr2 (0.8 mmol) into a three-neck flask with 20 ml of octadecene as the solvent, then heated to 120 °C with nitrogen protection; the air and water impurities in the three-neck flask were extracted. The reaction mixture was kept for 30 min, heated up to 180 °C, and immediately infused with 3 ml of Cs-OA to obtain a yellow solution, which was reacted for 10 seconds. The solution was cooled to room temperature with a cold-water bath, and the precipitates were centrifuged and washed with n-hexane three times, toluene twice, anhydrous ethanol once, and finally vacuum dried at 60 °C for 8 hours, to obtain the CsPbBr3/BaWO4 heterostructure.
3. Results and discussion
3.1. Interfacial adhesion
The work of adhesion (Wad)46–48 is commonly employed to characterize the interfacial stability and bonding strength. The work of interfacial adhesion can be described as the reversible energy necessary to divide the heterostructure into two free surfaces, quantified by the following equation: | Wad = [EPVK + EBWO − Etotal]/A | (1) |
where EPVK and EBWO correspond to the total energy of the fully relaxed perovskite and BaWO4 slabs, respectively. Etotal represents the total energy of the perovskite/BaWO4 interface, while A specifies the interface area. It is widely accepted that a higher Wad value corresponds to enhanced bonding strength. The calculated Wad values of the CsPbBr3/BaWO4 and MAPbBr3/BaWO4 heterostructures are compared in Table 1. The formation of all eight observed interfacial configurations is facilitated by the positive adhesion work. The perovskite/BaWO4 interface exhibits larger Wad values when compared to the weak perovskite/MXene system,30 while exhibiting equivalent Wad values to the strong perovskite/Ga2O3 system,31 thereby suggesting a high degree of interface stability. Among them, the MAPbBr3/BaWO4 interface exhibits larger Wad values, ranging from 0.014 to 0.052 eV Å−2, when compared to those of the CsPbBr3/BaWO4 interface, which span from 0.008 to 0.035 eV Å−2. This comparison further suggests that the MAPbBr3/BaWO4 structure possesses a relatively higher degree of stability. The comprehensive investigations indicate that the Wad values at the interfaces between BaW- and Pb-terminations are higher compared to the other three interfaces, regardless of CsPbBr3/BaWO4 and MAPbBr3/BaWO4. Therefore, the formation of BaW/PbBr and BaW/MAPb interfaces was more facile compared to their counterparts in experiments. The stability of the interface correlates with the extent of atomic rearrangement.
Table 1 Calculated adhesion work (eV Å−2), charge transfer (e), plane-averaged electrostatic potential difference (eV) and work function (eV) of the relaxed perovskite/BaWO4 interfaces
Interface |
Adhesion work |
Charge transfera |
Potential difference |
Work function |
The positive values indicate charge transfer from perovskite to BaWO4, whereas the negative values exhibit charge transfer from BaWO4 to perovskite.
|
BaW/CsBr |
0.018 |
−0.2 |
2.0 |
2.8 |
OO/CsBr |
0.024 |
2.0 |
1.0 |
6.7 |
BaW/PbBr |
0.035 |
−1.5 |
2.0 |
2.6 |
OO/PbBr |
0.008 |
1.8 |
1.0 |
6.5 |
BaW/MABr |
0.036 |
−0.6 |
1.0 |
2.8 |
OO/MABr |
0.014 |
0.7 |
−0.5 |
6.7 |
BaW/MAPb |
0.052 |
−1.4 |
1.0 |
2.5 |
OO/MAPb |
0.026 |
1.9 |
−0.5 |
6.7 |
To clarify the bonding mechanism in the perovskite/BaWO4 heterostructures, the electron localization function (ELF),49 as well as the atomic structures of CsPbBr3/BaWO4 and MAPbBr3/BaWO4 systems, was analyzed, as shown in Fig. 2. In the colorimetric representation of electron localization, red is used to represent electrons with a high degree of localization, while blue is used to represent electrons with a high degree of delocalization. Localization and delocalization represent the spatial distribution patterns of electrons within the system. Localization signifies the concentration of electron density in specific regions, whereas delocalization denotes the free movement of electrons throughout the system, indicating enhanced electron mobility. The nearly spherical electron distribution indicates the strong ionic bonding character between Pb and Br atoms. In contrast, the BaWO4 side exhibits the covalent bonding for the WO4 anions and the ionic bonding character for the Ba cations. The MAPbBr3/BaWO4 configurations with less blue area in the interface region indicate stronger interfacial interaction than the CsPbBr3/BaWO4 configurations. Upon closer inspection of the interface region, noticeable distortions of the Pb-Br6 octahedra in the cubic structure can be observed.50 These distortions are attributed to the strong interactions between BaWO4 and perovskite. Moreover, the CsPbBr3/BaWO4 configurations undergo more significant distortions compared to the MAPbBr3/BaWO4 configurations. Among the various terminations, the BaW/PbBr and BaW/MAPb configurations with the highest Wad values demonstrate more significant distortions compared to their counterparts, which is coincident with the results in Table 1. To provide a quantitative measure of charge transfer between perovskite and BaWO4, we conducted Bader charge analysis51 on representative atoms. The values of calculated charge transfer are presented in Table 1. The charge transfer direction is significantly influenced by the surface termination, irrespective of the CsPbBr3/BaWO4 or MAPbBr3/BaWO4 systems. Specifically, the BaW/perovskite (BaW/CsBr, BaW/PbBr, BaW/MABr, and BaW/MAPb) configurations exhibit electron transfer from BaWO4 to the perovskite layer, whereas the OO/perovskite (OO/CsBr, OO/PbBr, OO/MABr, and OO/MAPb) configurations indicate charge transfer from the perovskite to the BaWO4 layer. The above results can be attributed to the interfacial bonding between perovskite and BaWO4.
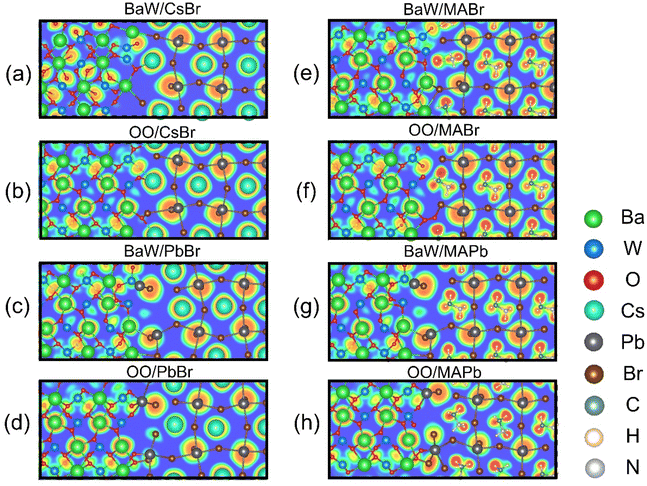 |
| Fig. 2 ELF of the relaxed perovskite/BaWO4 interfaces: (a) BaW/CsBr, (b) OO/CsBr, (c) BaW/PbBr, (d) OO/PbBr, (e) BaW/MABr, (f) OO/CsBr, (g) BaW/MAPb, and (h) OO/MAPb. | |
3.2. Electronic structures
To thoroughly elucidate the intricate characteristics of interface coupling and charge redistribution within the perovskite/BaWO4 heterostructures, we explored the z-axis dependence of the three-dimensional and plane-averaged charge density difference (Δρ).52 The yellow-colored segment signifies charge accumulation, whereas the cyan-colored segment denotes charge depletion. Fig. 3 suggests that the interface region is the primary site for charge transfer and redistribution. The magnitude of the charge distribution at the interface is significantly influenced by the strength of the interfacial interaction. The BaW/PbBr and BaW/MAPb configurations exhibit denser electron isosurfaces compared to the other configurations, consistent with their highest Wad values listed in Table 1. The plane-averaged charge density difference curves obtained from isosurfaces exhibit distinct trends depending on various surface terminations. The curves of the OO/perovskite (OO/CsBr, OO/PbBr, OO/MABr, and OO/MAPb) configurations exhibit a similar pattern, with electron accumulation on the BaWO4 side and depletion on the perovskite side, indicating a distinct contrast at the interface. In contrast, the curves of BaW/perovskite (BaW/CsBr, BaW/PbBr, BaW/MABr, and BaW/MAPb) configurations display an irregular distribution, signifying complex charge redistribution at the interface regions. The above results are entirely consistent with the direction of charge transfer presented in Table 1. The OO/perovskite configurations are bonded with the Cs–O and Pb–O bonds. Such configurations under O-rich conditions contain more interfacial bonds and exhibit distinct charge redistributions at the interface. The accumulation of various carriers at the interfaces on both ends of the heterointerface leads to the formation of a built-in electric field, which is directed from the hole accumulation region to the electron accumulation area.53
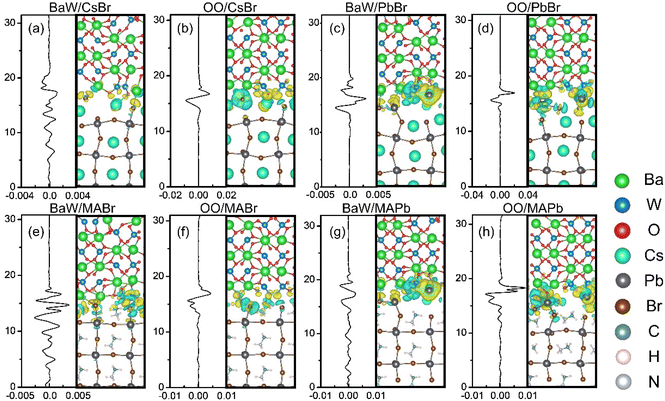 |
| Fig. 3 Plane-averaged electron density difference Δρ(z) and 3D charge density difference (0.002 e Å−3) of the relaxed perovskite/BaWO4 interfaces: (a) BaW/CsBr, (b) OO/CsBr, (c) BaW/PbBr, (d) OO/PbBr, (e) BaW/MABr, (f) OO/CsBr, (g) BaW/MAPb, and (h) OO/MAPb. | |
To offer a comprehensive assessment of electronic transport behavior at the perovskite/BaWO4 heterostructures, the plane-averaged electrostatic potentials in the vertical direction were analyzed and are plotted in Fig. 4. The band alignment and the work function of the perovskite/BaWO4 heterostructures can be estimated via the electrostatic potentials.54 Evaluation of the potential offset and work function of the heterostructures is based on the theoretical framework of the plane-averaged electrostatic potentials. When two distinct surfaces come into contact and create heterostructures, the disparity in Fermi energy leads to electron transport at the heterointerface, resulting in band offsets. As shown in Fig. 4, a notable contrast exists in the atomic arrangements of perovskite and BaWO4, with atoms near the interface undergoing substantial migration toward their final stable state, ultimately leading to a macroscopic discrepancy in the plane-averaged electrostatic potentials. The plane-averaged electrostatic potential difference (ΔV) between the perovskite and BaWO4 was estimated and is summarized in Table 1. The positive value indicates that the plane-averaged potentials of the BaWO4 side are higher than those at the perovskite side. The potential offset, influenced by the atomic configurations near the interface, plays a crucial role in shaping the electronic barrier and ultimately affects the device performance.55 The BaW/perovskite configurations display greater ΔV compared to the OO/perovskite configurations, irrespective of whether CsPbBr3 or MAPbBr3 is involved. Simultaneously, the CsPbBr3/BaWO4 configurations show a larger ΔV compared to the MAPbBr3/BaWO4 configurations. In particular, for the OO/MABr and OO/MAPb configurations, negative ΔV values are observed, indicating a reversal in the potential offset. The work function signifies the minimum energy needed to extract electrons from the Fermi level to reach the vacuum level. Table 1 details the work functions of the corresponding perovskite/BaWO4 heterostructures. Importantly, the work functions of the OO/perovskite configurations undergo substantial modulation towards higher values, presenting a stark contrast to the work function observed in the BaW/perovskite configurations. The results of potential differences and work function measurements exhibit a high degree of consistency with the charge transfer data outlined in Table 1. The considerably altered work function indicates that surface engineering holds promise as an effective approach for achieving ohmic contact of n- and p-type.
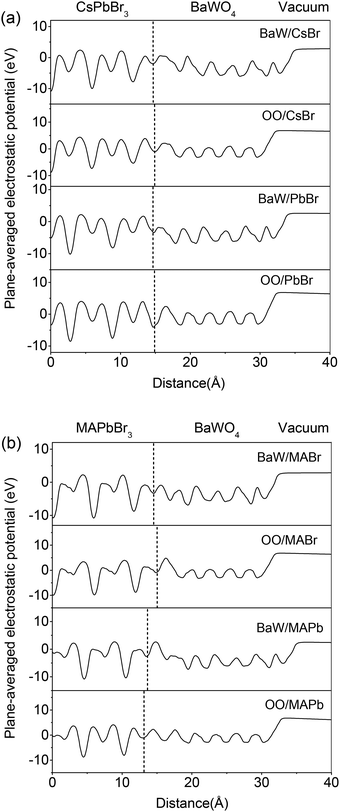 |
| Fig. 4 Plane-averaged electrostatic potential of the relaxed perovskite/BaWO4 interfaces: (a) CsPbBr3/BaWO4 and (b) MAPbBr3/BaWO4. | |
The density of states (DOS) provides a quantitative description of the electronic states in different orbitals, which is essential for a comprehensive understanding of the interfacial bonding mechanism. For a more in-depth exploration of the perovskite/BaWO4 heterostructures, we calculated and depicted the atom-projected DOS in Fig. 5. More details of the calculated DOS for CsPbBr3, MAPbBr3, and BaWO4 in the bulk phase are plotted in Fig. S2.† As a member of the scheelite family, BaWO4 has a larger band gap compared to CsPbBr3 (or MAPbBr3).56 The calculated DOS of the perovskite/BaWO4 heterostructures shows that the top of the valence band is entirely composed of O 2p and Br 4p orbitals while the bottom of the conduction band is dominated by the W 5d orbitals. The formation of the perovskite/BaWO4 heterointerface is a consequence of the hybridization of Br 4p and W 5d states. The eight different interfacial configurations exhibit similar DOS features. The OO/perovskite configurations are identified as belonging to a type-II heterostructure, with the conduction band minimum (CBM) contributed by the perovskite and the valence band maximum (VBM) contributed by BaWO4. A close examination of the top of the valence band reveals that the O 2p and peaks move toward the lower energy region for the BaW/perovskite configurations. In particular, the BaW/PbBr and BaW/MAPb configurations exhibit the largest peak shift,57 suggesting enhanced binding energy between the two materials at the interface. The observation of interfacial defect states across the band gap of the BaW/perovskite configurations also suggests that hybridization is enhanced at the interface. Hence, the typical type-II band alignment observed in the OO/perovskite configurations was transformed into the typical type-I band alignment in the BaW/perovskite configurations. This is consistent with the results of charge transfer, potential difference and work function as outlined in Table 1. The findings imply that the electronic structures of the perovskite/BaWO4 heterostructures are dependent on the surface termination of BaWO4, not that of perovskite. The surface termination of BaWO4 has crucial potential significance in the transformation of a type-I heterostructure to a type-II heterostructure.
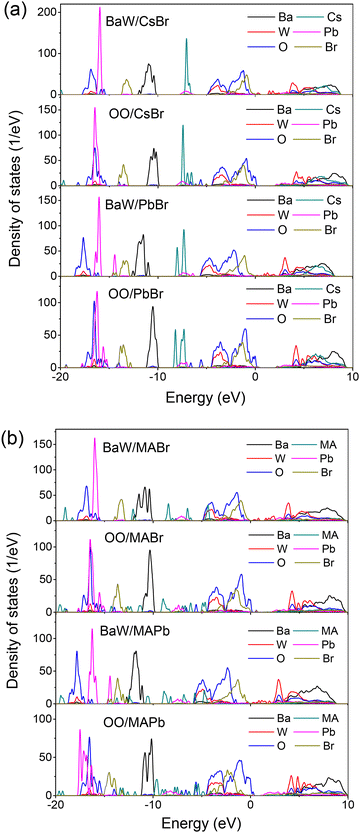 |
| Fig. 5 DOS of the relaxed perovskite/BaWO4 interfaces: (a) CsPbBr3/BaWO4 and (b) MAPbBr3/BaWO4. | |
3.3. Optical properties
The light absorption coefficient of the perovskite/BaWO4 heterostructures is a key parameter for evaluating their optical response to sunlight. The absorption spectra of the CsPbBr3/BaWO4 and MAPbBr3/BaWO4 heterostructures are calculated and displayed as in Fig. 6. For comparison, the light absorption spectra of CsPbBr3, MAPbBr3, and BaWO4 in the bulk phase were calculated, which are shown in Fig. S3.† The shapes of the absorption curves of CsPbBr3/BaWO4 and MAPbBr3/BaWO4 heterostructures are quite similar. The optical absorption spectrum of the heterostructures shows three distinct peaks at 3.5, 5.5, and 9.5 eV. The first peak at 3.5 eV is primarily due to the transition from Br 4p to Pb 6p states between the conduction and valence bands.58 The second peak around 5.5 eV is attributed to the optical transition from O 2p orbitals to W 5d orbitals. The third peak around 9.5 eV originates from the transition from hybridized W 5d and O 2p states to W 5d states.59 A close inspection of the absorption spectra reveals that the absorption intensity of the perovskite exhibits a similar trend for both CsPbBr3/BaWO4 (BaW/CsBr > OO/CsBr > BaW/PbBr > OO/PbBr) and MAPbBr3/BaWO4 (BaW/MABr > OO/MABr > BaW/MAPb > OO/MAPb). The BaW/CsBr heterostructure (or BaW/MABr) exhibits the lowest interfacial distortion and remarkably well-preserved perovskite-type structures, leading to a significantly higher absorption coefficient. These results suggest that the optical properties of the perovskite/BaWO4 heterostructures are significantly affected by the surface termination of the perovskite layers, but not the BaWO4 layers. To improve the device performance through interface modification, focusing on the perovskite layer should be a promising strategy.
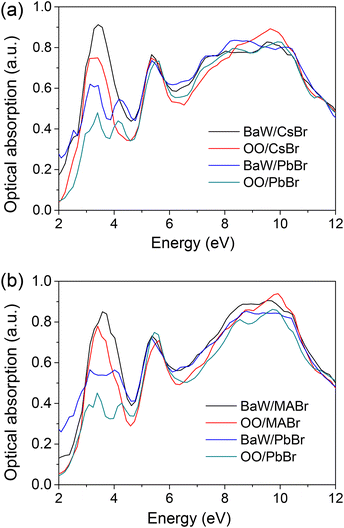 |
| Fig. 6 Absorption coefficients of the relaxed perovskite/BaWO4 interfaces: (a) CsPbBr3/BaWO4 and (b) MAPbBr3/BaWO4. | |
3.4. Experimental verification
The CsPbBr3/BaWO4 heterostructure was successfully fabricated to further verify the first-principles predictions. The X-ray diffraction (XRD) patterns of CsPbBr3, BaWO4, and CsPbBr3/BaWO4 are displayed in Fig. 7. The XRD pattern showed diffraction peaks at 2θ values of 15.1°, 21.3°, 30.6°, 37.7°, and 43.6° corresponding to the (100), (110), (200), (211), and (220) phases of the typical cubic CsPbBr3, respectively.60,61 In addition, the diffraction peaks at 17.3°, 27.9°, 31.7°, 42.8°, 45.6°, and 53.5° are attributed to the (101), (112), (200), (204), (220), and (312) phases of the tetragonal BaWO4, respectively.62 All diffraction peaks are consistent with the crystalline phase of CsPbBr3 (JCPDS No. 18-0364) and BaWO4 (JCPDS No. 43-0646). The results showed that CsPbBr3 and BaWO4 exhibited high crystallinity with well-maintained cubic perovskite and tetragonal scheelite crystal phases during the formation of the CsPbBr3/BaWO4 heterostructures.
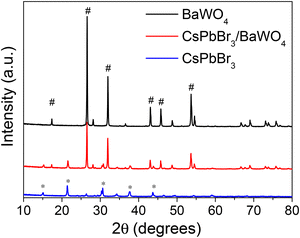 |
| Fig. 7 Experimental XRD patterns of CsPbBr3, BaWO4, and CsPbBr3/BaWO4. | |
To evaluate the chemical bond formation and interactions, FT-IR spectral analysis of CsPbBr3, BaWO4, and CsPbBr3/BaWO4 was performed and the results are displayed in Fig. 8. The spectrum of BaWO4 reveals a vibrational mode at 820 cm−1, associated with asymmetric W–O vibrations, thereby confirming the presence of the typical WO4 in the prepared BaWO4 material.63,64 The additional absorption peak of BaWO4 around 1400 cm−1 may be attributed to the stretching vibrations of the organic groups present in the precursor. Two small peaks are recognized around 1600 and 2900 cm−1 in the spectrum of CsPbBr3. The peak at 1600 cm−1 can be assigned to the N–H bending vibrations of the ligands, whereas the peak at 2900 cm−1 is associated with the oleyl group.65 The FT-IR analysis confirms the formation of the CsPbBr3/BaWO4 heterostructures.
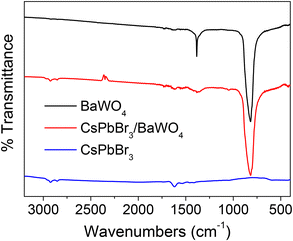 |
| Fig. 8 FT-IR patterns of CsPbBr3, BaWO4, and CsPbBr3/BaWO4. | |
The structural features of the prepared CsPbBr3/BaWO4 heterostructures were carefully identified by scanning electron microscopy (SEM) and transmission electron microscopy (TEM), as demonstrated in Fig. 9. The TEM images clearly depict the CsPbBr3 and BaWO4 materials. Specifically, the analysis indicates that the interplanar spacings of 2.9 Å correspond to the CsPbBr3 (200) plane,66 whereas the interplanar spacings of 2.7 Å correspond to the BaWO4 (200) plane,67 respectively. The confirmation of lattice-matched growth between CsPbBr3 and BaWO4 is intricately validated through TEM observations, closely aligning with the predictions derived from first-principles calculations.
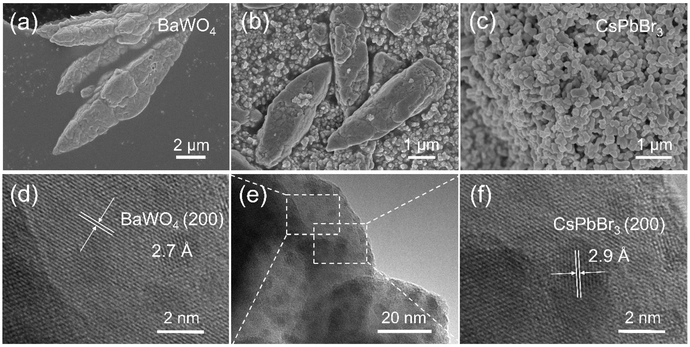 |
| Fig. 9 SEM images of (a) BaWO4, (b) CsPbBr3/BaWO4, and (c) CsPbBr3; TEM images of (d) BaWO4, (e) CsPbBr3/BaWO4, and (f) CsPbBr3. | |
4. Conclusions
To summarize, first-principles and experimental studies were employed to investigate the interfacial properties between perovskite and BaWO4. The interfacial properties include atomic arrangement, adhesion work, charge redistribution, potential difference, work function, and their orbital hybridization, as well as their optical absorption. The results indicate that the MAPbBr3/BaWO4 configurations possess stronger interfacial interaction than the CsPbBr3/BaWO4 configurations. The formation of BaW/PbBr and BaW/MAPb configurations was more facile compared to their counterparts. Our study highlights the importance of surface termination for the interfacial properties. The interfacial charge transfer direction, potential difference, and band alignment of the perovskite/BaWO4 heterostructures were found to be significantly influenced by the surface termination of BaWO4. The absorption intensity of the perovskite/BaWO4 heterostructures is significantly affected by the surface termination of the perovskite. The lattice-matching growth of CsPbBr3/BaWO4 was achieved and the existence of the heterostructure was confirmed by XRD, SEM, and TEM characterization. This study helps us to gain better insight into the nature of the perovskite/BaWO4 heterostructures, thereby laying the groundwork for the realization of high-performance perovskite-based devices.
Conflicts of interest
The authors declare that they have no known competing financial interests or personal relationships that could have appeared to influence the work reported in this paper.
Acknowledgements
This work was supported by the National Natural Science Foundation of China (Grant No. 12381240132), the China Scholarship Council (Grant No. 202308410380), the Postgraduate Education Reform and Quality Improvement Project of Henan Province (Grant No. YJS2023JD60), and the Henan International Joint Laboratory of Nanocomposite Sensing Materials.
References
- S. R. Smock, Y. Chen, A. J. Rossini and R. L. Brutchey, The surface chemistry and structure of colloidal lead halide perovskite nanocrystals, Acc. Chem. Res., 2021, 54(3), 707–718 CrossRef CAS PubMed.
- P. Liu, W. Chen, W. Wang, B. Xu, D. Wu, J. Hao, W. Cao, F. Fang, Y. Li, Y. Zeng, R. Pan, S. Chen, W. Cao, X. W. Sun and K. Wang, Halide-rich synthesized cesium lead bromide perovskite nanocrystals for light-emitting diodes with improved performance, Chem. Mater., 2017, 29(12), 5168–5173 CrossRef CAS.
- G. Xiao, Y. Cao, G. Qi, L. Wang, C. Liu, Z. Ma, X. Yang, Y. Sui, W. Zheng and B. Zou, Pressure effects on structure and optical properties in cesium lead bromide perovskite nanocrystals, J. Am. Chem. Soc., 2017, 139(29), 10087–10094 CrossRef CAS PubMed.
- Y. Cai, W. Li, D. Tian, S. Shi, X. Chen, P. Gao and R.-J. Xie, Organic sulfonium-stabilized high-efficiency cesium or methylammonium lead bromide perovskite nanocrystals, Angew. Chem., Int. Ed., 2022, 61(37), e202209880 CrossRef CAS PubMed.
- S. Wang, A. A. Yousefi Amin, L. Wu, M. Cao, Q. Zhang and T. Ameri, Perovskite nanocrystals: Synthesis, stability, and optoelectronic applications, Small Struct., 2021, 2(3), 2000124 CrossRef CAS.
- G. H. Ahmed, J. Yin, O. M. Bakr and O. F. Mohammed, Successes and challenges of core/shell lead halide perovskite nanocrystals, ACS Energy Lett., 2021, 6(4), 1340–1357 CrossRef CAS.
- C. Otero-Martínez, N. Fiuza-Maneiro and L. Polavarapu, Enhancing the intrinsic and extrinsic stability of halide perovskite nanocrystals for efficient and durable optoelectronics, ACS Appl. Mater. Interfaces, 2022, 14(30), 34291–34302 CrossRef PubMed.
- K. Xing, S. Cao, X. Yuan, R. Zeng, H. Li, B. Zou and J. Zhao, Thermal and photo stability of all inorganic lead halide perovskite nanocrystals, Phys. Chem. Chem. Phys., 2021, 23(32), 17113–17128 RSC.
- M. Liu, Q. Wan, H. Wang, F. Carulli, X. Sun, W. Zheng, L. Kong, Q. Zhang, C. Zhang, Q. Zhang, S. Brovelli and L. Li, Suppression of temperature quenching in perovskite nanocrystals for efficient and thermally stable light-emitting diodes, Nat. Photonics, 2021, 15(5), 379–385 CrossRef CAS.
- C. Zhang, S. Wang, X. Li, M. Yuan, L. Turyanska and X. Yang, Core/shell perovskite nanocrystals: Synthesis of highly efficient and environmentally stable FAPbBr3/CsPbBr3 for LED applications, Adv. Funct. Mater., 2020, 30(31), 1910582 CrossRef CAS.
- S. Bera and N. Pradhan, Perovskite nanocrystal heterostructures: Synthesis, optical properties, and applications, ACS Energy Lett., 2020, 5(9), 2858–2872 CrossRef CAS.
- A. Kipkorir, J. DuBose, J. Cho and P. V. Kamat, CsPbBr3–CdS heterostructure: Stabilizing perovskite nanocrystals for photocatalysis, Chem. Sci., 2021, 12(44), 14815–14825 RSC.
- L. Ruan and Y. Zhang, NIR-excitable heterostructured upconversion perovskite nanodots with improved stability, Nat. Commun., 2021, 12(1), 219 CrossRef CAS PubMed.
- C. Yuan, Y. He, R. Chen, Y. Sun, J. Li, W. Cui, P. Chen, J. Sheng and F. Dong, Perovskite nanocrystals-based heterostructures: synthesis strategies, interfacial effects, and photocatalytic applications, Sol. RRL, 2021, 5(2), 2000419 CrossRef CAS.
- L. Rao, Q. Zhang, B. Sun, M. Wen, J. Zhang, S. Yu, T. Fu and X. Niu, CsPbBr3/Cs4PbBr6 heterostructure solids with high stability and photoluminescence for white light-emitting diodes, J. Alloys Compd., 2022, 919, 165857 CrossRef CAS.
- S. Wang, C. Bi, A. Portniagin, J. Yuan, J. Ning, X. Xiao, X. Zhang, Y. Y. Li, S. V. Kershaw, J. Tian and A. L. Rogach, CsPbI3/PbSe heterostructured nanocrystals for high-efficiency solar cells, ACS Energy Lett., 2020, 5(7), 2401–2410 CrossRef CAS.
- L. Ruan and Y. Zhang, Upconversion perovskite nanocrystal heterostructures with enhanced luminescence and stability by lattice matching, ACS Appl. Mater. Interfaces, 2021, 13(43), 51362–51372 CrossRef CAS PubMed.
- L. Zhang, Y. Liu, Y. He, X. Zhang, C. Geng, R. Yang and S. Xu, Stable CsPbX3/ZnO heterostructure nanocrystals for light-emitting application, J. Phys. Chem. Lett., 2021, 12(45), 10953–10957 CrossRef CAS PubMed.
- Q. Zhang, Y. Zhou, Y. Wei, M. Tai, H. Nan, Y. Gu, J. Han, X. Yin, J. Li and H. Lin, Improved phase stability of γ-CsPbI3 perovskite nanocrystals using the interface effect using iodine modified graphene oxide, J. Mater. Chem. C, 2020, 8(7), 2569–2578 RSC.
- M. Imran, L. Peng, A. Pianetti, V. Pinchetti, J. Ramade, J. Zito, F. Di Stasio, J. Buha, S. Toso, J. Song, I. Infante, S. Bals, S. Brovelli and L. Manna, Halide perovskite–lead chalcohalide nanocrystal heterostructures, J. Am. Chem. Soc., 2021, 143(3), 1435–1446 CrossRef CAS PubMed.
- J. Ghosh, L. P. L. Mawlong, G. B. Manasa, A. J. Pattison, W. Theis, S. Chakraborty and P. K. Giri, Solid-state synthesis of stable and color tunable cesium lead halide perovskite nanocrystals and the mechanism of high-performance photodetection in a monolayer MoS2/CsPbBr3 vertical heterojunction, J. Mater. Chem. C, 2020, 8(26), 8917–8934 RSC.
- S. Gull and G. Li, Deep red emission from Cs4PbI6/CsPbI3/ZnS heterostructure for enhanced stability and photoluminescence quantum yield, Ceram. Int., 2023, 49(4), 5915–5921 CrossRef CAS.
- D. Sivaganesh, S. Saravanakumar, V. Sivakumar, R. Sangeetha, L. J. Berchmans, K. S. S. Ali and A. M. Alshehri, Effect of preparation techniques on BaWO4: Structural, morphological, optical and electron density distribution analysis, J. Mater. Sci.: Mater. Electron., 2021, 32(2), 1466–1475 CrossRef CAS.
- A. Azzouzi, M. Benchikhi and R. El Ouatib, Room-temperature co-precipitation synthesis of (Ca,Sr,Ba)WO4 solid solutions: Structural refinement, morphology and band gap tuning, Ceram. Int., 2020, 46(15), 23706–23718 CrossRef CAS.
- M. Kowalkińska, P. Głuchowski, T. Swebocki, T. Ossowski, A. Ostrowski, W. Bednarski, J. Karczewski and A. Zielińska-Jurek, Scheelite-type wide-bandgap ABO4 compounds (A = Ca, Sr, and Ba; B = Mo and W) as potential photocatalysts for water treatment, J. Phys. Chem. C, 2021, 125(46), 25497–25513 CrossRef.
- S. Tang, S. Wang, X. Yu, H. Gao, X. Niu, Y. Wang, X. Zhao, G. Sun and D. Li, Gamma-ray irradiation assisted polyacrylamide gel synthesis of scheelite type BaWO4 phosphors and its colorimetric, optical and photoluminescence properties, ChemistrySelect, 2020, 5(34), 10599–10606 CrossRef CAS.
- P. Liu, Y. Xu, Y. Zhang, L. Han, H. Lian and J. Lin, Epitaxial growth of lattice-matched BaWO4/CH3NH3PbX3 (X = Cl, Br, I) hetero-micro/nanostructure with suppressed halide ion migration, Adv. Opt. Mater., 2023, 11(12), 2300440 CrossRef CAS.
- P. Liu, Y. Xu, B. Li, Y. Zhang, H. Lian and J. Lin, Structural engineering of BaWO4/CsPbX3/CsPb2X5 (X = Cl, Br, I) heterostructures towards ultrastable and tunable photoluminescence, Nano Res., 2024, 17(3), 1636–1645 CrossRef CAS.
- P. Shi, Y. Ding, B. Ding, Q. Xing, T. Kodalle, C. M. Sutter-Fella, I. Yavuz, C. Yao, W. Fan, J. Xu, Y. Tian, D. Gu, K. Zhao, S. Tan, X. Zhang, L. Yao, P. J. Dyson, J. L. Slack, D. Yang, J. Xue, M. K. Nazeeruddin, Y. Yang and R. Wang, Oriented nucleation in formamidinium perovskite for photovoltaics, Nature, 2023, 620(7973), 323–327 CrossRef CAS PubMed.
- L. Fang, Y. Guo, S. Zhang, Y. Lv, Y. Xue, X. Bai, J. Li, C. Lai and Y. Wang, Influence of transition metal substitution on Cs2AgBiBr6/M3C2 (M = Ti, V, Cr, Zr, Nb, Mo, Hf, Ta, and W) interfaces: First-principles and experimental studies, Appl. Surf. Sci., 2023, 641, 158538 CrossRef CAS.
- Y. Guo, L. Fang, Q. Li, X. Bai, Y. Xue, C. Lai and Y. Wang, Insight into the interface engineering between methylammonium lead halide perovskites and gallium oxide: A first-principles approach, Phys. Chem. Chem. Phys., 2023, 25(46), 31804–31812 RSC.
- Y. Ma, G. Han, M. Yang, M. Guo, Y. Xiao, Y. Guo and W. Hou, Inhibiting Li+ migration by thenoyltrifluoroacetone toward efficient and stable perovskite solar cells, Inorg. Chem. Front., 2023, 10(8), 2294–2303 RSC.
- M. Zhang, Q. Luo, C. Sheng, D. Cao, X. Chen and H. Shu, Space-confined growth of large-mismatch CsPb(BrxCI1−x)3/GaN heterostructures with tunable band alignments and optical properties, Inorg. Chem. Front., 2022, 9(18), 4661–4670 RSC.
- P. E. Blöchl, Projector augmented-wave method, Phys. Rev. B: Condens. Matter Mater. Phys., 1994, 50(24), 17953–17979 CrossRef PubMed.
- G. Kresse and D. Joubert, From ultrasoft pseudopotentials to the projector augmented-wave method, Phys. Rev. B: Condens. Matter Mater. Phys., 1999, 59(3), 1758–1775 CrossRef CAS.
- G. Kresse and J. Furthmüller, Efficiency of ab initio total energy calculations for metals and semiconductors using a plane-wave basis set, Comput. Mater. Sci., 1996, 6(1), 15–50 CrossRef CAS.
- G. Kresse and J. Furthmüller, Efficient iterative schemes for ab initio total-energy calculations using a plane-wave basis set, Phys. Rev. B: Condens. Matter Mater. Phys., 1996, 54(16), 11169–11186 CrossRef CAS PubMed.
- J. P. Perdew, K. Burke and M. Ernzerhof, Generalized gradient approximation made simple, Phys. Rev. Lett., 1996, 77(18), 3865–3868 CrossRef CAS PubMed.
- M. Methfessel and A. T. Paxton, High-precision sampling for Brillouin-zone integration in metals, Phys. Rev. B: Condens. Matter Mater. Phys., 1989, 40(6), 3616–3621 CrossRef CAS PubMed.
- H. J. Monkhorst and J. D. Pack, Special points for Brillouin-zone integrations, Phys. Rev. B: Solid State, 1976, 13(12), 5188–5192 CrossRef.
- S. Grimme, Semiempirical GGA-type density functional constructed with a long-range dispersion correction, J. Comput. Chem., 2006, 27(15), 1787–1799 CrossRef CAS PubMed.
- K. Momma and F. Izumi, VESTA 3 for three-dimensional visualization of crystal, volumetric and morphology data, J. Appl. Crystallogr., 2011, 44(6), 1272–1276 CrossRef CAS.
- S. P. Culver, M. J. Greaney, A. Tinoco and R. L. Brutchey, Low-temperature synthesis of homogeneous solid solutions of scheelite-structured Ca1−xSrxWO4 and Sr1−xBaxWO4 nanocrystals, Dalton Trans., 2015, 44(33), 15042–15048 RSC.
- Y. Xie, B. Peng, I. Bravić, Y. Yu, Y. Dong, R. Liang, Q. Ou, B. Monserrat and S. Zhang, Highly efficient blue-emitting CsPbBr3 perovskite nanocrystals through neodymium doping, Adv. Sci., 2020, 7(20), 2001698 CrossRef CAS PubMed.
- S. Shahrokhi, M. Dubajic, Z.-Z. Dai, S. Bhattacharyya, R. A. Mole, K. C. Rule, M. Bhadbhade, R. Tian, N. Mussakhanuly, X. Guan, Y. Yin, M. P. Nielsen, L. Hu, C.-H. Lin, S. L. Y. Chang, D. Wang, I. V. Kabakova, G. Conibeer, S. Bremner, X.-G. Li, C. Cazorla and T. Wu, Anomalous structural evolution and glassy lattice in mixed-halide hybrid perovskites, Small, 2022, 18(21), 2200847 CrossRef CAS PubMed.
- J. E. Castellanos-Águila, L. Lodeiro, E. Menéndez-Proupin, A. L. Montero-Alejo, P. Palacios, J. C. Conesa and P. Wahnón, Atomic-scale model and electronic structure of Cu2O/CH3NH3PbI3 interfaces in perovskite solar cells, ACS Appl. Mater. Interfaces, 2020, 12(40), 44648–44657 CrossRef PubMed.
- A. Pecoraro, A. De Maria, P. Delli Veneri, M. Pavone and A. B. Muñoz-García, Interfacial electronic features in methyl-ammonium lead iodide and p-type oxide heterostructures: new insights for inverted perovskite solar cells, Phys. Chem. Chem. Phys., 2020, 22(48), 28401–28413 RSC.
- H. Zhang, J. Wang, W. Huang, L. Wang and Z. Lu, Interface bonding and failure mechanism of Ti(001)/Si(001) and TiO2(001)/Si(001) interfaces: A first-principles study, Surf. Interfaces, 2022, 30, 101833 CrossRef CAS.
- M. W. Stoltzfus, P. M. Woodward, R. Seshadri, J.-H. Klepeis and B. Bursten, Structure and bonding in SnWO4, PbWO4, and BiVO4: Lone pairs vs inert pairs, Inorg. Chem., 2007, 46(10), 3839–3850 CrossRef CAS PubMed.
- X. Gong, O. Voznyy, A. Jain, W. Liu, R. Sabatini, Z. Piontkowski, G. Walters, G. Bappi, S. Nokhrin, O. Bushuyev, M. Yuan, R. Comin, D. McCamant, S. O. Kelley and E. H. Sargent, Electron–phonon interaction in efficient perovskite blue emitters, Nat. Mater., 2018, 17(6), 550–556 CrossRef CAS PubMed.
- C. Quarti, F. De Angelis and D. Beljonne, Influence of surface termination on the energy level alignment at the CH3NH3PbI3 perovskite/C60 interface, Chem. Mater., 2017, 29(3), 958–968 CrossRef CAS.
- B. Du, Q. Wei, Y. Cai, T. Liu, B. Wu, Y. Li, Y. Chen, Y. Xia, G. Xing and W. Huang, Crystal face dependent charge carrier extraction in TiO2/perovskite heterojunctions, Nano Energy, 2020, 67, 104227 CrossRef CAS.
- Y. Guo, Y. Xue and L. Xu, Interfacial interactions and enhanced optoelectronic properties of GaN/perovskite heterostructures: Insight from first-principles calculations, J. Mater. Sci., 2021, 56(19), 11352–11363 CrossRef CAS.
- A. Giampietri, G. Drera and L. Sangaletti, Band alignment at heteroepitaxial perovskite oxide interfaces. Experiments, methods, and perspectives, Adv. Mater. Interfaces, 2017, 4(11), 1700144 CrossRef.
- D. Q. Fang and S. L. Zhang, Theoretical prediction of the band offsets at the ZnO/anatase TiO2 and GaN/ZnO heterojunctions using the self-consistent ab initio DFT/GGA-1/2 method, J. Chem. Phys., 2016, 144(1), 014704 CrossRef CAS PubMed.
- M. Tyagi, S. G. Singh, A. K. Chauhan and S. C. Gadkari, First principles calculation of optical properties of BaWO4: A study by full potential method, Phys. B, 2010, 405(21), 4530–4535 CrossRef CAS.
- R. Lindblad, D. Bi, B.-w. Park, J. Oscarsson, M. Gorgoi, H. Siegbahn, M. Odelius, E. M. J. Johansson and H. Rensmo, Electronic Structure of TiO2/CH3NH3PbI3 perovskite solar cell interfaces, J. Phys. Chem. Lett., 2014, 5(4), 648–653 CrossRef CAS PubMed.
- M. Cao, Y. Damji, C. Zhang, L. Wu, Q. Zhong, P. Li, D. Yang, Y. Xu and Q. Zhang, Low-dimensional-networked cesium lead halide perovskites: properties, fabrication, and applications, Small Methods, 2020, 4(12), 2000303 CrossRef CAS.
- P. Yadav, P. Dev Bhuyan, S. K. Rout, Y. Sonvane, S. K. Gupta and E. Sinha, Correlation between experimental and theoretical study of scheelite and wolframite-type tungstates, Mater. Today Commun., 2020, 25, 101417 CrossRef CAS.
- G. Ghosh, B. Jana, S. Sain, A. Ghosh and A. Patra, Influence of shape on the carrier relaxation dynamics of CsPbBr3 perovskite nanocrystals, Phys. Chem. Chem. Phys., 2019, 21(35), 19318–19326 RSC.
- S. Thawarkar, P. J. S. Rana, R. Narayan and S. P. Singh, Ni-doped CsPbBr3 perovskite: Synthesis of highly stable nanocubes, Langmuir, 2019, 35(52), 17150–17155 CrossRef CAS PubMed.
- D. Rangappa, T. Fujiwara and M. Yoshimura, Synthesis of highly crystallized BaWO4 film by chemical reaction method at room temperature, Solid State Sci., 2006, 8(9), 1074–1078 CrossRef CAS.
- E. Hannachi, Y. Slimani, M. I. Sayyed and K. G. Mahmoud, Scheelite-type BaWO4 doped with Ho2O3 oxide as a promising lead-free shield for gamma rays: Structural, optical properties, and radiation attenuation efficiency, Mater. Sci. Semicond. Process., 2023, 167, 107802 CrossRef CAS.
- M. I. A. Abdel Maksoud, M. A. Youssef and H. S. Hassan, Evaluation of barium tungstate nanocrystals for the sorption of radioactive cobalt and europium from aqueous solutions, Sci. Rep., 2023, 13(1), 21570 CrossRef CAS PubMed.
- L. M. Wheeler, E. M. Sanehira, A. R. Marshall, P. Schulz, M. Suri, N. C. Anderson, J. A. Christians, D. Nordlund, D. Sokaras, T. Kroll, S. P. Harvey, J. J. Berry, L. Y. Lin and J. M. Luther, Targeted ligand-exchange chemistry on cesium lead halide perovskite quantum dots for high-efficiency photovoltaics, J. Am. Chem. Soc., 2018, 140(33), 10504–10513 CrossRef CAS PubMed.
- H. Yang, W. Yin, W. Dong, L. Gao, C.-H. Tan, W. Li, X. Zhang and J. Zhang, Enhancing the light-emitting performance and stability in CsPbBr3 perovskite quantum dots via simultaneous doping and surface passivation, J. Mater. Chem. C, 2020, 8(41), 14439–14445 RSC.
- Y. Mao and S. S. Wong, General, room-temperature method for the synthesis of isolated as well as arrays of single-crystalline ABO4-type nanorods, J. Am. Chem. Soc., 2004, 126(46), 15245–15252 CrossRef CAS PubMed.
|
This journal is © the Partner Organisations 2024 |
Click here to see how this site uses Cookies. View our privacy policy here.