DOI:
10.1039/D4QI01449A
(Review Article)
Inorg. Chem. Front., 2024,
11, 6794-6852
Recent advances in rational design, synthesis and application of metal–organic frameworks as visible-light-driven photocatalysts
Received
10th June 2024
, Accepted 26th August 2024
First published on 29th August 2024
Abstract
The development of green renewable energy sources such as solar energy has become a focal point of research in addressing energy shortages and environmental pollution. Metal–organic frameworks (MOFs) as a novel photocatalytic material have garnered widespread attention due to their highly ordered porous structure and large surface area. This paper comprehensively reviews the advancements in MOF photocatalysts under visible light over the past five years; it provides concepts and directions for readers entering the field of MOF photocatalysis, covering construction, optimization and application. It specifically focuses on applications, including organic transformations, pollutant degradation, CO2 reduction, N2 fixation, and H2 production. Finally, the future development prospects are also discussed to meet the requirements for using MOF photocatalysis as a low-cost, stable technology suitable for practical applications.
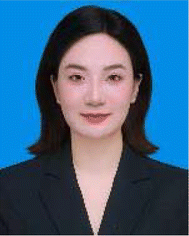 Xu-Sheng Li | Xu-Sheng Li was born in Shannxi Province, China, in 1999. She received her B.S. degree in 2021 from Xi'an Technological University, China. She is now pursuing her M.D. degree under the supervision of Prof. Ping Liu (Northwest University, China). Her research interests focus on the design and preparation of photocatalytic MOFs. |
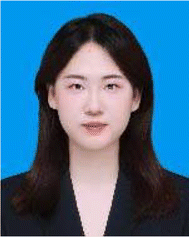 Yu-Jie He | Yu-Jie He was born in Gansu Province, China, in 2000. She received her B.S. degree in 2022 from Zhengzhou University, China. She is now pursuing her M.D. degree under the supervision of Prof. Ping Liu (Northwest University, China). Her research interests focus on photocatalysis functional MOFs, and MOFs for photocatalytic organic reactions. |
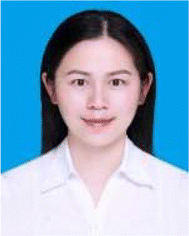 Jiao Chen | Jiao Chen obtained her B.S. degree (2016) in Applied Chemistry from Southwest University, and she received her Ph.D. (2021) in Organic Chemistry from Northwest University under the supervision of Prof. Jianli Li and Prof. Shengyong Zhang. She currently performs postdoctoral research in the College of Life Sciences at Northwest University. Her research interests focus on developing near-infrared fluorescent sensors for biomedical sensing, diagnosis, and treatment. |
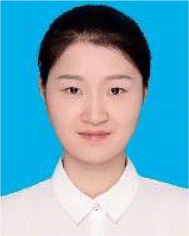 Quan-Quan Li | Dr Quan-Quan Li was born in Jiangsu, China, in 1990. She received her B.E. degree in 2013 from the Yancheng Institute of Technology and then her Ph.D. degree from Northwest University, China. In 2019, she joined the group of Prof. Jin-Xi Song (Northwest University, China) as a postdoctoral researcher, and now she is a lecturer at Yulin University. Her research interest focuses on the design and synthesis of photocatalytic MOFs and their functional applications. |
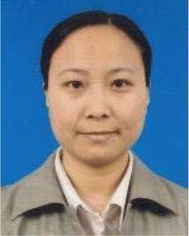 Ping Liu | Prof. Ping Liu was born in Shandong Province, China. She obtained her Ph.D. degree in 2005 from Northwest University, China (Supervisor: Yao-Yu Wang). She joined the group of Prof. Zhensen Wu at Xidian University as a post-doctoral researcher (2006–2010), and in 2011, she was a visiting scholar at the University of Strasbourg, France. Now she is a full professor at Northwest University. Her research interest focuses on the rational design and synthesis of coordination polymers and their functional applications. |
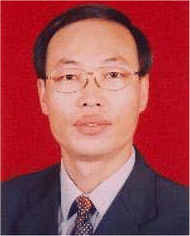 Jian-Li Li | Jianli Li studied chemistry at Northwest University and completed his Ph.D. (2007) in Organic Chemistry under the supervision of Prof. Zhen Shi. Then he did postdoctoral research at Northwest University in Prof. Guifang Zhao's group. He is currently working as a Full Professor at the College of Chemistry & Materials Science, Northwest University. His research expertise covers a broad spectrum of different fields of synthetic chemistry, including the design and development of photofunctional materials for biological sensing and theranostics, exploration of new synthetic methods for biomedical molecules, design and application of new catalytic materials, and photocatalytic energy and resource chemistry. |
1. Introduction
According to estimates by the International Energy Agency (IEA), the demand for fossil fuels has accounted for 81% of the world's total energy demand over the past few decades. Fossil fuels are non-renewable energy sources, and their combustion releases large amounts of CO2, CO, and SO2.1 To alleviate the energy shortages and environmental pollution caused by high demand, developing green energy with a sustainable development concept has become a research hotspot. Solar energy, as a green and renewable energy source, offers new solutions to existing energy and environmental problems. However, the key challenge is how to efficiently and rationally utilize solar energy to convert it into energy required by humans under mild conditions.2–4
Inspired by natural photosynthesis, in 1972, Fujishima et al. used single-crystal n-type TiO2 (rutile) semiconductor electrodes to achieve the photolysis of water under UV irradiation, making the first breakthrough in the conversion of light energy to chemical energy.5,6 TiO2 can only absorb ultraviolet light, which constitutes about 7% of the total solar radiation, thus limiting the photocatalytic efficiency, due to its narrow absorption spectrum. In recent years, traditional visible light photocatalysts such as g-C3N4, CdS and Fe2O3 have been extensively developed and applied. To overcome the limitations of traditional materials and combine the advantages of existing materials, it is crucial to develop visible-light photocatalysts that have a broad light-response range.7–19
In 2012, Li et al. first reported the photocatalytic reduction of CO2 under visible light using NH2-MIL-125(Ti).23,24 Since then, MOF-based visible-light-driven photocatalysts have flourished.25 MOFs are a class of porous complexes, typically formed by metal nodes (such as ions or clusters) linked with organic ligands (Fig. 1), and characterized by highly ordered porous structures and large surface areas, offering abundant active and adsorption sites. Their inherent narrow band gaps and high stability have sparked significant research interest.20–22
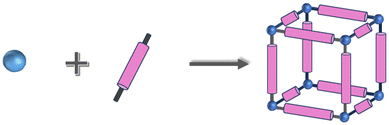 |
| Fig. 1 The MOF structure is created through the bonding of metal ions as nodes and organic molecules as linkers. | |
However, due to the poor light absorption capacity and the low efficiency with electron–hole separation of traditional MOFs, their practical application is limited. Therefore, some effective methods to improve performance have been proposed, including the modification of metal sites, functionalization of ligands, addition of various species (such as dyes and metal complexes) and construction of heterojunctions.26–29 The number of publications on MOF photocatalysis has rapidly increased over the past five years, reaching over a thousand by 2023 (Fig. 2). Notable advances have been made in several fields, including organic transformations, H2 production, pollutant degradation, CO2 reduction and N2 fixation.30–34
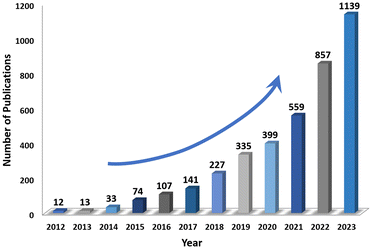 |
| Fig. 2 Publications trends of metal–organic framework (MOF) based photocatalysts. Publication results were obtained from Web of Science with the topic of “metal organic framework” and “photocatalysis” by the end of December 2023. | |
Although numerous reviews on MOF photocatalysis have been summarized, most of them focused on a single or a narrow application scope. To address this challenge this review provides a comprehensive summary and systematic outlook on the advances of MOF photocatalysts under visible light over the past five years. It offers ideas and directions for readers entering the field of MOF photocatalysis, from “construction–modification–application”. The article is structured in two parts: (i) performance optimization of MOF photocatalysts including the modification of metal sites, functionalization of organic linkers, addition of photosensitizers, construction of heterojunctions, encapsulation of functional guest species, morphology regulation and defect engineering; and (ii) focusing on the MOF photocatalysts in organic transformations, CO2 reduction, N2 fixation, pollutant degradation and hydrogen production. This not only gives guidance for the logical creation and modification of MOF photocatalysis but also provides fresh perspectives on their application.
2. Modulation strategies for the performance of MOF photocatalysts
In the heterogeneous photocatalytic system, the catalyst and reactants exist in different phases, facilitating easy recovery and reuse, showcasing advantages in industrial applications. MOFs possess atomic precision, customizable structures and unique physicochemical properties, rendering them highly promising in the field of photocatalysis.35 MOFs are constituted by metal ions/metal clusters in conjunction with organic ligands, linked via moderately strong coordination bonds that connect intramolecular pore structures. Polydentate organic ligands often consist of oxygen or nitrogen.36 The inorganic metal clusters are generally composed of transition metal ions, including common divalent ions such as Ni2+, and Zn2+, trivalent ions such as Fe3+, Sc3+, Co3+, Cr3+, and V3+, and group trivalent ions such as Al3+, In3+ and certain rare earth metal ions. The first MOF was synthesized and named by Yaghi et al. in 1995. Since then, MOFs have evolved into a broader family, currently encompassing notable subclasses such as MIL, UiO, ZIF, and PCN.37,38 In recent years, MOFs such as UiO-66, MIL-100, MIL-88A, MIL-53 and MIL-101 have been investigated as photocatalysts, exhibiting semiconductor-like properties with the capability to absorb light upon irradiation.39,40 In 2007, Horcajada et al. first synthesized MIL-100 by Fe–O octahedral clusters and benzene-1,3,5-tricarboxylic acid.41 More recently, in 2024, Li et al. successfully synthesized a novel CS/MIL-(Fe) photocatalyst (CS = charcoal sponge), which possessed a large surface area, excellent mechanical properties, and significantly enhanced photocatalytic performance.42 MIL-101 is also one of the representative MOFs responsive to visible light, a characteristic ascribed to the spin-allowed d-d transition of Cr3+.
However, issues such as the rapid recombination of photoinduced electron–hole pairs in traditional MOF heterogeneous catalysts can lead to low photocatalytic efficiency. To further enhance the efficiency and expand the application scope, understanding the specific processes of photocatalysis is beneficial and may aid in addressing the aforementioned issues.
The photocatalytic mechanism included a sequence of consecutive steps, including at least: (i) the absorption of light, (ii) the generation of photogenerated electron–hole pairs occurs at the site where the energy of the photon is absorbed, (iii) the photogenerated electron–hole pairs’ migration or recombination, (iv) on the surface, the photocatalyst adsorption of reactants, (v) occurrence of spatially separated redox reactions by the photogenerated electron–hole pairs on the active sites of the photocatalyst, and (vi) product desorption.43–51 It is worth noting that molecular oxygen O2 could be activated by the holes and electrons of photocatalysts, resulting in the production of various reactive oxygen species (ROS), e.g. superoxide anion radical (˙O2−), hydrogen peroxide (H2O2), singlet oxygen (1O2), and hydroxyl radical (˙OH). These generated ROS can further act as important intermediates for advanced oxidation.52 In principle, by controlling the band gap to increase light absorption and reduce the recombination rate of photogenerated carriers, the photocatalytic activity of catalysts can be enhanced. The required functionalities can be introduced through direct synthesis or post-synthetic modification (PSM) strategies.23,53,54
Recently, various effective methods have been developed to enhance the photocatalytic activity of MOFs under visible light exposure. This section will outline the commonly used and effective methods to improve photocatalytic performance in recent years, including modification of metal sites, functionalization of ligands, addition of photosensitizers, control of the heterostructure construction, encapsulation with functional guest species, morphology regulation and defect engineering.
2.1. Modification of metal sites
The choice of metal nodes is crucial in the development of MOFs customized for visible-light-driven applications. In MOFs, metal nodes function in performance like single semiconductor quantum dots. The charge separation state is generated during irradiation for photocatalysis. Consequently, choosing different metal nodes can modify the activity of MOF-based photocatalysts. These metal ions play a role in adjusting the electronic structure of MOFs, influencing parameters such as, for instance, bandgap, conductivity, and band position, and even promoting the separation of charges between excited-state ligands and metal ions, in addition to extending the lifetime of charge carriers.55 Additionally, modifying the local structure of MOF base materials is effective in optimizing the charge transfer process. The incorporation of metal ions into this structural optimization strategy also results in metal-to-metal cluster charge transfer (MCCT), enhancing spatial electron–hole separation and simultaneously suppressing charge recombination. This also creates additional active sites.56,57
Zhang et al. synthesized four homostructural porphyrin MOFs, namely UNLPF-10a, UNLPF-10b, UNLPF-11 and UNLPF-12, composed of free base, InIII-, SnIVCl2- and SnIV-porphyrins; the structure of the porphyrin ligand is shown in Fig. 3.58 Among these, UNLPF-12 demonstrated outstanding photostability and highly efficient photocatalytic activity in several significant organic transformations. The metalation of high-valent metal cations such as InIII and SnIV significantly transforms the electronic configuration of porphyrin macrocycles (Fig. 4A). This process resulted in the creation of a highly oxidized photoexcited state, which can undergo effective reductive quenching processes. These processes effectively enabled a range of organic reactions, encompassing aerobic hydroxylation of arylboronic acids, amine coupling reactions and Mannich reactions (Fig. 4B).
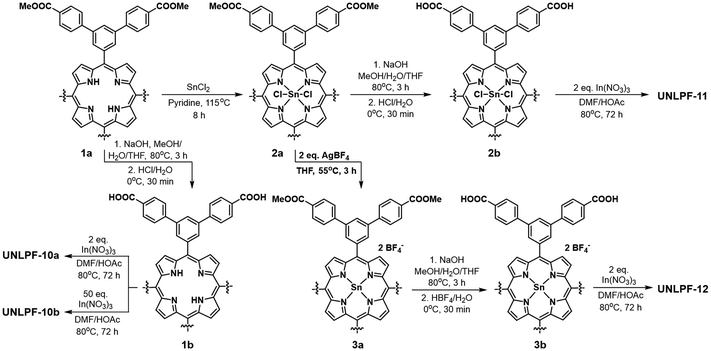 |
| Fig. 3 Synthesis of porphyrinic MOFs from ref. 58. Copyright 2015, American Chemical Society. | |
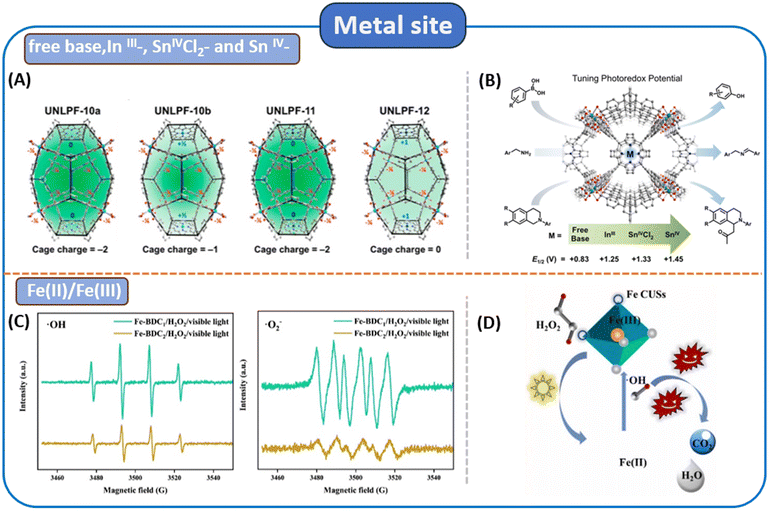 |
| Fig. 4 (A and B) Controlling the charge density of UNLPF-10a, -10b, -11, and -12 via the metalation of porphyrins; charges are shown with respect to cage occupancy in the overall framework. Schematic representation of four isomorphous porphyrin-based MOFs catalyzing a series of organic transformations from ref. 58. Copyright 2015, American Chemical Society. (C and D) Under visible light, DMPO was used to detect the EPR signals of ˙OH and ˙O2− generated by the trapping agent and the possible degradation mechanism from ref. 60. Copyright 2022 Elsevier Ltd. | |
Iron, an abundant element on Earth, possesses some degree of visible light absorption capability.59 Yang et al. synthesized Fe-BDC1 (ligand = terephthalic acid (H2BDC)) through a straightforward stirring method;60 compared with Fe-BDC2 synthesized by the solvothermal method, the adsorption capacity for RhB (rhodamine B) increased by approximately 5.85 times. In Fig. 4C, the characteristic peak signal of Fe-BDC1 can be clearly observed, while the peak intensity of Fe-BDC2 is negligible. The improved catalytic activity of Fe-BDC1 is mainly attributed to the expansion of its specific surface area (SSA) and the promotion of efficient electron separation and transfer processes, thereby accelerating the
Fe(II)/
Fe(III) cycle and increasing the yield of ROSs. The possible degradation mechanism was proposed as follows (Fig. 4D): firstly, pollutants were adsorbed on the surface or within the pores of the MOF. Due to Lewis acid–base interactions, H2O2 rapidly coordinated with Fe CUSs. Meanwhile, under visible light irradiation, electrons and holes were effectively separated, with the separated electrons migrating along the Fe–O bond, leading to the reduction of
Fe(III). Subsequently, internal electron transfer between
Fe(III) and the coordinated H2O2 initiated the Fenton reaction, generating ROSs. Thereafter, the adsorbed organic pollutants were primarily degraded rapidly by ˙OH.
For instance, the incorporation of high-valent cations can also enhance photocatalytic performance. For example, Liu et al. introduced Ti4+ into Co(II) clusters, to serve as heterometallic nodes, which significantly enhanced the stability and high activities for the photocatalytic water oxidation of Co-MOF photocatalysts.61 A stepwise synthetic strategy was employed to first synthesize a trinuclear [Co2Ti(μ3-O)(COO)6] metal cluster as shown in Fig. 5A followed by assembling of the cluster with visible light-responsive ligands (ligand = 3,3′,5,5′-azobenzenetetracarboxylic acid (ABTC)) into MOFs through a solvothermal process. This resulted in a novel single-site bimetallic Co-MOF (namely PFC-20-Co2Ti) (PFC = porous materials from FJIRSM, CAS); the constructed PFC-20-Co2Ti is isomorphic to PCN-250, serving as an efficient and reusable photocatalyst for the water oxidation of O2. The incorporation of single-site Ti(IV) sites at the nodes modulated the charge distribution, and enhanced the valence state of the bimetallic nodes, endowing MOFs with strong coordination ability and improved catalytic kinetics. This step-by-step synthesis approach was also applicable to other transition metals (such as Mn and Ni) for preparing bimetallic MOF catalysts with water stability and activity. Yang et al. installed high-valent W6+ ions onto the Ti-oxo clusters of MIL-125(Ti) (ligand = H2BDC).62 Without disturbing the crystal structure formation of the MOF substrate, the holes attracted by high-valence W6+ ions combine with the Ti-oxo clusters, serving as electron donors in the catalytic system. The resulting structure facilitated the metal-to-cluster charge transfer (MCCT), achieving a spatial separation of electrons and holes, further enhancing light absorption, suppressing charge recombination, promoting interface reactions and lengthening the lifetime of the photogenerated charge carriers. Improved H2 production performance was seen. Jiang et al. installed Fe3+ onto Zr-oxo clusters, to synthesize a representative Fe-UIO-66 (ligand = H2BDC) by a microwave-assisted approach.63 The introduction of Fe3+ activated the “inert” MOF for photocatalytic C–H activation. Pan et al. selected ZIF-8 as an ideal model for metal ion doping which was cheap and was synthesized easily.64 Co2+, Ni2+, or Cu2+ as photoreactive metal ions were added into the ZIF-8 metal nodes, resulting in a suite of MxZny-ZIF (ZIF = ZIF-8, M = Co, Ni, Cu, ligand = 2-methylimidazole (2-MeIM)) (Fig. 5B). With Co(II) doping of the metal ion sites, the type of photogenerated ROS transitioned from the initial singlet 1O2 to multiple ROS like 1O2, ˙O2−, which can play an important role in the field of photocatalysis.
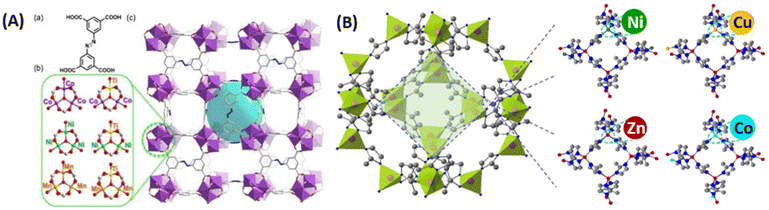 |
| Fig. 5 (A) The crystal structure of PFC-20 from ref. 61. Copyright 2021, Chinese Chemical Society. (B) Schematic illustration of the crystal structures of ZIF-8 and M/Zn-ZIFs (M = Co, Ni, Cu) from ref. 64. Copyright 2022, Elsevier Ltd. | |
Compared with monometallic catalysts, the introduction of a second metal alters the interfacial electronic structure and charge distribution. Jiang et al. combined Cu and Ni DMSPs (dual-metal-site pairs) in their respective single-site forms into an MOF to obtain MOF-808-CuNi (ligand = 1,3,5-benzenetricarboxylic acid (H3BTC)) in a flexible microenvironment. The resulting MOF-808-CuNi exhibited a high CH4 yield (158.7 μmol g−1 h−1).65
Zhong and Wang et al. made a series of isostructural CoxNiy-MOFs based on 4,5-dicarboxylic acid (H3IDC) and 4,4′-bpy, which were synthesized for photocatalytic CO2 reduction.66 X-ray diffraction (XRD) patterns demonstrated that mixed MOFs maintained similar structures to that of monometallic MOF (Fig. 6A). In Fig. 6B, comparing the energy-band of Co-MOF, CoxNiy-MOFs and Ni-MOF, the conduction band potentials of CoxNiy-MOFs are more negative than the redox potential of photocatalytic CO2 reduction to CO (−0.52 V vs. NHE), which meets the thermodynamic requirement of CO2–CO conversion. Compared with monometallic catalysts, the addition of a second metal altered the surface electronic structure and charge distribution, thereby affecting the photocatalytic performance.
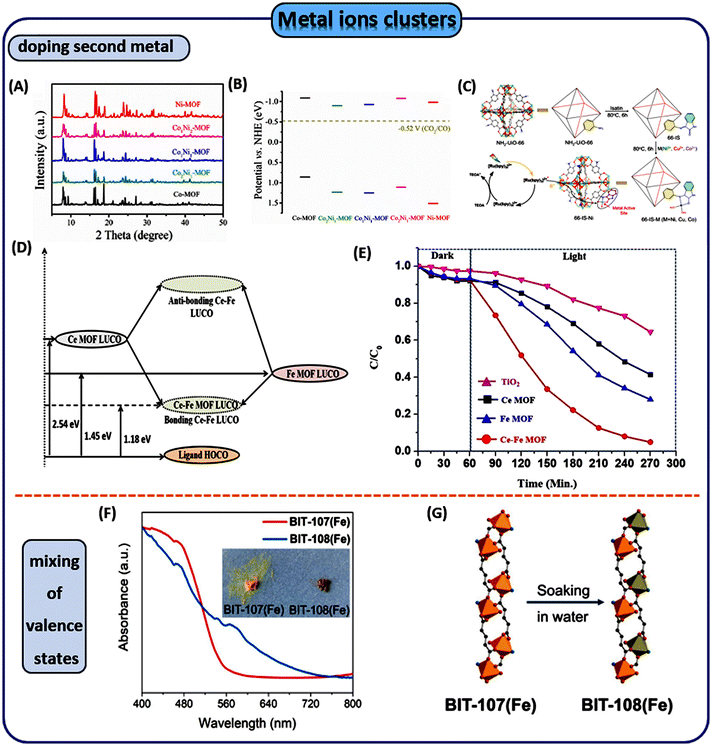 |
| Fig. 6 (A) XRD patterns and (B) the band structures of CoxNiy-MOFs from ref. 66. Copyright 2021, Elsevier B.V. (C) Schematic illustration of the preparation of 66-IS-M and its photocatalytic mechanism from ref. 67. Copyright 2023, American Chemical Society. (D and E) The emergence of a novel lowest unoccupied crystal orbital (LUCO) in a heterometallic Ce–Fe MOF leads to a reduced transition energy, in comparison with the homometallic Ce and Fe MOFs. Degradation of synthetic materials compared with TiO2 from ref. 68. Copyright 2020, John Wiley & Sons, Ltd. (F) UV–vis and picture inset. (G) Schematic diagram of valence change from ref. 69. Copyright 2021, Elsevier B.V. | |
Yang et al. prepared catalysts by anchoring metal ions (Co, Ni, Cu) in NH2-UiO-66 through the covalent coupling of isatin-Schiff basemetal complexes with microporous structures (Fig. 6C).67 During the reaction process, the anchored metal ions serve as active sites and exhibit high activity. Then, Pichiah Saravanan et al. synthesized a structurally stable 3d–4f heterometallic compound constructed with transition metal (Fe) and one lanthanide metal cluster (Ce) linked with a photon-harvesting organic ligand coordination polymer through a solvothermal approach.68 In comparison with homometallic Ce-MOF or Fe-MOF, the Ce–Fe MOF (ligand = 2-amino-1,4-benze-nedicarboxylic acid) exhibited exceptional light absorption capability. As shown in Fig. 6D, this enhanced activity is credited to the close intermingling of two distinct metal clusters in a heterometallic setting. Resulting in the creation of low-energy conduction orbitals, this process effectively decreased the transition energy. Therefore, the Ce–Fe MOF achieved a remarkable degradation rate of 94.6% for Acetaminophen (PCM), and the degradation of PCM is shown in Fig. 6E.
The coordination of heteroatoms and the mixing of valence states can also enhance transitions such as visible-light-driven MMCT. This effectively optimized the band structure and charge transfer dynamics of the metal clusters. Furthermore, the catalytic pathways may also be favorably modulated. Wang et al. synthesized an O,N co-ligated mixed valence state Fe(II/III) MOF named BIT-108 (ligand = 3′,5′-di(1H-1,2,4-triazol-1-yl)-[1,1′-biphenyl]-3,5-dicarboxylic acid (H2DTzBPhDC)) by partially oxidizing the ions of the Fe(II)2N2O10 clusters.69 Using the Fe(II/III) MOF as a catalyst and platinum as a co-catalyst, a 25-fold increase in the visible light-induced H2 production rate was achieved compared with the original Fe-MOF. The parent iron(II) framework BIT-107 (Fe) undergoes ageing in water to form high-spin iron(III), color from Fe(III) orange to green, resulting in mixed-valence iron nodes. BIT-108 (Fe) has an absorption spectrum spanning 400–700 nm and its excited state is capable of reducing protons in solution. Mechanistic studies indicated that the catalytic action is caused by the transfer of electrons from the sacrificial agent to the Fe(III) center at the nodes (Fig. 6F–G).
Optimization can also be achieved by adjusting the valence states of metal sites. Pang et al. used cellulose acetate (CA), a natural polymer, which is rich in electron-donating oxygen-containing groups.70 These groups such as hydroxyl and acetyl groups exhibit strong coordination capabilities. Integrating CA into the visible-light-responsive PCN-222 (ligand = (4-carboxyphenyl)-porphyrin (H2TTPP)) resulted in the formation of interface-optimized Zr-MOF composite materials via a simple one-pot reaction. In the synthesized composite material, the oxygen-containing groups in cellulose formed strong coordination interactions with the Zr4+ ions of Zr-MOFs. The oxygen atoms on the coordinating groups have a strong electron-donating capability, enabling them to transfer their electrons to the nearby Zr4+ ions at the interface of the composite material, resulting in a lower valence state (Zr3+) and a reduced bandgap, which is advantageous for photoelectron transfer.
2.2. Functionalization of organic linkers
While the regulation of metal sites plays a significant role in the catalytic process, precisely modulating the structure of the ligands can greatly influence the photocatalytic activity.71 When visible light irradiates MOFs, the organic linkers act as light-absorbing antennas. The variation of organic linkers within MOFs affects the efficiency of light absorption and charge transfer. Therefore, the functionalization of organic linkers by optimizing the LMCT (ligand-to-metal charge transfer) mechanism profoundly influences the stability of MOF frameworks, light collection capabilities and catalytic site performance.72 Introducing visible-light-responsive organic ligands such as pyridyl, porphyrin, and organic chromophores into the MOF can alter its ability to absorb visible light.73,74 Additionally, the optical properties of the MOF can be modified by introducing substituents with different electron-donating and electron-withdrawing abilities. The mixed linker strategy can effectively narrow the band gap and expand the light absorption range. Furthermore, selectively removing ligands from mixed-ligand MOFs through thermal decomposition to introduce hierarchical porosity is an effective method.75,76
Wang et al. introduced a novel MOF photocatalyst utilizing bipyridine (ligand = 9,10-bis(4′-pyridylethynyl)-anthracene (BPEA)) as a pillared ligand used in visible-light harvesting.77 This Zn-MOF, named NNU-36, was synthesized using a controlled pillar-layer method (Fig. 7A–C). Under visible light exposure, NNU-36 demonstrated remarkable efficiency in reducing and degrading Cr(VI) in an aqueous solution. The success of NNU-36 underscores the feasibility of incorporating ideal visible light-responsive moieties into MOFs, offering promising prospects for photocatalytic applications.
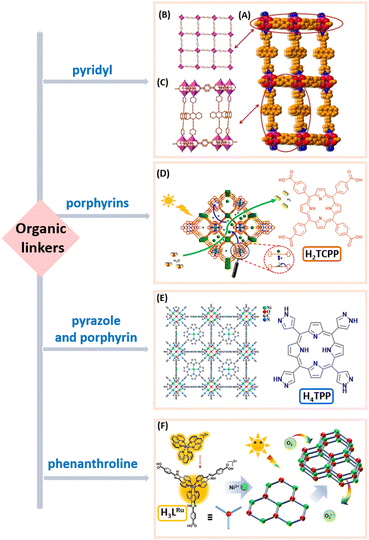 |
| Fig. 7 (A–C) Structure of NNU-36; the layer structure is composed of BPDC ligands and zinc dimers; the BPEA ligand binding to the Zn-N bonds in the layer structure to form NNU-36 from ref. 77. Copyright 2017, American Chemical Society. (D) Porphyrin MOF photocatalysis water decomposition to produce hydrogen from ref. 78. Copyright 2018, American Chemical Society. (E) Crystal structures and coordination spheres of PCN-601 from ref. 79. Copyright 2020, American Chemical Society. (F) Schematic illustration of LTG-NiRu from ref. 80. Copyright 2023, American Chemical Society. | |
As a crucial component in natural light energy conversion, porphyrins exhibit distinctive photophysical properties owing to their unique π-conjugated structure. Furthermore, MOFs constructed with porphyrin rings offer versatile tunability in optical and catalytic properties, driving extensive research interest in these materials.
In 2018, Jiang et al. presented a groundbreaking study on MOF photocatalysis leveraging the distinct behaviour of metal porphyrins.78 Employing In(OH)3 as a precursor, they synthesized USTC-8(In) (ligand = tetra(4-carboxyphenyl)porphyrin (H2TCPP)), a highly stable MOF based on out-of-plane (OOP) porphyrins, through the controlled release of metal ions. Under visible light irradiation, In(III) ions on the OOP porphyrin were reduced and easily detached, preventing back electron transfer and enhancing photocatalytic performance. In the structure, indium ions both form indium-oxygen carbonyl chains and metalate the porphyrin ring in situ, positioned above the porphyrin plane, providing a unique structure. USTC-8(In) demonstrated superior photocatalytic activity in H2 production compared with the structurally similar USTC-8 and USTC-8(0.2In), highlighting its high stability and unique porphyrin structure (Fig. 7D). Then, In 2020, Cao et al. synthesized a Ni-MOF named PCN-601 (ligand = 5,10,15,20-tetra(1H-pyrazol-4-yl)porphyrin (H4TPP)), incorporating pyrazole and porphyrin units, which combined light-harvesting capabilities, active catalytic sites and a substantial surface area.79 PCN-601 has been proved to be an outstanding and highly durable photocatalyst, attaining room-temperature, visible light-powered full CO2 reduction through water vapor (Fig. 7E). Kinetic studies indicated that the robust coordination provided by pyrazole groups and Ni-oxo clusters endowed PCN-601 with an optimal band alignment, which in turn promotes rapid electron transfer from the ligands to the nodes. PCN-601 exhibited CO2-to-CH4 generation rates surpassing those of similar carboxylic acid-based porphyrin MOFs and classical Pt/CdS photocatalysts by over 3 and 20 times, respectively.
Chen et al. ingeniously combined the intrinsic benefits of mesoporous MOFs with the [Ru(Phen)3]2+ photosensitizers in a heterometallic Ni(II)/Ru(II) mesoporous MOF (LTG-NiRu, ligand = H3LRu).80 This integration involved linking pre-modified metal ligands with nickel metal centers. LTG-NiRu boasted a sturdy framework and larger one-dimensional channels, allowing the [Ru(Phen)3]2+ to anchor onto the inner walls of the mesoporous MOF channels. This configuration effectively addressed the challenges of product/catalyst separation and catalyst recovery in multiphase systems. Notably, LTG-NiRu exhibited outstanding performance for aerobic oxygenation (Fig. 7F).
The modification of organic linkers (such as introducing new functional groups) can adjust the band gap in MOFs.81–83 Typically, electron-donating substituents induce a redshift in the light absorption of MOFs, thereby enhancing their photocatalytic activity. For instance, Husam N. Alshareef et al. utilized a straightforward stirring hydrothermal method to fabricate 1D titanium phosphonate MOF photocatalysts.84 The homogeneous incorporation of organic phosphine linkers reduced the bandgap. This is attributed to the remarkable electron-donating ability of the –OH functional group, which effectively shifts the top of the valence band, and then tunes light absorption to fall within the visible spectrum. Additionally, the unique one-dimensional nanowire topology enhanced the transport and separation of photoinduced charge carriers. Consequently, under visible and full-spectrum simulator irradiation, phosphonic acid titanium nanowires exhibited significantly enhanced photocatalytic hydrogen evolution activity. At the same time, Shang et al. achieved a groundbreaking advancement by functionalizing hierarchical porous ZIF-8 nanocrystals through a simple thermal treatment in air at temperatures as low as 200 °C.85 This transformation converted the ZIF-8 nanocrystals from conventional ultraviolet-driven photocatalysts to novel broad-spectrum-driven photocatalysts. The thermal treatment not only led to a significant increase in surface area but also broadened the light absorption range from ultraviolet to infrared. The formation of isocyanate groups (–N
C
O) in the thermally treated ZIF-8 (ZIF-8-T) was identified as the crucial factor enabling ZIF-8-T to exhibit outstanding photocatalytic performance in the degradation of formaldehyde.
Jiang et al. reported a titanium-based MOF, MIL-125, for its ability to perform dark photocatalytic hydrogen production.86 By introducing various functional groups onto the ligand, distinct MOFs were synthesized (MIL-125-X, X = NH2, NO2, Br), each exhibiting markedly different activities (Fig. 8A). The electron-donating or electron-withdrawing nature of these functional groups influenced the electron storage capacity and lifetime of Ti3+ intermediates under light exposure, resulting in varied dark photocatalytic activities among the MOFs with different functional groups. Among these, MIL-125-NH2 displayed superior activity compared with MOFs with other functional groups. Next, Wang et al. conducted a significant study where a range of MIL-101-X(Fe) MOFs (X = –H, –NH2, –OH, –OCH3) with different substituents was synthesized successfully.87 These MOFs were then subjected to adsorption-degradation experiments involving various antibiotics. The innovative approach involved altering the substituents within the side chains of MIL-101(Fe), resulting in changes in particle morphology (Fig. 8B) and active surface properties, thereby significantly enhancing their efficacy in removing stubborn pollutants. This research provided mechanistic insights into modulating Fenton-like reactions by manipulating substituents on the side chains of MOFs.
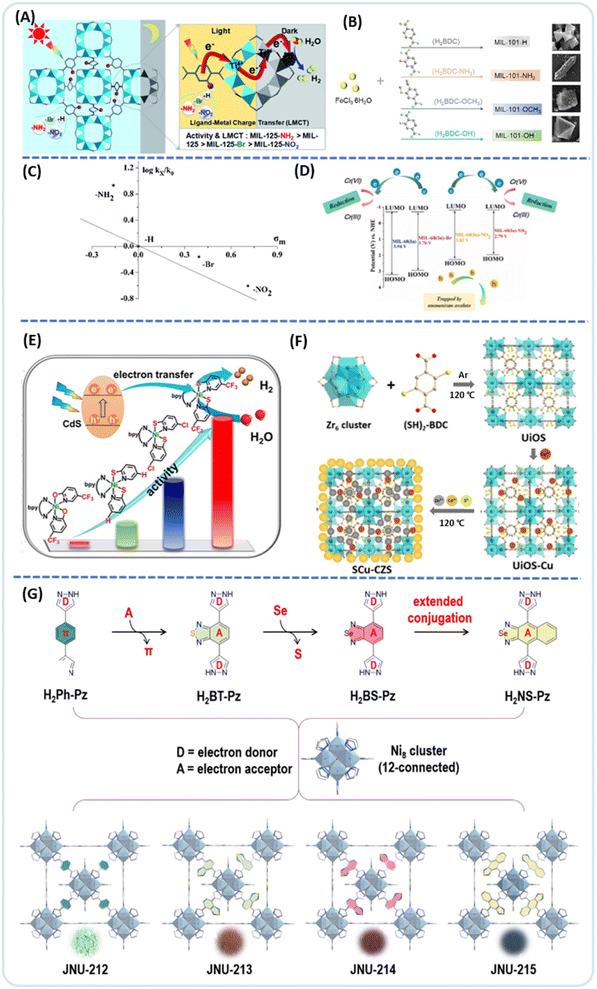 |
| Fig. 8 (A) Showing the dark photocatalysis over MIL-125 and MIL-125-X (X = NH2, NO2, Br) from ref. 86. Copyright 2022, Royal Society of Chemistry. (B) Schematic illustration of the synthesis of Fe-based MOFs from ref. 87. Copyright 2023, Elsevier B.V. (C and D) Hammett plot and possible mechanism of photocatalytic selective reduction of Cr(VI) to Cr(III) over MIL-68(In)-X from ref. 88. Copyright 2018, Elsevier B.V. (E) The synthesis of CdS/UiO–Ni–X–Y (X = S, O; Y = H, Cl, CF3) for photocatalytic H2 production from ref. 71. Copyright 2024, American Chemical Society. (F) Schematic illustration of the synthesis process of SCu-CZS from ref. 92. Copyright 2020, Elsevier B.V. (G) Schematic illustration of linker engineering in the synthesis of ultra-stable Ni8-MOFs from ref. 93. Copyright 2023, American Chemical Society. | |
Wu et al. selected a classic indium-based MOF MIL-68(In)-X for study due to its affordability, adjustability and stability.88 They synthesized MIL-68(In)-X (X = H, NH2, NO2, Br) in one step using a solvothermal method and organic linkers with different substituents corresponding to H2BDC (–H), H2BDC-NH2 (–NH2), H2BDC-NO2 (–NO2) and H2BDC-Br (–Br), to modulate electronic structure changes. The photoactivity of MIL-68(In)-X was influenced by the different substituents strongly and a Hammett-type linear free-energy relationship (LFER) was observed between the reaction rates and the electronic properties of the substituents. In other words, modifying MIL-68(In) with electron-donating substituents (like –NH2) can enhance the photoreductive performance of Cr(VI), while substituents that withdraw electrons (like –NO2, –Br) diminish its photoactivity (Fig. 8C and D). Jiang et al. prepared a series of sandwich-structured composites UiO-66-NH2@Pt@UiO-66-X (X = –H, –Br, –NA (naphthalene, in short), –OCH3, –Cl, –NO2).89 By varying the X groups in the UiO-66-X shell, they balanced the proton reduction capability of Pt and the charge separation of the UiO-66-NH2 core. UiO-66-NH2@Pt@UiO-66-H exhibited optimal photocatalytic H2 production activity. UiO-66 which were amino-functionalized can elevate charge separation or expand the light absorption of MOFs into a visible range.81–83,90
In addition to amino groups, thiol (–SH) groups also act as electron-donating groups, capable of effectively coordinating with metal centers (particularly noble metals), thereby creating additional unsaturated metal sites for catalysis. When thiol (–SH) groups are incorporated into the UiO-66, metal ions can be incorporated into the MOF's cavities through coordination bonds. Using thiol as a connector in UiO-66/Cd could effectively prevent CdS quantum dot aggregation, improving photocatalytic efficiency. Shi et al. initially created a UiO-66 MOF (UiO-66-(SH)2) using thiol as a ligand, then added CdS and ultimately formed the UiO-66-(S-CdS)2 photocatalyst.91 Well-dispersed CdS quantum dots are trapped in UiO-66-(SH)2 pores and the sulfur bonds linking UiO-66 and CdS act as an efficient charge transfer bridge, boosting interfacial carrier transfer and hydrogen production. In 2024, Jiang et al. decorated CdS nanoparticles (NPs, 27 ± 0.3 nm) on the surface of UiO-67,71 which is assembled from Zr-oxo clusters and 2,2′-bipyridine-5,5′-dicarboxylic acid, thus forming a CdS/UiO composite material. Subsequently, the chelated N sites on the MOF linker can install single Ni(II) sites, which are further six-coordinated by different molecules to obtain CdS/UiO–Ni–X–Y (X = S, O; Y = H, Cl, CF3), where X and Y act on the primary and secondary coordination spheres. The results indicated that the optimized CdS/UiO–Ni–S–CF3 composite material achieved a high H2 production rate (Fig. 8E). The increased in activity is due to the functional groups in the primary coordination atom and secondary coordination sphere, which critically regulate the charge separation kinetics and energy barriers of the rate-determining step (RDS); in particular, the primary coordination sphere has a more pronounced effect compared with the secondary coordination sphere.
Subsequently, Shi et al. loaded CdS/ZnS quantum dots onto Cu(II)-modified thiol-functionalized UiO-66 (UIOS-Cu) MOFs, as shown in Fig. 8F.92 CdS and ZnS quantum dots are evenly distributed within the MOF channels and on the surface, with Cu(II) ions attached to thiol groups. The HOMO of UIOS-Cu is elevated above the VB of CdS due to Cu(II) modification, promoting the efficient transfer of photoinduced holes from the VB of CdS to the HOMO of UIOS-Cu.
By adjusting the electron-accepting capacity in the D–A–D building blocks, the charge separation efficiency can be further enhanced. Li et al. reported a series of isostructural fcu-topology Ni8-MOFs using linearly bridged dipyrazole as the organic linker (named JNU-212, JNU-213, JNU-214 and JNU-215, JNU = Jinan University).93 As shown in Fig. 8G, by changing the units from benzene to benzothiadiazole, to benzoselenadiazole and to naphthoselenadiazole, their corresponding MOFs exhibited progressively improved photoelectrochemical performance. The benzoselenadiazole unit in JNU-214 exhibited the most compatible band gap and the highest ROS generation efficiency, making it a marked photocatalyst in the oxidative coupling reaction. Tuning the electron-accepting capability of pyrazole-bridging units, studies have demonstrated that through ligand engineering, the charge separation and transfer effectiveness of Ni8-MOFs can be significantly improved. Similarly, Li et al. reported a porphyrin-based donor–acceptor (D–A) MOF consisting of a meso-tetra(4-carboxyphenyl)porphyrin (TCPP) donor and a thieno [3,2b:20,30-d]thiophene-S,S-dioxide (BTDO) acceptor via coordination with Zn2+ ions and denoted as TCPP-Zn-BTDO.94 This combination enhanced the electron push–pull effect, and the formation of the donor–acceptor (D–A) structure effectively broadened the spectral absorption range, which facilitated the separation of photogenerated carriers, thus enhancing the photocatalytic performance.
Incorporating an electron-enriched skeleton into a single-ligand MOF is an effective strategy to optimize charge separation efficiency and improve photocatalytic performance.95–97
Naphthalenediimides (NDIs) are a class of chemically active, π-electron deficient compounds, widely used in the photocatalysis field.98–101 Jin et al. utilized a straightforward one-pot synthesis strategy to develop Zr-NDI-H2DPBP (ligand = 5,15-di(p-benzoato)porphyrin (H2DPBP)) as a heterogeneous photocatalyst for the aerobic oxidation coupling of anilines at room temperature (Fig. 9A).94 Its imine production rate greatly surpasses other non-noble metal MOF photocatalysts and it can be effectively used for many aniline derivatives. Experimental and DFT computational results show that in the mixed-ligand Zr-NDI-H2DPBP, the PET occurs between the donor H2DPBP and acceptor NDI ligands and, along with the sensitization of H2DPBP, significantly enhances the conversion of oxygen in the ground-state to active ˙O2− and 1O2, ultimately enhancing the photocatalytic activity of Zr-NDI-H2DPBP.
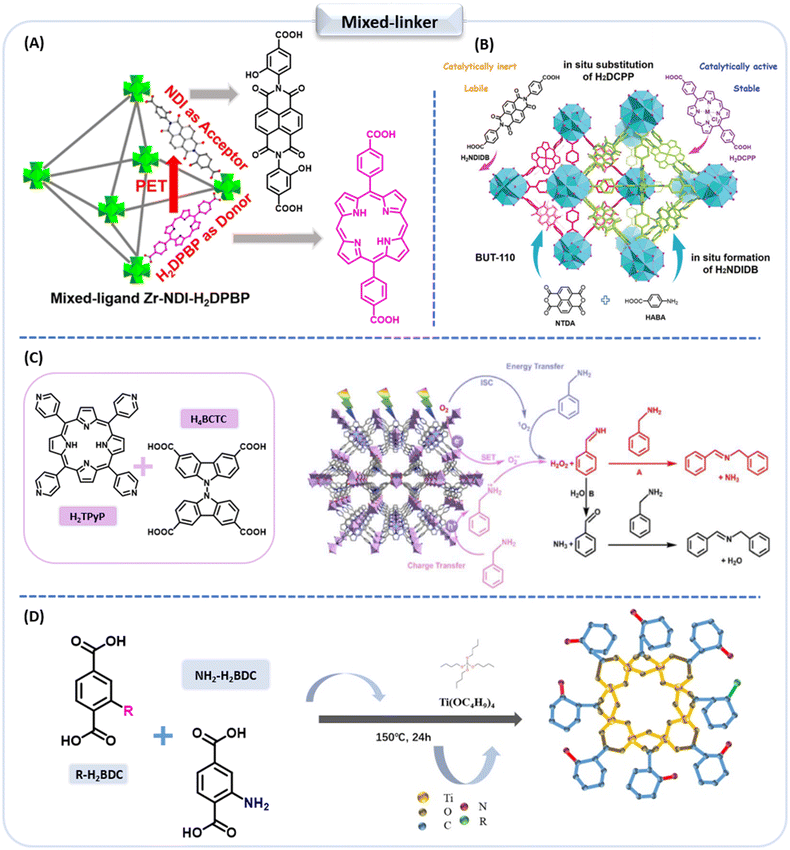 |
| Fig. 9 (A) The targeted PET model of mixed-ligand MOF Zr-NDI-H2DPBP from ref. 94. Copyright 2023, American Chemical Society. (B) In a Zr(IV)-MOF in situ porphyrin substitution from ref. 102. Copyright 2021, Wiley-VCH GmbH. (C) Proposed mechanisms for the photocatalytic oxidative benzylamine coupling reaction over CCNU-16 from ref. 105. Copyright 2022, Royal Society of Chemistry. (D) Schematic diagram for the synthetic process of NH2/R-MIL-125 (R = Cl, H, CH3) from ref. 106. Copyright 2022, American Chemical Society. | |
Researchers have also used ligand substitution strategies to boost the photo-catalytic efficacy of MOFs while stabilizing their structure. For instance, Li et al. proposed a strategy to enhance the stability and functionality of Zr(IV)-MOFs through in situ porphyrin substitution.102 A linear porphyrin ligand, 4,4′-(porphyrin-5,15-diyl)dibenzolate (DCPP2−), that is size and geometry matched, was chosen to replace the NDIDB2− (4,4′-(1,3,6,8-tetraoxobenzo[lmn][3,8] phenanthroline-2,7(1H,3H,6H,8H)-diyl) dibenzoate) ligand. This strategy enhanced the stability and functional linkage of BUT-109 (Zr), resulting in the BUT-110 series with varying DCPP2− content (Fig. 9B). The chemical stability of the BUT-110 series was greatly enhanced and the incorporation of metalloporphyrins endowed the BUT-110 MOF with exceptional photocatalytic activity for CO2 reduction without photosensitizers.
Carbazole, triphenylamine and porphyrin are typical multifunctional materials known for their efficient light absorption and excellent electron-donating properties.103,104 When loaded into MOF frameworks, carbazole ligands enhance charge transfer and photocatalytic activity. Wen et al. have synthesized a new MOF, Zn5(TPyP)(BCTC)2(H2O)3 (CCNU-16), from porphyrin- and carbazole-based organic linkers, which photocatalytically oxidized amines and sulfides in visible light (Fig. 9C).105 The TOF (turnover frequency) for benzylamine and thioanisole reached 121.0 h−1 and 190.7 h−1, respectively, Experimental results indicate that the incorporation of the H4BCTC ([9,9′-bicarbazole]-3,3′,6,6′-tetracarboxylic acid) ligand enhances CCNU-16 activity.
The construction of dual-ligand MOFs has mainly focused on the introduction of two linkers with excellent light absorption properties, and the rational design of metal sites and organic ligands is of great significance in promoting electron transfer in dual-ligand MOFs. Jiang et al. employed NH2-MIL-125(Ti) as a model system to enhance the photoinduced electron transfer between organic ligands and metal clusters using the dual-quantum-induced push–pull effect.106 Utilizing the Hammett constant (σm) as a guide, they synthesized a dual-ligand MOF NH2/Cl-MIL-125, with –NH2 (σm = −0.16) and –Cl (σm = 0.37) chosen as the electron-donating and electron-withdrawing group, respectively (Fig. 9D). Concurrently, –CH3 (σm = −0.07, electron-donating) and –H (σm = 0, neither electron-donating nor electron-withdrawing) were selected as reference groups to validate the electron push–pull mechanism. Under full-spectrum irradiation, NH2/Cl-MIL-125 exhibited higher photocatalytic hydrogen evolution activity compared with single-ligand NH2-MIL-125, NH2/CH3-MIL-125 and NH2/H-MIL-125. This improvement is likely due to the electron push–pull interaction between –NH2 and –Cl, which effectively facilitated electron transfer.
2.3. The addition of photosensitizers
The introduction of guest molecules is a powerful means to improve the photocatalytic performance of MOFs.107 The main interaction types usually include π–π stacking, coordination bonds, hydrogen bonds, halogen bonds, and electrostatic interactions with charged compounds. These interactions can significantly affect the host–guest interactions (Fig. 10).108
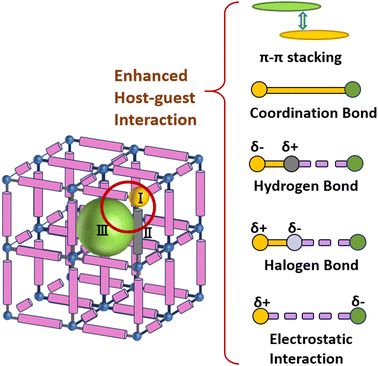 |
| Fig. 10 Three factors: the site of metal sites (I); modifications to the organic linker moiety (II); the introduction of dopants either during synthesis or post-synthesis (III); and the main interaction types between host–guest. | |
Using photosensitizers, such as organometallic complexes, represents another effective approach for crafting MOFs with visible light absorption capabilities. Zhang et al. pioneered the utilization of Ru(bpy)3Cl2 as a template and rigid tricarboxylate ligands to synthesize a battery of Ru(bpy)3@MOFs, wherein the [Ru(bpy)3]2+ fragments were encapsulated within sodalite-type cages.109 The open channels and polyhedral cages within the MOFs effectively distributed the encapsulated photocatalysts, enhancing the mobility of reactants and products, and thereby improving the catalytic activity. Ru(bpy)3@MOFs exhibited excellent catalytic performance under visible light in the aerobic oxidation of benzyl halides and the cyclization of tertiary anilines and maleimides (Fig. 11A).
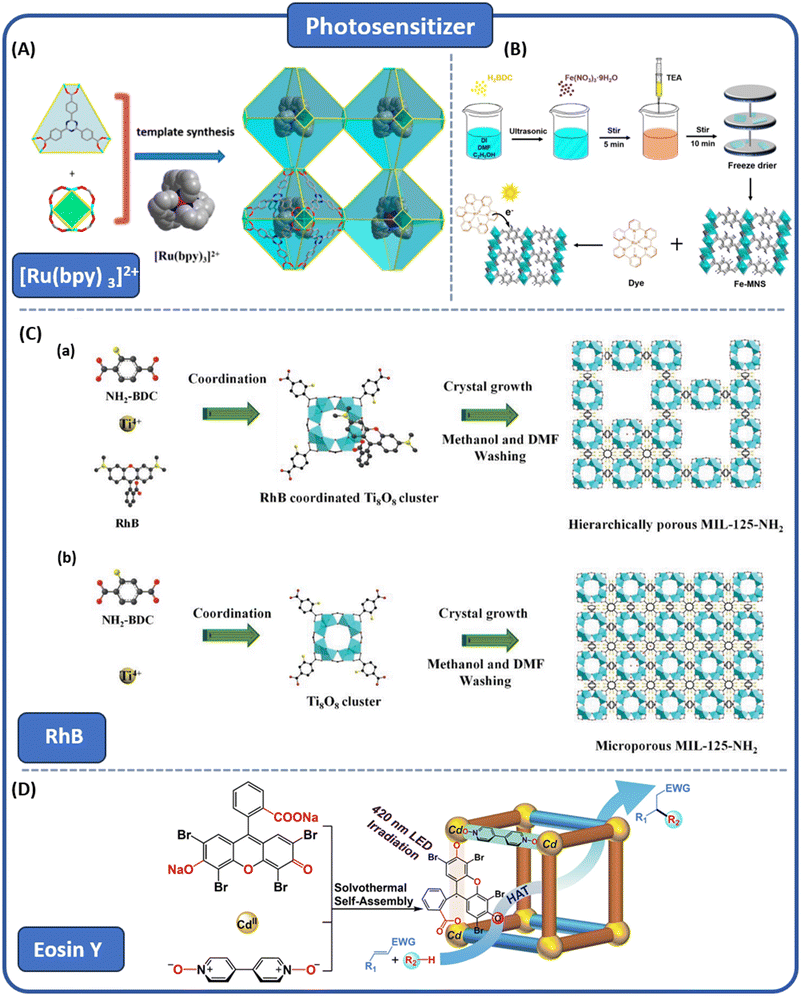 |
| Fig. 11 (A) The aerobic oxidation of benzyl halides and the cycloaddition reaction of tertiary amines with maleimides under visible light catalysed by Ru(bpy)3@MOFs from ref. 109. Copyright 2019, American Chemical Society. (B) Scheme diagram of the preparation of 2D Fe-MNS and dye-sensitized system from ref. 110. Copyright 2021, Elsevier Inc. (C) Schematic diagram of the formation of MIL-125-NH2 by RhB doping from ref. 111. Copyright 2023, Wiley-VCH GmbH. (D) Construction schematic diagram of Eosin Y-based MOF, displaying an illustration of photocatalytic inert C–H bond activation and transformation from ref. 112. Copyright 2022, American Chemical Society. | |
Dye-sensitized systems offer a means to enhance both light absorption and charge separation efficiency, while also allowing for the modulation of the catalyst band structure. Li et al. achieved this by incorporating the visible light-responsive dye sensitizer [Ru(bpy)]32+ into two-dimensional Fe-MOF (ligand = H2BDC) nanosheets (Fig. 11B), resulting in a negative shift of the LUMO potential of two-dimensional Fe-MNS from 0.11 V to 0.15 V (relative to the reversible hydrogen electrode, RHE).110 Consequently, the [Ru(bpy)]32+/Fe-MNS catalytic system demonstrates excellent photocatalytic CO2 reduction activity.
A major limitation in the application of transition metal complexes as photosensitizers lies in their prohibitive expense. To overcome this restriction, several research groups have investigated potential alternatives by employing inexpensive dyes or dye-like groups for sensitizing MOFs. For instance, Jong Hyeok Park et al. utilized the rigid dye modifier rhodamine B (RhB) to controllably adjust the pore size of Ti-based MOF MIL-125-NH2 (Fig. 11C).111 This approach allows for the enlargement of the pore size while preserving the integrity of the MOF crystals, thereby enhancing their photocatalytic activity. Notably, this method exhibits outstanding performance in purifying toluene.
Zhao et al. developed a heterogeneous MOF with excellent performance by incorporating Eosin Y into a porous MOF.112 This modification efficiently facilitates the activation of dormant C–H bonds in reactants through hydrogen atom transfer mechanisms. Notably, the introduction of Eosin Y significantly enhances the chemical stability of the catalyst. Moreover, by introducing a secondary auxiliary ligand, the carbonyl groups of xanthene on the Eosin Y dyes were entirely preserved and periodically aligned within the confined channels of this rigid framework. This arrangement facilitates the formation of excited-state radicals, thereby promoting the activation of inert C–H bonds and enhancing the reaction efficiency (Fig. 11D).
2.4. Heterostructure construction
One effective method to enhance photocatalytic efficiency is to design rational heterojunction photocatalysts, to effectively separate the photogenerated electron–hole pairs in semiconductor photocatalysts.113,114 A heterojunction typically refers to the interface formed by two different semiconductors that satisfy lattice and thermal matching. Currently, there are various types of heterojunction, including Type I, II, III, p–n junctions, Z-scheme heterojunctions, and S-scheme heterojunctions, as in Fig. 12; Type I, II, and III are conventional heterojunctions (A corresponds to semiconductor A, B corresponds to semiconductor B, which semiconductor can be an n-type semiconductor, or a p-type semiconductor).115–117 Combining MOFs with other semiconductors to exploit the synergistic effect of heteromaterials can effectively improve the charge dynamics, and reduce electron–hole recombination.
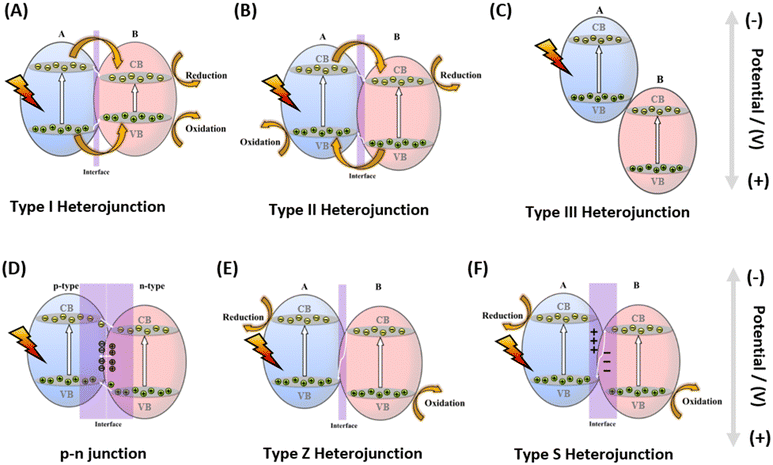 |
| Fig. 12 (A–F) Type I heterojunction, Type II heterojunction, Type III heterojunction, p–n junction, Type Z heterojunction, and Type S heterojunction, respectively. | |
Type I (straddle) heterojunctions have the following characteristics: (1) the conduction band (CB) and valence band (VB) potentials of A are higher and lower than B respectively; and (2) electrons migrate to the B, and holes migrate to the A. Thus electrons and holes are both on B118 (Fig. 12A).
Type II (staggered) heterojunctions have the following characteristics: (1) the CB and the VB levels of semiconductor A are higher than the corresponding levels of the semiconductor B; and (2) there are preferential VB holes at A and CB electrons on B. This results in a spatial separation of electron–hole pairs (Fig. 12B).
Type III (broken gap) heterojunctions have the following characteristics: (1) the staggered gap becomes extreme, resulting in non-overlapping bandgaps; and (2) the electron–hole migration and separation cannot occur. This is not suitable for enhancing the separation of e−–h+ pairs (Fig. 12C).
Among the aforementioned conventional heterojunctions, in Type II heterojunctions, photoinduced electrons and holes are trapped in two spatially separated potential wells, with the resulting holes and electrons moving in opposite directions, effectively promoting the separation of electron–hole pairs.119–122
For example, in 2022, Wang et al. constructed three MOFs with different metal ions but the same ligands (2-aminoterephthalic acid), named In-MOF, Zr-MOF, and Fe-MOF, which were in situ grown on a covalent organic framework (COF) containing triazine (TP-TA), forming a Type II heterojunction for light-driven CO2 reduction reactions.123 Technically, a more negative CB potential would allow a relatively more ready photocatalytic reduction. The order of photocatalytic activity, In-MOF@TP-TA > Zr-MOF@TP-TA > Fe-MOF@TP-TA, aligns with the conduction band (CB) potentials of the MOF components (from negative to positive) (Fig. 13A), thereby corroborating the direction of charge flow within the type II heterostructures. Compared with In-MOF, TP-TA has a more negative CB and a more positive VB, leading to an internal electric field between the VB of TP-TA and the CB of In-MOF, facilitating the separation and migration of electrons and holes.
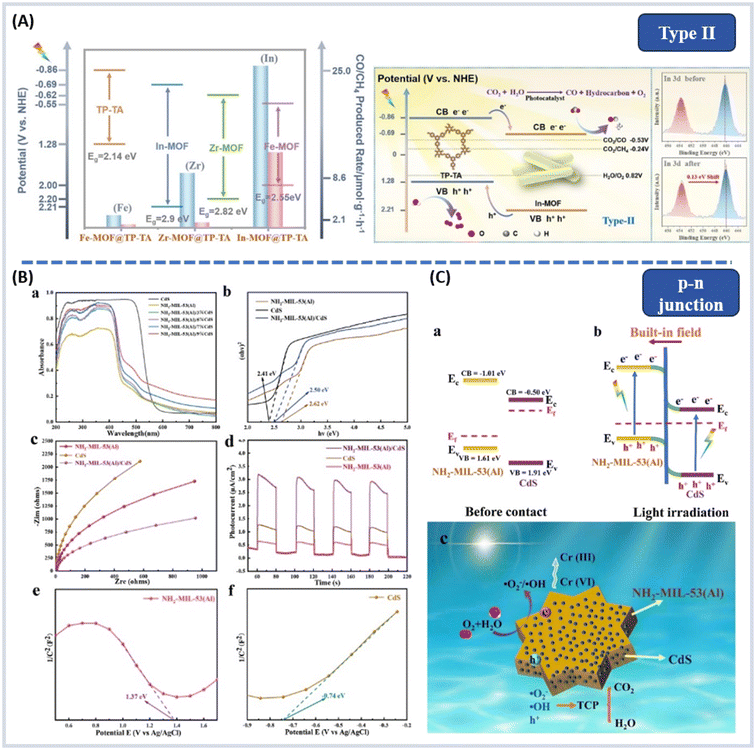 |
| Fig. 13 (A) MOFs’ band-structure and mechanism of photocatalytic CO2 reduction of In-MOF@TP-TA hybrid material, and XPS spectra studies (inset) from ref. 123. Copyright 2021, American Chemical Society. (B) (a) The UV–vis DRS spectra, (b) the band gaps, (c) the EIS curves, (d) photocurrent intensity, and (e and f) Mott–Schottky plots. (C) Possible visible light photocatalytic mechanism of NH2-MIL-53(Al)/CdS p–n heterojunctions from ref. 124. Copyright 2021, Elsevier B.V. | |
Type-II heterojunctions can spatially separate e−–h+ pairs, and the enhancement in e−–h+ separation achieved is insufficient to overcome the ultrafast e−–h+ recombination in the semiconductor. On the other hand, p–n heterojunctions can accelerate e−–h+ migration across the heterojunction, improving the efficiency of charge separation by creating an additional electric field.
p–n junctions (staggered) have the following characteristics: (1) electrons migrate from the CB of the p-type photocatalyst to the n-type photocatalyst, and move from the VB of the p-type photocatalyst to the n-type; and (2) between the interfaces the two semiconductors generated an internal electric field, because there are opposite preferential charge carriers at the closely contacted interface (Fig. 12D).
Zhou et al. used a simple two-step hydrothermal method to synthesize NH2-MIL-53(Al)/CdS p–n heterojunctions (ligand = 2-aminoterephthalic acid (NH2-BDC)). The photocatalytic reduction of Cr(VI) and the removal of the trichlorophenol achieved efficiencies of 99.23% and 98.85% under visible light.124 In Fig. 13B, ultraviolet–visible diffuse reflectance spectroscopy (DRS) indicated that the introduction of CdS effectively expands the absorption range and improves the optical response. Compounding CdS reduces the band gap to 2.50 eV, proving that heterojunction formation narrows the band gap and enhances visible light absorption. Electrochemical impedance spectroscopy (EIS) and photocurrent–time (IT) curves show that NH2-MIL-53 (Al) combined with CdS promotes the separation and transfer of photogenerated charge carriers. Mott–Schottky (MS) tests showed that NH2-MIL-53 (Al) is a p-type semiconductor, and the CdS is an n-type semiconductor. Before contact, the Fermi energy level (Ef) of p-type NH2-MIL-53 (Al) is near the top of the VB, and that of n-type CdS is near the bottom of the CB. After contact, NH2-MIL-53 (Al) moves negatively, CdS moves positively, and the Fermi level drives to equilibrium. The formation of p–n heterojunctions creates a built-in field, favoring spatial charge separation (Fig. 13C).
In 1979, Bard introduced the traditional Z-scheme heterojunction photocatalyst structure, named for its Z-shaped electron transfer process.125 The traditional Z-scheme photocatalytic system consists of two different semiconductors, along with an acceptor/donor pair, but is only applicable in the liquid phase. In 2006, Tada and colleagues arranged the concept of an all-solid-state Z-scheme photocatalyst, consisting of two different semiconductors and a solid electronic mediator between them.126 Not only does it optimize the spatial separation of electrons and holes and the redox potential, but it can also be applied in solution, gas and solid media, thus expanding its range of applications, although the electronic mediators are costly and scarce.127 To address this issue, in 2013, Yu et al. proposed a direct Z-scheme heterojunction photocatalyst that enhances photocatalytic activity in the presence of an electronic mediator, significantly advancing photocatalytic performance.128
In direct Z-scheme heterojunctions (staggered), e− on the CB of B recombine with VB holes on A, and electrons and holes that are longer lived are mostly located on A in the CB and B in the VB, respectively (Fig. 12E).113
In 2023, Feng et al. utilized ultrasonic impregnation to uniformly load metal sulfide ZnCo2S4 (ZCS) nanoparticles with extra metal atoms onto the surface of MOF-199 (Cu, ligand = H3BTC), forming a direct Z-scheme heterojunction for stable and efficient photocatalytic H2 evolution.129 Under simulated sunlight, ZCS/M (ZnCo2S4/MOF-199) exhibited high photocatalytic activity with a hydrogen evolution rate of 11.6 mmol g−1 h−1, surpassing those of MOF-199 and ZCS alone by factors of 48.4 and 83.3, respectively and exhibiting an apparent quantum yield (AQY) of 4.92% at 420 nm. Moreover, the uniform anchoring of ZCS onto the surface of MOF-199 effectively mitigated ZCS aggregation issues, thereby exposing a greater number of active sites. Based on ESR analysis findings, ZCS/M is capable of generating ˙OH under light exposure, suggesting a Z-scheme electron transfer pathway. Due to the lower Fermi level (Ef) of MOF-199 compared with that of ZCS, upon contact between MOF-199 and ZCS, electrons within ZCS will autonomously migrate to MOF-199 until an Ef equilibrium is established between them. Simultaneously, due to electron accumulation on the surface of MOF-199 and electron depletion on the surface of ZCS, a plasmonic field is formed from ZCS to MOF-199, inducing band bending in both MOF-199 and ZCS (Fig. 14A). Owing to band bending and the internal electric field (IEF), photogenerated electrons on the conduction band (CB) of MOF-199 can cascade along the bent band to the valence band (VB) of ZCS, where they recombine with holes, preserving the strong reducing electrons on the CB of ZCS. This study presented a novel strategy for designing and fabricating direct Z-scheme heterojunctions with transition metal sulfides on the MOF platform to enhance photocatalytic hydrogen generation in water splitting. In 2022, Shi et al. modulated the heterojunction interface to promote electron–hole separation; an ultrathin C3N5 nanosheet (N-CN) was prepared by facilely exfoliating the bulk C3N5 and was further applied to the construction of the NH2-UiO-66/N-CN heterojunction.130 Before contact, there was no charge transfer between the NH2-UiO-66 and N-CN (Fig. 14B(a and b)). Fig. 14B(c and d) showed that after the interface contact between NH2-UiO-66 and N-CN, electrons transfer from N-CN to NH2-UiO-66. An electron depletion layer and an electron accumulation layer were formed in the N-CN and NH2-UiO-66, respectively. This forms a space charge region and induces corresponding band bending. Driven by the internal electric field, a Z-scheme charge transfer pathway (Fig. 14B(e)) caused electrons from the CB of NH2-UiO-66 to migrate to the VB of N-CN and recombine with holes there. Photogenerated electrons in the CB of N-CN and holes in the VB of NH2-UiO-66 were retained for the photocatalytic reaction. The NH2-UiO-66/N-CN-2 remained stable over 10 cycles over 30 hours and exhibited a significantly enhanced H2 evolution rate, which is one of the best photocatalysts in UiO-66 family.
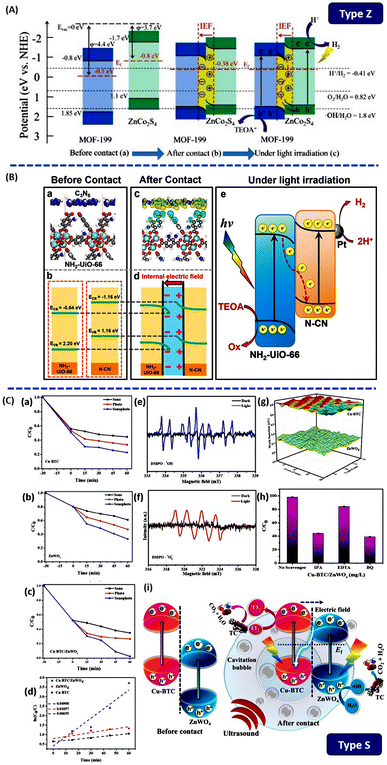 |
| Fig. 14 (A) Schematic diagram of the direct Z-scheme formation process and electron migration path from ref. 129. Copyright 2023, Elsevier. (B) (a and b) Atomistic models of the NH2-UiO-66/N-CN interface and energy-level diagrams, respectively, before contact; (c) calculated charge density differences of the NH2-UiO-66/N-CN interface (yellow color: positive charges; light blue color: negative charges; C: gray; N: blue; O: red; H: white; green: Zr); (d and e) heterojunction energy-levels and proposed mechanism from ref. 130. Copyright 2022, American Chemical Society. (C) (a–c) The degradation of TC, (d) kinetic study, (e and f) ESR spectra of Cu-BTC/ZnWO4 heterostructure, (g) work function, (h) sonophotocatalytic TC degradation in presence of various radical scavengers, (i) degradation of TC using sonophotocatalysis on Cu-BTC/ZnWO4 S-scheme photocatalysts from ref. 134. Copyright 2023, Elsevier. | |
In 2019, a new concept of S-type heterojunction was proposed, first introduced by Professor Jiaguo Yu and his research group. By correcting misunderstandings related to Type II, liquid-phase (traditional) Z-scheme and all-solid-state Z-scheme electron transfer mechanisms, they introduced the innovative concept of the S-type heterojunction.131–133
S-scheme heterojunctions have the following characteristics: (1) if A has a smaller work function and a higher Fermi level, the electron density at the A/B interface is larger on B to create an electron depletion layer and an accumulation layer; and (2) the CB of the reducing part and the VB of the oxidizing part retain strong photogenerated electrons and holes, avoiding the recombination of insignificant photogenerated carriers and introducing a strong redox potential (Fig. 12F).
Bernaurdshaw Neppolian et al. utilized an ultrasonic-assisted hydrothermal method to combine Cu-BTC (ligand = H3BTC) with zinc tungstate (ZnWO4).134 Under visible light irradiation and ultrasound treatment, the Cu-BTC/ZnWO4 heterojunction completely removed tetracycline (TC) within 60 minutes. When subjected to ultrasound and light irradiation at the same time, the degradation efficiency of Cu-BTC increased to 65%, ZnWO4 to 74% and Cu-BTC/ZnWO4 to 98%, as shown in Fig. 14C(a–d). The degradation efficiency of TC by Cu-BTC and ZnWO4 catalysts exhibited the order of sonocatalysis < photocatalysis < sonophotocatalysis. Electron spin resonance (ESR) showed that Cu-BTC/ZnWO4 S-scheme photocatalysts produce ˙O2− and ˙OH under light irradiation. The addition of trapping agents during the sonophotocatalytic degradation of TC indicated that h+ also plays a dominant role Fig. 14C(e–h). The coupling of Cu-BTC and ZnWO4 can produce active species. The prepared Cu-BTC/ZnWO4 heterostructure provided additional nuclei for the formation and growth of cavitation bubbles in the liquid, thereby enhancing the acoustic cavitation. Additionally, these cavitation bubbles generated a significant amount of heat energy during their collapse, increasing the pyrolysis rate of H2O molecules. In sonophotocatalysis, ultrasound eliminates the intermediates produced during degradation through mass transfer, continuously refreshing the surface area and active sites of the Cu-BTC/ZnWO4. Fig. 14C(g) showed that the work functions (Φ) of n-type Cu-BTC and n-type ZnWO4 were 5.5 and 5.13 eV, respectively. Due to the higher EF of Cu-BTC compared with ZnWO4, electrons migrated from the Cu-BTC to ZnWO4 until an EF equilibrium was reached, resulting in Cu-BTC band edge and upward bending, ZnWO4 downward bending, establishing an internal electric field at the interface. The interaction of the internal electric field and Coulomb force promotes the rapid migration of excited electrons in the ZnWO4 CB to the interface, where they recombine with holes in the Cu-BTC VB. The electrons accumulated in the Cu-BTC and the holes accumulated in the ZnWO4 and were retained and actively participated in the reaction. The charge transfer mechanism is shown in Fig. 14C(i). Zr-based MOF, developed by Xu et al., involved combining UiO-66-NH2 with cadmium sulfide nanoparticles (CdS-NPs) using a chemical bath deposition method to form a core–shell structure.135 The presence of type-S heterojunctions not only significantly enhanced the selective oxidation of benzyl alcohol under illumination but also effectively generated H2.
The interface engineering between MOF and COF has proved to be effective at optimizing charge transfer.136 Bi et al. employed a solvothermal method to construct an olefin-linked COF (TTCOF)/NH2-UIO-66(Zr), S-type heterojunction of COF/MOF, which is used for photocatalytic CO2 reduction to CO in gas–solid reaction systems.137 Under light irradiation with only H2O (g) as a mild reducing agent, the CO yield is 6.56 μmol g−1 h−1, which is 4.4 times and 5 times higher than that of the original TTCOF and NH2-UIO-66(Zr), respectively.
In summary, selecting materials with matching energy levels to construct staggered heterostructures like Type-II, Z, and S-type heterojunctions can effectively enhance the photocatalytic efficiency. Additionally, a deeper understanding of the charge-separation dynamics in composite materials can further develop more efficient photocatalysts.138
2.5. Encapsulation with functional guest species
2.5.1. Metal NPs.
The wide application of noble metal single-atom nanoparticles such as Pd, Pt, Ru, Au, Ag, etc., with higher Fermi levels, as co-catalysts, broadens the spectral response range of MOF-based photocatalysts, facilitates photogenerated charge carrier separation and improves the photocatalytic activity of MOFs.139,140
For example, Jiang et al. used UiO-66-NH2 incorporating uniform Pt NPs as an ideal platform to study the effects of interfacial microenvironment modulation (by removing polyvinylpyrrolidone (PVP) from Pt) on electron transfer and photocatalysis.141 The PVP appeared to influence the electron transfer between the Pt and MOF. Compared with PtPVP@UiO-66-NH2, the removal of interfacial PVP boosts photocatalytic H2 production. This provided important insights for adjusting the microenvironment of the catalytic center in photocatalysis. Then Jiang et al. indicated that the position of metal NPs in the MOF affects photocatalysis.142 By incorporated inside or supported Pt NPs on a UiO-66-NH2, Pt@UiO-66-NH2 and Pt/UiO-66-NH2 were obtained. Both composite materials showed increased H2 production activity, but there were significant differences.
Zhao et al. synthesized the Pt/CuII-MOF (CuII-MOF: {[Cu(HL)(BDC)]·0.75H2O}n HL = 4′-(4-hydroxyphenyl)-4,2′:6′,4′′-terpyridine) photocatalyst using the photodeposition method.143 The synergistic effect of dual catalytic active centers promoted the photocatalytic H2 evolution from water. The beneficial electronic interaction between unsaturated coordination CuII ions and uniformly dispersed Pt NPs enhanced the H2 production ability of Pt (4.38 wt%)/CuII-MOF nanoribbons by 2.51 mmol g−1 h−1. This is 4.7 times and 1.9 times higher than that of individual Pt NPs and crystalline CuII-MOF respectively. Herme G. Baldoví et al. also used the photodeposition method to deposit Ru on MIL-125(Ti)-NH2 for the dual photothermal reaction mechanism of carbon dioxide methanation.144 In addition to this, a charge transfer between metal sites and supports is crucial for catalysis. Jiang et al. selected three MOFs that do not respond to visible light, ZIF-8, UiO-66, and MIL-125, to stabilize small-sized Pt nanoparticles; the order of activity for the photocatalytic oxidation of benzylamine is Pt/ZIF-8 > Pt/UiO-66 > Pt/MIL-125.145 XPS and CO-DRIFT (CO adsorption) spectroscopy indicate that different MOFs contribute differently to the electron donation to Pt. Under 450 nm light, the electrons excited in the Pt bands of Pt/UiO-66 and Pt/MIL-125 are injected back into the MOFs, whereas this does not occur in Pt/ZIF-8 due to its higher energy gap. The higher electron density in Pt/ZIF-8, induced by the aforementioned processes, enhances its ability to generate ˙O2−, resulting in the highest electron density and improved oxidative coupling efficiency.
In 2021, Dai et al. first utilized a two-step method to synthesize the ternary heterostructure nanocomposite Pd@UiO-66-NH2@ZnIn2S4, where ZnIn2S4 and UiO-66-NH2 feature a closely interfaced and suitable band gap.146 The introduction of Pd nanoparticles into the photocatalyst, the narrowing of the band gap and the high band gap alignment in Pd@UiO-66-NH2@ZnIn2S4 significantly enhanced the visible light utilization, broadened the spectral response and promoted photogenerated charge carrier separation. In the realm of photocatalytic hydrogen production, 0.3% Pd@UiO-66-NH2@ZnIn2S4 composite material achieved activities of 5.26 and 22.35 mmol g−1 h−1 under visible light and simulated sunlight, respectively. The band gap alignment between the ZnIn2S4 and UiO-66-NH2, along with the incorporation of Pd NPs, broadened the spectral response range, promoted the separation of photogenerated carriers, and enhanced the photocatalytic performance (Fig. 15A and B).
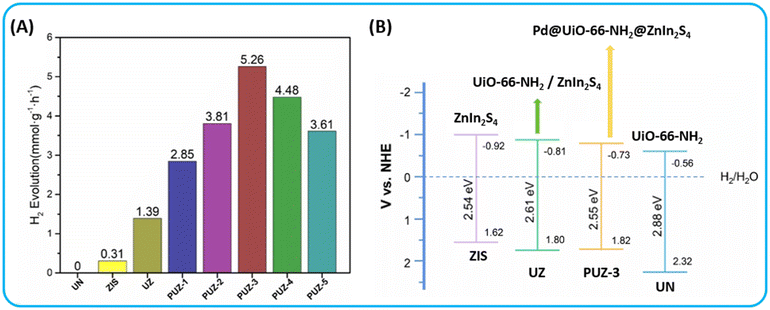 |
| Fig. 15 (A and B) hydrogen production rates of various photocatalysts, UN = UiO-66-NH2, ZIS = Pure ZnIn2S4, UZ = UiO-66-NH2/ZnIn2S4, PUZ = Pd@UiO-66-NH2@ZnIn2S4. Schematic illustration of the band structures of UN, ZIS, UZ and PUZ-3 from ref. 146 Copyright 2021, Royal Society of Chemistry. | |
Furthermore, Jiang et al. designed Pt@MIL-125/Au and Pt/MIL-125/Au, based on the integration of the plasmonic effect and the Schottky junction; Pt@MIL-125/Au exhibited excellent photocatalytic H2 production activity under light irradiation.147 Jiang et al. synthesized UCNPs-Pt@MOF/Au (UCNPs = upconversion nanoparticles, MOF = UiO-66-NH2) composites using plasmonic and upconversion effects,148 where MOF absorbs UV light and Au responds to visible light, and UCNPs convert NIR light to UV-vis that MOF and Au can absorb. Thus, this material has broadband light absorption characteristics from ultraviolet to near-infrared regions, applied for photocatalytic H2 production. In 2021, Wang et al. immobilized AuNPs within UiO-66 particles through impregnation and reduction, forming a uniquely designed gas membrane solution (GMS) reaction interface composed of porous Au@MOF particles.149 The high dispersion and stability of AuNPs facilitated N2 molecule and hydrated proton transfer, enhancing the plasma photocatalytic reaction at the gas–liquid interface. Results showed that under visible light, the ammonia release rate was 18.9 mmol gAu−1 h−1, with an AQE of 1.54% at 520 nm (Fig. 16A).
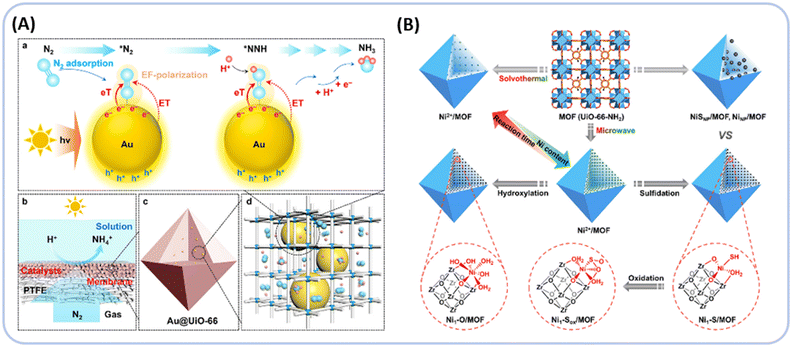 |
| Fig. 16 (A) Schematic illustration for direct P2NRR on AuNPs encapsulated in a UiO-66 matrix from ref. 149. Copyright 2021, Royal Society of Chemistry. (B) Efficient microwave-assisted method in contrast to NiNP/MOF and one-pot synthesized NiSNP/MOF from ref. 156. Copyright 2021, American Chemical Society. | |
Then, Jiang et al. investigated the single-atom alloy (SAA) catalyst for photocatalysis.150 As a result, the Pt component on the Pd surface can be controlled from the shell-form to SAA dispersion precisely, based on the rational control of Pt loading. The photocatalytic H2 production activity of Pd10@Ptx/UiO-66-NH2 can be greatly increased by precisely fabricating the Pd10@Pt1 SAA co-catalyst, based on the rational control of Pt loading.
Transition metal (TM) catalysts, apart from noble metals, have garnered widespread attention in catalysis. Loading active metal NPs onto heterogeneous supports is a prevalent method that maximizes the proportion of active sites and tunes the performance of the catalysts.151–154 For instance, Wang et al. showed that Cu-doped UiO-66 creates modified mesoporous defects, increasing the surface area by 58% compared with UiO-66, which facilitates the adsorption of molecular oxygen.155 Additionally, Cu doping enhances the photocurrent and reduces the interfacial charge transfer resistance, thereby improving the charge separation efficiency. Jiang et al. employed a microwave-assisted method to introduce various earth-abundant metals such as Ni2+, Co2+, and Cu2+ in single-atom form onto Zr6-oxo clusters (Fig. 16B).156 The study specifically focuses on Ni1-X/MOF systems (MOF, UiO-66-NH2 unless otherwise mentioned; Ni1–X, Ni1–S, Ni1–O, Ni1–Sox; ox, oxidation). Among these single-atom catalysts (SACs), Ni1-S/MOF features a unique sulfur-coordinated Ni(I) center with a reduced oxidation state, which offers a significantly lower proton activation barrier compared with its counterparts. The results demonstrate that under visible light, Ni1-S/MOF exhibits the highest photocatalytic H2 production activity, over 270 times that of the original MOF, far surpassing other Ni1-X/MOF catalysts.
Furthermore, metal NPs can also be transformed into structurally precise metal nanoclusters (NCs) for photocatalysis. In 2024, Jiang et al. encapsulated four MAg24 (M = Ag, Pd, Pt, Au) NCs into UiO-66-NH2, through electrostatic interactions. Among them, AuAg24@UiO-66-NH2 exhibited the highest H2 production rate of up to 3.6 mmol g−1 h−1.157 The MAg24 clusters encapsulated into the MOF produced an electron transfer pathway similar to the Z-scheme heterojunction, which promoted charge separation, and optimized Ag electronic state.
2.5.2. Metal–oxide NPs.
TiO2 was a commonly used classical photocatalytic material with the band gap of 3.2 eV.158,159 The use of ZnO in the field of photocatalysis has expanded increasingly.160–162 The ZnO band gap energy (3.3 eV) is very close to that of TiO2, theoretically endowing it with similar photocatalytic properties to TiO2. Fe3O4 has garnered considerable research interest in the field of photocatalysis, particularly because of its magnetic properties, enabling facile separation of the catalyst from solutions.163
Recently, researchers have made efforts to develop metal oxide@MOF nanocomposites as novel photocatalysts.164 A comprehensive summary detailed the effects and applications of semiconductor-like metal oxides such as TiO2, ZnO and Fe3O4 in photocatalysis by Zeng et al.165 Additionally, certain metal oxides such as BiOX (X = I, Br and Cl) have been extensively researched as ideal photocatalysts, where the unique [Bi2O2]2+ layers interlaced with halide ions facilitate electron transfer in various directions while reducing the transfer distance.166 In 2023, Yao et al. developed core–shell Bi-MOF@BiOX (X = I, Br and Cl) heterostructured hybrid materials using a one-pot solvothermal method.167 Utilizing the competitive coordination effects of CAU-17 (ligand = trimesic acid) and BiOX on Bi3+, CAU-17 was transformed into another structurally and compositionally similar Bi-MOF. Bi3+ acted as a “bridge”, facilitating intimate interfacial contact between BiOX and Bi-MOF, greatly accelerating the charge and mass transfer.
CeO2 is a common and frequently used rare-earth metal oxide and has a positive photocatalytic response due to its excellent unique structure and electro-optical properties. Xu et al. synthesized a novel CeO2/Ce-MOF (ligand = 1,3,6,8-tetra(4-carboxylphenyl)pyrene (H4TBAPy)) homologous S-type photocatalyst, by a one-step hydrothermal method.168 CeO2 nanoparticles grow on the surface of cubic Ce-MOF, forming a CeO2/Ce-MOF S-heterojunction structure. Significantly, the CO2 concentration generated by CeO2/Ce-MOF (404.2 ppm) was ≈6.7 and 3.4 times superior to those generated by CeO2 and Ce-MOF, respectively. The AQE at 420 nm is 7.51%, which is 6.7 times and 3.4 times that of CeO2 and Ce-MOF, respectively. The improvement in efficiency is primarily attributed to two factors: (i) the crucial role of the effective separation capability of photogenerated carriers and prolonged exciton reaction lifetime; and (ii) the formation of an internal electric field (IEF) being sufficient to overcome the relatively large exciton binding energy. This also increases the dissociation efficiency of the electron–hole pairs by 50.4% (Fig. 17A(a and b)).
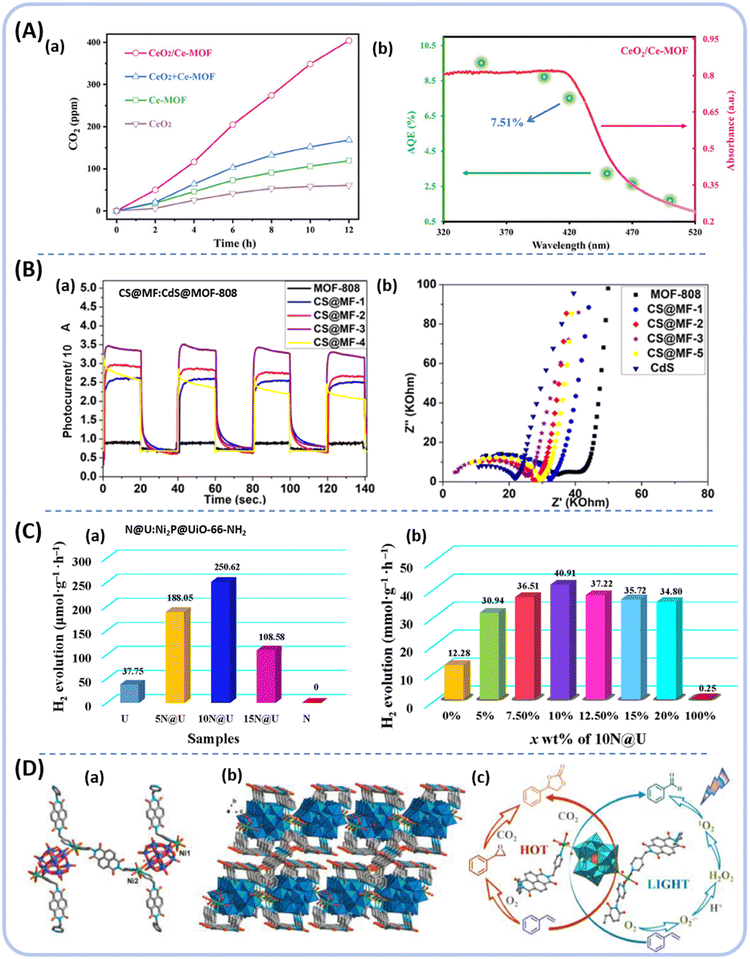 |
| Fig. 17 (A) (a) Time course curve of CO2 liberation from CH3CHO decomposition for samples, (b) AQE of CeO2/Ce-MOF from ref. 168. Copyright 2023, Wiley-VCH GmbH. (B) (a) Transient photocurrent response, (b) EIS Nyquist plots of MOF-808, CdS@ MOF-808-X, and CdS from ref. 176. Copyright 2021, American Chemical Society. (C) (a) H2 production rates with different contents of x mg Ni2P@UiO-66-NH2, (b) x% 10 mg Ni2P@UiO-66-NH2/Zn0.5Cd0.5S (x% 10 N@U/ZCS) with different contents of 10 N@U cocatalyst from ref. 187. Copyright 2022, Elsevier B.V. (D) (a) Ball-and-stick representation of NiW-DPNDI, (b) 3D open network of NiW-DPNDI, (c) the design concept of NiW-DPNDI for photocatalysis styrene oxidation from ref. 194. Copyright 2023, Elsevier B.V. | |
2.5.3. Metal-sulfide NPs.
Traditional semiconductor photocatalysts can only absorb UV light, with wavelengths less than 387 nm.169–171 Metal sulfides, with suitable conduction band positions and narrow band gaps, such as CdS with a band gap of ∼2.4 eV and a fairly negative conduction band potential, exhibit an excellent response to visible light.172–175
Consequently, in 2021, Hou et al. synthesized porous CdS@Zr-MOF photocatalysts based on representative catalysts such as CdS and Zr-MOF (ligand = H3BTC), which were used for the synthesis of imines in air.176 The transient photocurrent response and EIS Nyquist plots showed that CdS@MOF-808-X (1–4 for different ratios of CdS loading of MOF-808) had a higher photocurrent response, more efficiency of separation and a transfer of photogenerated carriers (Fig. 17B(a and b)). Among the CdS@MOF-808 composites, CdS@MOF-808-3 showed both a photocatalytic activity yield (95%) and selectivity (nearly 100%) in the oxidative coupling of amines.
Shi et al. synthesized a new photocatalyst, PdS@UiOS@CZS (UiOS: thiol-functionalized UIO-66, CZS: Cd0.5Zn0.5S).177 PdS quantum dots were encapsulated within its cage and CZS solid solution NPs were anchored on its outer surface. The resulting PdS@UiOS@CZS exhibited a photocatalytic hydrogen evolution rate of 46.1 mmol h−1 g−1 irradiation from 420 to 780 nm. The encapsulation of PdS QDs enhanced the ability of the UIOS pores to attract holes. Huo et al. coated CdS NPs on Ni-MOF, and a 3D stratified CdS/Ni-MOF composite with a tightly connected interface was successfully fabricated.178 In the prepared photocatalysts, the 20%-CdS/Ni-MOF demonstrated the highest CO production rate.
Moreover, MoS2 features a tunable bandgap structure, ranging from an indirect bandgap of 1.2 eV in bulk MoS2 to 1.8 eV in monolayer MoS2, which can be activated under visible light conditions.179–182 Woong Kim et al. investigated a photocatalyst driven by the MoS2@MIL-88(Fe) (ligand = BDC) interface, which consists of molybdenum disulfide (MoS2) anchored to an MOF and is prepared for activation under visible light illumination.183 MoS2@MIL-88(Fe) composite material exhibited a narrow optical bandgap of 2.75 eV. The MoS2 and MIL-88(Fe) provide effective redox sites for the mineralization of MB and RhB dyes. Reusability studies indicate that after five cycles, its photocatalytic activity remains excellent.
2.5.4. Metal-phosphide NPs.
Transition metal phosphides (TMPs), such as Ni2P, Ni12P5, and Co2P, have been reported as alternative noble metal co-catalysts for photocatalytic H2 production owing to their unique structure and electronic properties.184–186 In 2022, Ge et al. used an in situ solvothermal method to encapsulate Ni2P within UiO-66-NH2. The Zn0.5Cd0.5S was decorated with a core–shell Ni2P@UiO-66-NH2 cocatalyst, forming ternary Ni2P@UiO-66-NH2/Zn0.5Cd0.5S composite materials.187 When Ni2P was wrapped in pure UiO-66-NH2, the catalytic H2 evolution activity significantly increased (Fig. 17C(a)), with the hydrogen production rate peaking at 10 mg of Ni2P addition. Fig. 17C(b) illustrated the effect of 10 Ni2P@UiO-66-NH2 content on the photocatalytic capability of ZCS sulfide. However, further increases in decorating capacity reduced the hydrogen production rate, and this likely due to excessive cocatalyst shielding of active sites on ZCS. Ni2P played a crucial role in this ternary photocatalyst.
Sasanka Deka et al. have for the first time developed a novel type of hollow ZnCo-MOF (ligand = 2-methylimidazole) spheres at room temperature, which were loaded with monodisperse transition-metal phosphide (TMPs), including NiCoP, FeCoP, Ni2P and CoP nanoparticles. These composite materials could act as efficient non-noble metal catalysts for hydrogen evolution reactions.188 Compared with systems based on Pt cocatalysts, these are not only more economical but also exhibit comparable excellent photocatalytic hydrogen evolution activity to Pt@ZnCo-MOF (Pt@ZCM), achieving 8583.4 μmol h−1 g−1 with an AQY of 28.2%. This achievement is related to the efficient n–n heterojunction charge separation and transfer, as well as the NiCoP cocatalyst reducing the reaction activation energy, thereby facilitating rapid hydrogen evolution kinetics. Jin et al. synthesized the Ni-MOF-74/Ni2P (ligand = H2BDC) precursor using a low-temperature phosphorization method.189 Using a solution mixing method, they produced a uniquely structured Ni-MOF-74/Ni2P/MoSx ternary heterostructure; the introduction of Ni2P enhanced the composite catalyst utilization of visible light, leading to increased electron generation and thus improved photocatalytic hydrogen evolution activity.
2.5.5. Polyoxometalates (POMs).
Polyoxometalates (POMs) are multinuclear, anionic metal oxide clusters composed typically of early transition metals in their highest oxidation states, such as W(VI), Mo(VI), or V(V).190,191 In POMs, the high oxidation states of the metal centers mean their lowest unoccupied molecular orbitals are composed of linear combinations of d-orbitals from metals with a strong tendency to accept electrons, allowing for multiple reversible reductions within a relatively narrow potential range. Leveraging their high stability and tunability, POMs have been successfully used to enhance the photocatalytic activity of heterogeneous catalysts.192 Consequently, Carsten Streb et al. reviewed the embedding of POM guests into MOF hosts and the synergistic effects of the resulting composites in multiphase catalysis.193 Following this, the field continued to develop. Han et al. synthesized a photoactive polyoxometalate-based MOF (NiW-DPNDI) using a solvothermal approach, comprising Ni(II) ions, [ZnW12O40]6− anions and N,N′-bis(4-pyridylmethyl)naphthalene diimide (DPNDI) molecules.194 The strong anion–π interaction between the [ZnW12O40]6− anion and the electron-deficient naphthalenic ring centroid, along with the π–π interaction between DPNDI groups, facilitates electron–hole separation and creates a short charge transfer pathway. NiW-DPNDI exhibits high efficiency and diverse chemical selectivity in the oxidation of styrene under both thermal and photocatalytic conditions (Fig. 17D).
2.5.6. Metal-free semiconductors.
Combining zero-dimensional (0D) quantum dots (QDs) with two-dimensional (2D) MOFs to create 0D/2D hybrid catalysts is an effective method to address the rapid recombination of photogenerated electron–hole pairs.195 Carbon dots (CDs) are a type of special carbon nanomaterial less than 10 nm in size.196,197 They are widely noted for their luminescence, low toxicity, stability, and easy production.198–200 Since CDs can act both as electron acceptors and photosensitizers and their ultra-small size allows for strategic encapsulation within the pores of MOFs, enhancing their photoelectron transfer efficiency, it is feasible to develop CD-hybridized MOFs photocatalysts. The MOF structure facilitates charge/mass transfer and increases the surface area to volume ratio of CD active sites.201
Li et al. successfully synthesized NH2-UiO-66 particles modified and embedded with CDs, as shown in Fig. 18A.202 The placement of the CDs significantly affects the photocatalytic activity of the NH2-UiO-66 particles. In addition, the incorporated CDs form small heterojunctions with the Zr–O clusters in the MOF, resulting in the MOF photocatalysts demonstrating rapid charge separation and transfer. Besides acting as electron acceptors, under long-wavelength light, CDs also serve as photosensitizers in MOF photocatalysts. The main difference between the two materials lays in the differing positions of CDs within the hybrid particles. As shown in Fig. 18B(A) and (B), CD/NH2-UiO-66 particles display a uniform size with a mean diameter of 180 nm, and under TEM (Fig. 18B(C)) the particle shows an octahedral shape with 3–4 nm tiny dot-like particles on the MOF surface. When CDs are encapsulated within MOF particles, the resulting CD@NH2-UiO-66 particles exhibit a similar octahedral morphology, but with an average size of about 150 nm (Fig. 18B(D) and (E)), while some black dots are observed under the MOF shell (Fig. 18B(F)). CD@NH2-UiO-66 exhibited higher CO2 reduction activity.
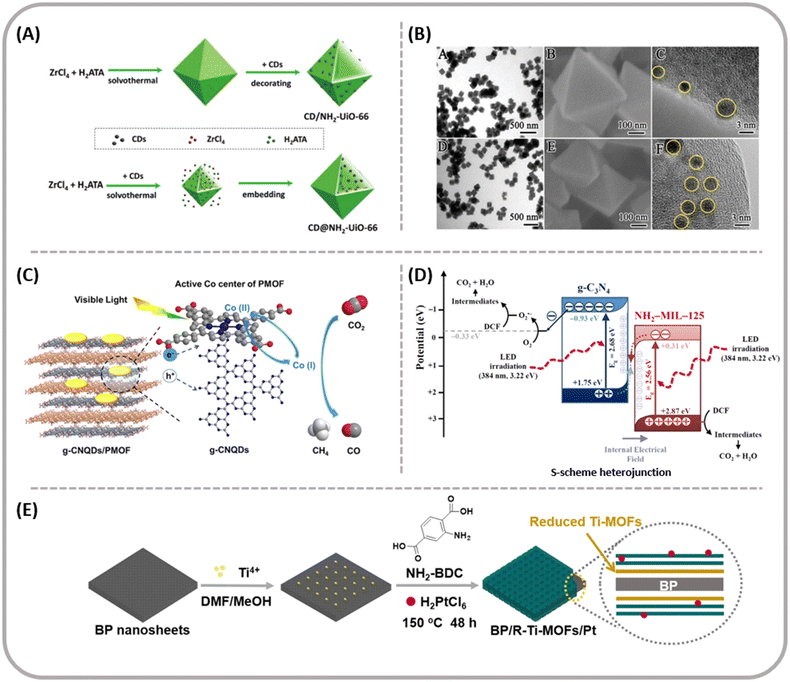 |
| Fig. 18 (A) Schematic illustration of CD-decorated NH2-UiO-66 particles and CD-embedded NH2-UiO-66 particles, respectively. (B) TEM, SEM and high-resolution TEM characterization. (A, B, and C) CD-decorated NH2-UiO-66 particles, (D, E, and F) CD-embedded NH2-UiO-66 particles from ref. 202. Copyright 2020, Royal Society of Chemistry. (C) Proposed mechanism of CO2 reduction over g-CNQDs/PMOF hybrids under visible-light irradiation from ref. 203. Copyright 2019, American Chemical Society. (D) Charge transfer of g-C3N4/Ce-BDC heterojunction from ref. 214. Copyright 2021, Elsevier B.V. (E) The synthesis of BP/R-Ti-MOFs/Pt from ref. 224. Copyright 2020, Elsevier B.V. and Science Press. | |
Wu et al. synthesized a novel hybrid catalyst by integrating two-dimensional (2D) ultrathin porphyrin MOF (PMOF) with zero-dimensional (0D) graphitic carbon nitride quantum dots (g-CNQDs).203 In the synthesized g-CNQDs/PMOF hybrid, g-CNQDs coordinate with the Co active sites in the porphyrin MOF, directly synergizing with the Co in the PMOF as compared with the bare PMOF (Fig. 18C). The migration of photogenerated carriers and gas substrates from g-CNQDs to the Co active centers is faster and more efficient. The production rates of CO and CH4 are 6.10 μmol g−1 h−1 and 6.86 μmol g−1 h−1, respectively.
Additionally, Wang et al. provided a detailed introduction to typical synthesis methods of CDs and MOFs composites, and then they prospected composites in photocatalysis and sensing applications.204
The unique properties of C60 in photocatalytic reactions have attracted the attention of researchers.205 Zhu et al. developed a new type of MOF photocatalyst by encapsulating C60 into the nanoscale zirconium-based MOF, NU-901 (ligand = 1,3,6,8-tetrakis(p-benzoate) pyrene).206 Host–guest interactions, the uneven charge distribution, and the electrostatic potential difference in the C60@NU-901 created a strong built-in electric field, effectively separating and transporting charge carriers to the surface. The electric field in C60@NU-901 is 10.7 times higher than in NU-901 alone and the photocatalytic hydrogen evolution of C60@NU-901 reached 22.3 mmol g−1 h−1.
Carbon nitride can exist as allotropes with different properties, with graphitic carbon nitride considered the most stable.207 Due to its moderate band gap (about 2.7–2.9 eV), reasonable electronic band structure, non-toxicity, low cost, good stability and ease of preparation, it has attracted widespread attention.208–211 In 2008, Wang and his colleagues first used g-C3N4 as a metal-free photocatalyst for hydrogen production.212,213 V. Muelas-Ramos et al. used a g-C3N4/NH2-MIL-125 (ligand = 2-amino benzene dicarboxylic acid) composite photocatalyst under LED irradiation to degrade diclofenac in water.214 The presence of MOF reduces the e−–h+ recombination rate of the g-C3N4. The valence band maximum (VBM) was estimated from XPS, with the VBM of NH2-MIL-125 and g-C3N4 being 2.87 and 1.75 eV, respectively. The CB of NH2-MIL-125 and g-C3N4 were approximately calculated as 0.31 and −0.93 eV (ECB = VBM − Eg). As shown in Fig. 18D, NH2-MIL-125 acts as an oxidative photocatalyst, while g-C3N4 serves as a reductive photocatalyst. The electrons in the CB of the MOF recombine with the holes in the VB of g-C3N4, leaving behind species with strong redox capabilities which can improve diclofenac degradation.
Graphene is a two-dimensional carbon allotrope that was first isolated in 2004 through mechanically exfoliating a single layer of graphite atoms.215 Graphene sheets are single layers of sp2 hybridized carbon atoms arranged in a flat honeycomb lattice. This structure gives graphene its unique physical and chemical properties, including distinctive surface characteristics, excellent conductivity and high surface area, which have been used to construct various nanocomposite materials.216–219
Lu et al. achieved a unique enhanced synergistic effect on MIL-53 (Fe) using ultra-small γ-Fe2O3 and GO via a hydrothermal method.220 Compared with traditional surface coating methods, incorporating γ-Fe2O3 (average particle size 2–3 nm) into the interiors of MOF particles increased the photocatalytic absorption and formed numerous micro-heterojunctions with the MOF units, and then effectively facilitated photogenerated carrier separation. By providing attachment points for MOF crystals, functionalized GO reduced the size of the MOF particles to 100–200 nm and improved their dispersibility. The large specific surface area and high electronic conductivity of graphene oxide also enhanced the active sites and quantum yield of the photocatalytic reaction. Consequently, γ-Fe2O3-MIL-53(Fe)-GO composites significantly enhanced the photocatalytic activity against norfloxacin.
Black phosphorus (BP), one of the allotropes of phosphorus and a 2D material, shows broad application prospects in the field of photocatalysis.221 It features tunable direct bandgaps ranging from approximately 2.1 eV for monolayer phosphorene to about 0.3 eV for bulk BP. It possesses high free carrier mobility and an absorption range from visible to near-infrared light. Combined with MOFs, it is expected to have even broader application prospects.222,223 Xu et al. reported a simple method for the synthesis of BP/R-Ti-MOF/Pt using an in situ synthesis approach (Fig. 18E).224 Ti4+ ions initially accumulate on the surface of BP to form BP/Ti complexes. Subsequently, in the presence of chloroplatinic acid (H2PtCl6), a solvothermal method is used to further grow BP/Ti complexes in a high concentration of NH2-BDC, yielding BP/R-Ti-MOF. Strong interactions between Ti and P lead to the reduction of Ti4+ valence states on the surface of the BP, forming abundant reducible Ti4+ species within R-Ti-MOFs/BP. This reduction in Ti4+ results in BP/R-Ti-MOFs/Pt showing enhanced photocatalytic hydrogen production efficiency compared with Ti-MOFs/Pt and BP + Ti-MOFs/Pt (physically mixed), with a bandgap width of 2.12 eV, lower than Ti-MOFs/Pt 2.58 eV, showing efficient charge transfer and light absorption abilities.
2.6. Morphology regulation
The morphology of the catalyst is one of the key factors influencing photocatalytic activity. The morphological control of photocatalysts includes the regulation of crystal shape (1D, 2D, and 3D), size, and planarity. Research has shown that different shapes of MOF nanomaterials can be synthesized through simple coordination modulation strategies.225–227 Gu et al. constructed nanorod self-assembled Bi-based MOF microspheres (Bi-MOF-M, ligand = H2BDC) using an ethylene glycol-assisted solvothermal method.228 The SEM of Bi-MOF-M was shown in Fig. 19A(a–c). After the in situ growth of Bi2MoO6 on the surface of Bi-MOF-M, the surface structure had an alteration, with the nanorods nearly invisible. The surface layer of the as-prepared composite shows a flower-like structure composed of petal-like nanosheets, and as shown in Fig. 19A(d–f) the petal thickness is about 20 nm. This method easily created a chemically bonded heterojunction interface. This allows it to maintain high catalytic activity of the primary nanocomponents while also being easily recoverable due to its micrometer-level material. It has exhibited high performance under visible-light in the degradation of tetracycline.
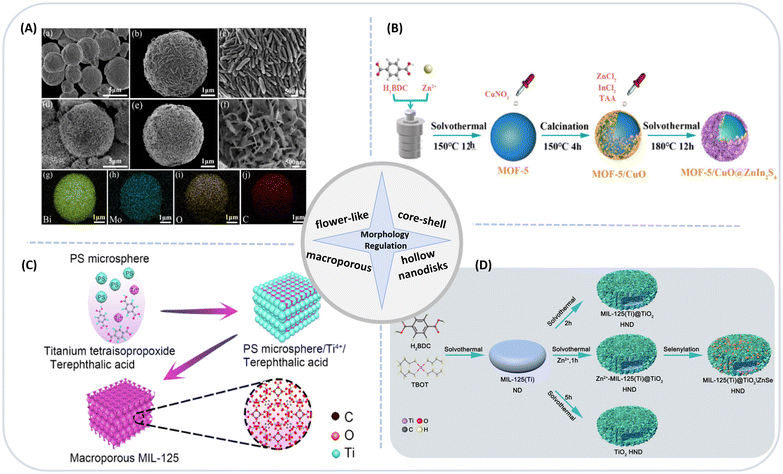 |
| Fig. 19 (A) The SEM images of Bi-MOF-M and Bi-MOF-M/BMO-0.3 from ref. 228. Copyright 2021, Elsevier Inc. (B) Schematic diagram for the formation of the MOF-5/CuO@ZnIn2S4 tandem heterojunction from ref. 229. Copyright 2022, Royal Society of Chemistry. (C) Synthesis procedure of the highly ordered macroporous MIL-125 from ref. 232. Copyright 2019, American Chemical Society. (D) Schematic diagram of the synthesis of MIL-125(Ti)@TiO2/ZnSe HNDs from ref. 237. Copyright 2022, Elsevier Inc. | |
Zhou et al. synthesized a 3D floral spherical shaped MOF-5/CuO@ZnIn2S4 core–shell Z-scheme heterojunction using a two-step solvothermal and oxidation method (Fig. 19B).229 The flower-ball-shaped core–shell structure provided numerous active sites for photocatalysis. The 3D floral spherical shape and its photothermal effect promote charge separation and accelerate photocatalysis. When applied to H2 production and the degradation of 2,4-dichlorophenol and phenol, the photocatalytic H2 production rate (1938.3 μmol g−1 h−1) was 18 times that of the original MOF-5, with photocatalytic degradation efficiencies for 2,4-dichlorophenol and phenol reaching 98.7% and 97.3%, respectively.
Liu et al. utilized Ce-MOFs (ligand = 1,4-dicarboxybenzene) as a template to rationally design and construct a Ce-doped ZnIn2S4 tetrakaidecahedron nanocage (ZTNs-Cex) photocatalyst for photocatalytic hydrogen evolution through a one-step hydrothermal method.230 The Ce-doped ZnIn2S4 featured a non-spherical 3D hollow nanostructure, inheriting the tetrakaidecahedron shape from the Ce-MOF template. By modifying the morphology, the light-harvesting ability is significantly improved, and the separation of photogenerated charge carriers is facilitated. The optimal ZTNs-Ce20 showed a hydrogen evolution photocatalytic activity of 7.46 mmol h−1 g−1 and an AQE of 6.56% at 380 nm, which are three times that of the original ZnIn2S4 (2.61 mmol h−1 g−1).
As reported by the IUPAC definition, porous materials are classified into microporous (pore size <2 nm), mesoporous (pore size 2–50 nm) and macroporous materials (pore size >50 nm), each showing significant value in specific applications. Macroporous materials can serve as reaction sites for polymer polymerization.231 Li et al. synthesized highly ordered hierarchical macroporous MOF materials (macroporous MIL-125) via a one-step solvent evaporation-induced self-assembly route (Fig. 19C).232 The obtained macroporous MIL-125 exhibits an extremely high specific surface area, with a BET surface area of up to 1083 m2 g−1. Furthermore, the macroporous MIL-125 exhibits an orderly hierarchical porous structure and excellent light absorption capabilities. Macroporous MIL-125, used as a photocatalyst for CO2 capture, exhibits high cycling stability, good tolerance to substrates and excellent yields in the CO2 carbonyl coupling reactions under ultraviolet light.
Compared with ultrathin MOFs, hollow structures with internal cavities and thin shells not only expose more active sites but also provide confined spaces for catalytic reactions and product separation.233–236 Tian et al. synthesized ZnSe-sensitized hierarchical MIL-125(Ti)@TiO2 hollow nanodisks (HNDs) using a two-step solvothermal process followed by a selenization technique (Fig. 19D).237 In the ternary hybrid, MIL-125(Ti)@TiO2 displays a hierarchical hollow sandwich-like heterostructured nanodisk, with TiO2 nanosheets distributed on both sides of the MIL-125(Ti) nanoshell. The in situ formed ZnSe nanoparticles enhance the visible light absorption of the MIL-125(Ti)@TiO2/ZnSe HND hybrid. Consequently, the optimized mixture features abundant catalytic sites, high charge separation efficiency and broad visible light absorption, and thus enhanced photocatalytic CO2 reduction performance and recyclability compared with single-component catalysts and binary hybrid catalysts.
2.7. Defect engineering
Defects in MOFs refer to regions where the regular periodic arrangement is disrupted due to the absence or misplacement of atoms or ions within the framework.238 Defect engineering is regarded as an effective method to regulate the properties of MOFs, such as photogenerated carrier mobility, pore size, number of catalytic active sites, and band gap.239,240 Maria Yaseen et al. effectively prepared a series of defect MOFs (D-NUiO66) Zr-UiO-66-NH2 using a modular acidic method (HCl).241 The optimized D-CeNUiO66 photocatalyst efficiently reduced CO2-to-CO with a peak rate of 38.6 μmol g−1 h−1 and demonstrated significant reproducibility in CO production. The integration of defect sites in D-NUiO66 enhanced the photoresponse to visible light, resulting in a reduced band gap of 2.9 eV. Jiang et al. synthesized an MOF featuring controlled structural defects, named UiO-66-NH2-X (X represents the molar equivalents of the modulator, acetic acid, with respect to the linker in synthesis).242 Structural defects in MOF can turn on photocatalysis. The H2 production showed a volcano-type trend with increasing structural defects, where Pt@UiO-66-NH2-100 exhibited the highest activity. This highlights that creating moderate structural defects is crucial for promoting efficient electron–hole separation.
3. Applications
In recent years, there has been rapid progress in the development of visible light-responsive MOFs. Extensive research on these materials has led to the emergence of numerous visible light-responsive MOFs and their composite materials. Many researchers have widely applied MOFs and their composites as efficient and recyclable photocatalysts in the field of photocatalysis. This part includes pollutant degradation, carbon dioxide (CO2) reduction, nitrogen (N2) fixation, hydrogen (H2) production, and organic transformations under light irradiation.
3.1. Pollutant degradation
These pollutants include dyes,243–245 antibiotics,246–248 and polycyclic aromatic hydrocarbons.249,250 Various types of heavy metal and organic pollutant are often stable in water, posing significant hazards despite their low concentrations. Among heavy metals, hexavalent Cr(VI) (chromium) is a common pollutant in surface and groundwater from many industrial processes,251,252 such as electroplating, tanning, metallurgy, metal finishing and petroleum refining. Studies have shown that Cr(VI) concentrations exceeding 0.1 ppm can have lethal effects on aquatic life. Similarly, over the past few years, more than 100
000 tons of antibiotics have been used globally, and extensively applied to treat bacterial infections in humans. Antibiotics are difficult for the human to metabolize and are directly excreted into the natural environment. Antibiotic resistance genes are a result of antibiotic accumulation in aquatic environments, posing a threat to human survival.195,253–255 Current technological solutions to pollution, such as adsorption,256 membrane separation,257 advanced oxidation processes,258 and photocatalytic degradation have been widely applied.259–262 Among these, photocatalytic technology, which utilizes light energy, has gained significant attention in recent years.
MOFs demonstrated significant advantages in water treatment, offering an effective strategy for pollutant removal. The use of semiconductors to degrade organic pollutants under visible light has proved to be an effective means of mineralizing harmful substances.263–265 In this process, pollutants are oxidized into compounds such as CO2, H2O, NO3−, or other oxides, phosphates, sulfates, and halides. This mineralization method, compared with others like ozonation, chlorination, and adsorption, does not use strong oxidizers or toxic substances, thereby not producing solid waste or polluting the atmosphere.266,267 The degradation of organic pollutants depends on the ability of MOF-based photocatalysts to generate ROS (such as ˙O2− and ˙OH) under visible light. Thus, modifying MOFs in various ways can facilitate the separation of photoinduced electrons and holes within the MOFs, thereby enhancing their photocatalytic performance.268
Within these MOFs, d-block transition metal-based MOFs (e.g., Zn(II), Fe(II)/(III), Co(II)/(III), Zr(IV), Ti(III)/(IV), Ni(II), Cd(II) and Cu(II)) demonstrate significant potential in photocatalytic activities aimed at environmental cleanup. Ligand regulation can effectively modify the band gap, which in turn boosts the efficiency of photocatalysis.269
According to reports, UiO-66 as a catalyst for degrading pollutants still suffers from low electron–hole separation efficiency. The mismatch in pore sizes also makes it difficult to efficiently adsorb and degrade pollutants.270–272 Kevin C.-W. Wu et al. reported a Zr-based MOF photocatalyst named VNU-1 that was synthesized using a solvothermal method.273 VNU-1(VNU represents Vietnam National University), a Zr-based MOF, was constructed using the bidirectional linker H2CPEB (1,4-bis(2-[4-carboxyphenyl]ethynyl)benzene). The designed MOF has a longer linker that enlarges the pore size and enhances the optical properties. The surface areas and pore size distributions of various Zr-based MOFs were analyzed using N2 adsorption–desorption isotherms and NLDFT simulation, demonstrating that larger linkers enhance pore dimensions. Fig. 20A(a) showed the widest light absorption spectrum, as measured by UV-Vis, signifying superior light-harvesting efficiency. TDDFT calculations confirmed that introducing amino functional groups to UiO-66-NH2 expands its light absorption range to the visible spectrum through sp2 hybridization of carbon and nitrogen in the aromatic rings. In Fig. 20A(b), the bandgap values decreased sequentially from UiO-66 (3.97 eV) to UiO-67 (3.63 eV) and further to VNU-1 (2.28 eV), indicating that longer organic linkers enhance charge separation. This phenomenon can be attributed to an increase in the carbon states within the organic linkers, raising the valence band maximum (VBM) and reducing the bandgap. Fig. 20A(c) clearly shows that the lifetime of photoinduced electron–hole pairs in VNU-1 increases from 0.47 ns to 0.63 ns. Compared with UiO-66 MOF, VNU-1 exhibited a 7.5 times higher adsorption capacity. It achieved 100% photodegradation of sulfamethoxazole within 10 minutes. Furthermore, in systems mixed with antibiotics of varying sizes and humic acids, it can selectively adsorb small-molecule antibiotics, leaving other natural organic components (such as humic acids) in the water. Ti3C2 is a typical 2D MXene material and can serve as an electron trap for the effective transfer of photogenerated charges.274 Li et al. synthesized bimetallic Sn-Bi-MOF (ligand = H2BDC) nanoparticles using the solvothermal method and grew them on the surface of Ti3C2, forming a Sn-Bi-MOF/Ti3C2 Schottky junction.275 When the layered structure of Ti3C2 contacts the bimetallic Sn-Bi-MOF nanoparticles, the resulting Schottky junction accelerates electron transfer to Ti3C2, effectively promoting electron transfer and carrier separation, thereby generating free radicals and enhancing the photocatalyst performance in the photocatalytic degradation of tetracycline.
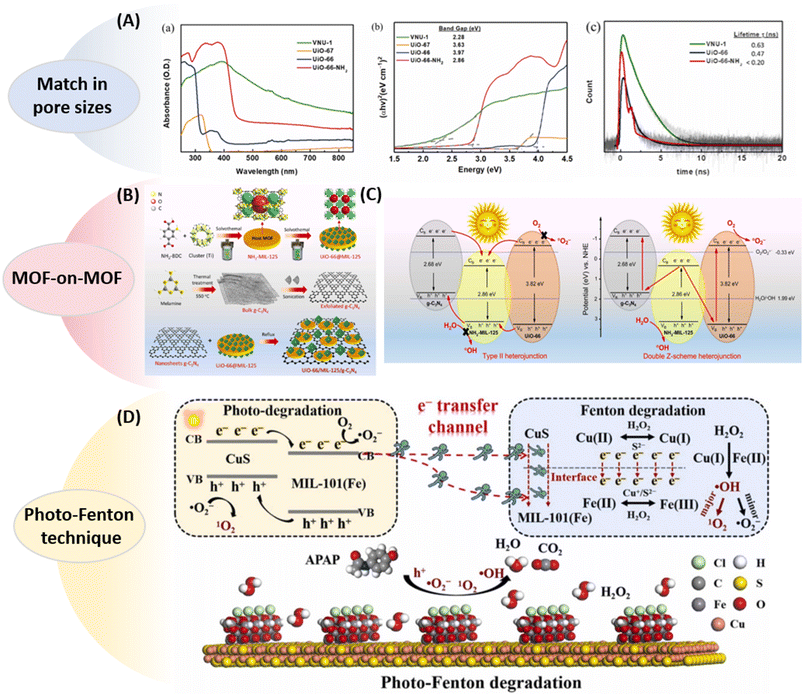 |
| Fig. 20 (A) (a) UV–vis spectra, (b) Tauc plot, and (c) time-resolved PL analysis of Zr-based MOFs from ref. 273. Copyright 2023, Elsevier B.V. (B) Schematic representation for the fabrication of UiO-66/MIL-125/g-C3N4 hybrid structure. (C) Schematic representation of the reaction mechanism of UiO-66/MIL-125/g-C3N4 photocatalyst from ref. 285. Copyright 2022, Elsevier B.V. (D) The possible photo-Fenton degradation mechanism from ref. 292. Copyright 2022, Elsevier B.V. | |
MOF-on-MOF composites represent a versatile and promising series of new materials. MOF-on-MOF is commonly constructed using the epitaxial growth strategy.276,277 Within the reaction system, two types of MOF with matching lattice structures are used; one MOF acts as a “seed” upon which the other grows epitaxially. Hou et al. used the epitaxial growth strategy in which PCN-134 (ligand = 1,3,5-tris(4-carboxyphenyl) benzene (BTB), TCPP) is used as an MOF seed to grow rod-like PCN-222 secondary MOFs on its surface.278 The prepared lines of P21-X exhibited excellent visible photocatalytic performance for the degradation of NZT (nizatidine). Among them, P21–3 exhibits the best catalytic activity, achieving a removal rate of 90.81% within 120 minutes of light exposure. MOF-on-MOF configurations can additionally create heterojunctions that enable the reverse migration of electrons and holes via the CB and VB, effectively enhancing charge separation and carrier mobility.279–281 Combining advanced oxidation processes (AOPs) that utilize visible light to generate reactive oxygen species (ROS) under sunlight can degrade pollutants in a more efficient and environmentally friendly way.282–284 A new generation of double Z-scheme UiO/MIL/CN heterojunctions was designed for ofloxacin photodegradation by Mohammad Ali Zolfigol et al.285 NH2-MIL-125 was first synthesized using the solvothermal method as a host MOF, followed by the growth of UiO-66 crystals on the surface of NH2-MIL-125 with the same technique. Then g-C3N4 nanosheets were modified on the surface of Zr-MOF-on-Ti-MOF as shown in Fig. 20B. The photogenerated electrons on the CB of NH2-MIL-125 spontaneously combine electrostatically with holes in the VB of g-C3N4 and UiO-66. The CB potentials of g-C3N4 (−1.08 V) and UiO-66 (−0.6 V) are more negative than the O2/˙O2− potential (−0.33 V vs. NHE), enabling the photogenerated electrons to reduce O2 to ˙O2−. Additionally, as the VB of both NH2-MIL-125 and UiO-66 is more positive than H2O/˙OH (1.99 V vs. NHE), the photogenerated holes in the VBs of NH2-MIL-125 and UiO-66 (3.17 V and 3.22 V) can activate water molecules to form ˙OH. Eventually, these ROS (˙O2− and ˙OH) can effectively degrade and mineralize the ofloxacin (Fig. 20C). The degradation rate of ofloxacin is approximately 1.79 times higher than that of the original UiO-66-on-MIL-125. Zhang et al. developed a nanoreactor composed of iron-doped carbon dots (Fe-CDs) and MOF-808.195 Fe-CDs/MOF-808 and Fe-CDs@MOF-808 were synthesized for cascade degradation and the detection of paraoxon and parathion, respectively.
In typical advanced oxidation processes (AOPs), hydrogen peroxide (H2O2) is used to generate hydroxyl radicals (˙OH).286 However, the instability of hydroxyl radicals limits their application. Recently, sulfate radicals (SO4˙−, SR), which have a longer half-life than ˙OH, have gained increasing attention and solid persulfate (Na2S2O8, PS) is more convenient for storage and transportation compared with liquid H2O2.287 Combining AOPs with photocatalysis and using materials containing metals such as iron, cobalt and manganese as photocatalysts is considered an ideal method to enhance degradation efficiency. Zhou et al. synthesized an Fe-based MOF MIL-88A (ligand = fumaric acid) via hydrothermal methods,288 which, when combined with sulfate-based advanced oxidation processes (SR-AOPs), effectively degrades tetracycline hydrochloride under visible light. The integration of photocatalysis with SR-AOPs significantly enhances the degradation capacity of tetracycline. Scavenging experiments and ESR analysis indicate that SO4˙− and ˙O2− radicals are the primary radicals responsible for the degradation of tetracycline. Similarly, Li et al. showed that sulfonamide antibiotics in water are effectively removed through the coupling of photocatalysis and persulfate advanced oxidation technology using Fe-doped UiO-66.289
Fenton technology is a widely used advanced oxidation process in wastewater purification. Using heterogeneous catalysts and combining photocatalysis with the Fenton method not only avoids the issues of traditional technology but also achieves efficient degradation. The photo-Fenton technique develops a fast electron transfer channel, enhancing the swift breakdown of H2O2 to produce reactive oxygen species, ensuring the efficiency of pollutant removal.290,291 Wang et al. designed a self-assembled CuS/MIL-Fe catalyst to effectively degrade Acetaminophen (APAP) through the photo-Fenton reaction (Fig. 20D).292 Using UV–vis and Tauc curve analyses, the band gaps (Eg) of CuS, MIL-101(Fe) and CuS/MIL-Fe were determined to be 1.80, 2.71 and 2.18 eV, respectively. MIL-101(Fe) and CuS are typical n-type and p-type semiconductors, respectively. When combined, these two types of semiconductor form a p–n heterojunction, improving the APAP degradation effectively. R Geetha Balakrishna et al. have developed a low-cost and simple method to enhance the performance and stability of Fe-MOFs through amine (NH2) functionalization and in situ integration of metal oxides.293 The dual photoexcitation pathway induced by NH2 functional groups facilitates electron transfer to the Fe center, directly exciting Fe-o clusters (Fe3+ to Fe2+), endowing the system with multifunctionality. The NH2-MIL-101(Fe) and α-Fe2O3 composite materials obtained were subsequently embedded into PAN and electrospun nanofibers, used for enhancing the self-cleaning properties of the membranes and the simultaneous separation and photocatalytic degradation of various pollutants. This heterostructure composed of metal oxides and MOF composites exhibits excellent photocatalytic activity, effectively facilitating carrier separation. Integrating the composite materials into nanofiber membranes for water treatment achieved an overall removal rate of 94%, with 20% of the MB being degraded simultaneously, making it a valuable reference for emerging processes that involve simultaneous degradation and separation. The summary of different MOF-based photocatalysts for pollutant degradation was shown in Table 1.
Table 1 The summary of different MOF-based photocatalysts for pollutant degradation
MOF |
Pollutant |
C
Pollutant (mg L−1) |
C
MOF (mg L−1) |
ROS |
Time (min) |
Efficiency (%) |
K (min−1) |
Ref. |
PDI refers to pyromellitic diimide. PET refers to polyethylene terephthalate. PDA refers to polydopamine. NS refers to nanosphere. Psf refers to polysulfone. PAN refers to polyacrylonitrile. MOX refers to metal–organic xerogel. P21 refers to PCN-134 and PCN-222. VNU refers to Vietnam National University. N.R = Not reported. |
MIL-88A(Fe) |
Tetracycline hydrochloride |
100 |
250 |
SO4−, ˙O2− |
90 |
100 |
0.0216 |
288
|
g-C3N4/PDI@NH2-MIL-53(Fe) |
Carbamazepine |
50 |
400 |
˙OH |
150 |
78 |
N.R |
294
|
PET@PDA@NH2-MIL-53(Fe) |
Phenol |
20 |
N.R |
˙OH, h+ |
60 |
98 |
0.0519 |
295
|
g-C3N4/PDI@NH2-MIL-53(Fe) |
Tetracycline |
50 |
400 |
˙OH |
60 |
90 |
N.R |
294
|
CuS/MIL-101(Fe) |
Acetaminophen |
5 |
200 |
˙OH, 1O2 |
30 |
99.8 |
0.2099 |
292
|
MIL-100(Fe)@ZnO NS |
Phenol |
5 |
200 |
˙OH |
120 |
92 |
0.0339 |
296
|
g-C3N4/PDI@NH2-MIL-53(Fe) |
p-Nitrophenol |
50 |
400 |
˙OH |
30 |
100 |
N.R |
294
|
MIL-101(Fe)/g-C3N4 |
Cr(VI) |
20 |
50 |
˙O2−, h+ |
60 |
92.6 |
0.0432 |
297
|
PSf/MIL-101(Fe) |
Methyl orange |
10 |
N.R |
˙OH, ˙O2− |
120 |
71.03 |
N.R |
298
|
rPAN@ (NH2-MIL-101(Fe) + α –Fe2O3) |
Methylene blue |
10 |
200 |
˙OH, ˙O2−, h+ |
120 |
93.63 |
N.R |
293
|
P21-X(PCN-134,PCN-222)(Zr) |
Nizatidine |
40 |
100 |
1O2 |
120 |
90.81 |
0.01345 |
278
|
VNU-1(Zr) |
Sulfamethoxazole (80 ppb) |
0.08 |
100 |
˙O2−, h+ |
5 |
100 |
0.478 |
273
|
UiO-66/NH2-MIL-125/g-C3N4 |
Ofloxacin |
20 |
100 |
˙OH, ˙O2− |
50 |
>99.1 |
0.07 |
285
|
NH2-MIL-53(Al)/CdS |
Cr(VI) |
10 |
400 |
h+, ˙OH |
180 |
99.23 |
0.0276 |
124
|
Ag-MOF |
Methyl orange |
10 |
50 mg |
O2−, h+ |
120 |
74.5 |
0.0123 |
299
|
3.2. Carbon dioxide (CO2) reduction
The excessive use of fossil fuels has led to the accelerated emission of carbon dioxide, becoming a key factor in adverse climate and environmental changes.300,301 Converting CO2 into useful chemicals (such as CO, HCOOH, CH3OH and C2H4) is imperative.302 Despite significant efforts and progress over the past decade, challenges in reaction activity and product selectivity persist in this research field.303 Photocatalytic methods can efficiently utilize solar energy while also reducing the concentration of CO2 in the air.304–311 The porous structure of MOF-based photocatalysts stands out among many materials by providing more reaction sites and abundant CO2 capture sites during the catalysis process.312–315 MOF photocatalysts need a LUMO level higher than the redox potential of the CO2 reduction half-reaction, which depends on the potential of various products. For example, the redox potentials for CO2 reduction to CO, formic acid, methanol and methane are 3.50, 3.42, 3.65 and 3.79 eV respectively (Fig. 21A).316 Bai et al. used a 2D Ni-based MOF NMF ([Ni(phen)(oba)]n·0.5nH2O, phen = 1,10-phenanthroline, oba = 4,4′-oxybis(benzoate)) composite with all-inorganic perovskite CsPbBr3; when the aspect ratio of CsPbBr3 nanocubes, nanorods and nanowires increases, the energy difference (ΔE) at the CB of CsPbBr3 and the NMF interface increases, promoting the transfer of photoelectrons from CsPbBr3 to NMF and enhancing the photocatalytic activity for CO2-to-CO conversion.317
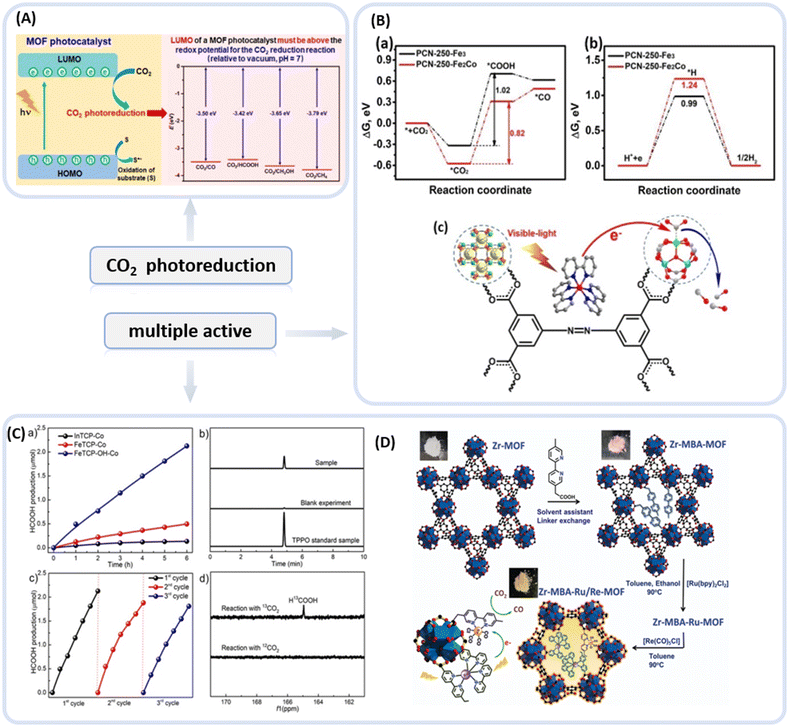 |
| Fig. 21 (A) The process of photocatalytic CO2 reduction and the redox potentials for the reduction of CO2 into CO, HCOOH, CH3OH and CH4 from ref. 316. Copyright 2020, Elsevier. (B) (a and b) Free energy profile of CO2RR toward the production of CO and free energy diagram of HER for PCN 250-Fe3 and PCN-250-Fe2Co, respectively; (c) photocatalytic routes for CO2 reduction from ref. 319. Copyright 2020, Royal Society of Chemistry. (C) (a) Time-dependent HCOOH evolution with InTCP-Co, FeTCP-Co and FeTCP-OH-Co as catalysts respectively, (b) detection of H2O2 by HPLC after CO2, (c) the HCOOH yield in 3 cycles, and (d) the 13C NMR spectra of reaction solutions with 13CO2/12CO2 and over FeTCP-OH-Co from ref. 320. Copyright 2021, John Wiley and Sons. (D) The construction of Zr-MBA-Ru/Re-MOF via post-synthetic linker exchange and immobilization of the molecular photosensitizer and catalyst toward CO2 reduction from ref. 321. Copyright 2021, Royal Society of Chemistry. | |
In most cases, rare metals such as Ru, Ir and Co are key components of photocatalysts, including photosensitizers and catalysts. Constructing CO2 photoreduction systems using abundant and non-toxic elements on Earth aligns with the principles of sustainable chemistry. Daisuke Tanaka et al. reported a type of SnII-based MOF [SnII2(H3ttc)2·MeOH]n(KGF-10; H3ttc = trithiocyanuric acid, MeOH = methanol) that converts CO2 into formate ion (HCOO−) under visible light.318 This represents the first example of using Sn-MOF to drive CO2 reduction with visible light. Even in the absence of precious-metal cocatalysts, KGF-10 catalyzes the conversion of CO2 to HCOO− with high selectivity (>99%) and an AQY of 9.8% at 400 nm.
Feng et al. enhanced CO2 conversion activity by varying the types of metal in MOF cluster nodes, achieving the highest-performing MOF photocatalyst for CO2 conversion.319 PCN-250-Fe3 (ligand = 3,3′,5,5′-azo-benzene tetra-carboxylic acid (H4abtc)) was made with FeIII2FeII metal-cluster nodes and open metal sites. Varying the type of MII metal ions in the PCN-250-Fe2M (M = Mn, Zn, Ni, Co) clusters exhibited better catalytic activity and selectivity for CO2 conversion. Among them, PCN-250-Fe2Mn achieved the highest photocatalytic activity under visible light, reaching 21.51 mmol h−1 g−1, making it the most effective MOF-based CO2 conversion photocatalyst under similar reaction conditions to date. DFT was utilized to further analyze the specific effects of the bimetallic PCN-250-Fe2Co on CO2 reduction performance (Fig. 21B). Introducing the second MII metal ion enhances the CO2 reduction pathway, inhibiting the formation of H2 evolution intermediates. This promotes the migration of photogenerated electrons to active sites and improves the adsorption and activation of CO2.
The preparation of hydroxylated MOFs with multiple active centers as catalysts achieved high efficiency and selectivity in artificial photosynthesis. Lin et al., by modifying the types of metal on the nodes and introducing excess hydroxyl groups on the ligands, prepared hydroxylated and heterometallic MOFs.320 Artificial photosynthesis was achieved without the addition of sacrificial agents and photosensitizers. Acid–base resistant frameworks of (In/Fe)TCP-Co (ligand = 5,10,15,20-tetrakis(3,4-dihydroxyphenyl)porphyrin-Co (3,4-TDHPP-Co)) and (In/Fe)TCP-OH-Co (ligand = 5,10,15,20-tetrakis(2,3,4-trihydroxyphenyl)porphyrin-Co (2,3,4-TTHPP-Co)) based on catechol-metalloporphyrin were prepared. The HCOOH evolution rate of FeTCP-Co far exceeded that of InTCP-Co by 3.7 times. The iron-oxo chain in FeTCP-Co is more favorable than the indium-oxo chain in InTCP-Co for photoinduced electron–hole separation, according to the experimental and DFT calculations findings. The reactivity of CO2 to HCOOH conversion was further enhanced by incorporating uncoordinated -OH into the (In/Fe)TCP-Co framework. Their HCOOH yields were respectively 5.6 and 4.3 times those of uncoordinated non-hydroxylated compounds. FeTCP-OH-Co achieved a selectivity for HCOOH of 97.8%. Ion chromatography (IC), gas chromatography (GC) and high-performance liquid chromatography (HPLC) were used to detect the reduced products HCOOH, trace CO and oxidized product H2O2, respectively (Fig. 21C(a and b)). The results showed that under the same conditions, FeTCP-Co had the highest photocatalytic activity, with the photocatalytic efficiency increasing in turn from InTCP-Co, FeTCP-Co and InTCP-OH-Co to FeTCP-OH-Co. Repeated experiments also demonstrated the good stability of the catalysts (Fig. 21C(c)). To validate the carbon source of the evolved products, isotope-labeling experiments were checked (Fig. 21C(d)). The enhancement in performance was due to the synergistic effects of strong visible light absorption, suitable band structure, ideal CO2 affinity and multiple evenly distributed redox active sites.
Molecular catalytic active sites, including photosensitizers, can also be grafted onto MOFs through post-synthetic modification (PSM), expanding the optical absorption range and enhancing the catalytic activity. Here, Tapas Kumar Maji et al. constructed a multifunctional Zr-MBA-Ru/Re-MOF (MBA = A [2-(50-methyl-[2,20-bipyridine]-5-yl)acetic acid])assembly using post-synthetic ligand exchange (PSE) and PSM.321 MOF-808 (Zr-MOF, ligand = H3BTC) was selected as the host due to its enclosed cage structure and robustness in water, first, formates were exchanged with MBA ligands through PSE to enter the chelating sites within the MOF unit. MBA was covalently grafted onto Zr-MOF, utilizing the N,N′ chelating center of the MBA ligands to chelate with [Ru(bpy)2]2+, and subsequently metalated with [Re(CO)5Cl] to obtain Zr-MBA-Ru/Re-MOF. The synthesized Zr-MBA-Ru/Re-MOF exhibited effective CO2 reduction without requiring other sacrificial electron donors, and the selectivity for CO was >99%, with a maximum yield of 440 μmol g−1 h−1. Additionally, using BNAH and TEOA as sacrificial electron donors, the selectivity for CO was 69%, with a maximum rate of 180 μmol g−1 h−1. To simulate natural photosynthesis, the catalytic activity under direct sunlight was studied in water, producing 210 μmol g−1 of CO within 6 hours (Fig. 21D).
The summary of different MOF-based photocatalysts for CO2 reduction is shown in Table 2.
Table 2 The summary of different MOF-based photocatalysts for CO2 reduction
MOF |
Production |
Sacrificial electron donor |
Reduction product |
Selectivity (%) |
Ref. |
MBA refers to 2-(50-methyl-[2,20-bipyridine]-5-yl)acetic acid. BNAH refers to 1-benzyl-1,4-dihydronicotinamide. TEOA refers to triethanolamine. TIPA refers to tri-isopropanolamine. Fc refers to ferrocene carboxylic acid. MOL refers to metal–organic layers. HHTP refers to 2,3,6,7,10,11-hexahydroxytriphenylene. BVO refers to BiVO4. TCPP refers to tetra(4-carboxyphenyl)porphyrin. BTC refers to 1,3,5-benzene tricarboxylate. CM refers to copper mesh. KGF-10 refers to H3ttc = trithiocyanuric acid, MeOH = methanol. BIH refers to 1,3-dimethyl-2-phenyl-2,3-dihydro-1H-benzo[d]imidazole. SAs refers to single-atom catalysts. N.R = not reported. |
Zr-MBARu/Re-MOF |
CO |
BNAH, TEOA |
180 μmol g−1 h−1 |
69 |
321
|
PCN-250-Fe2Mn |
CO |
TIPA |
21.51 μmol g−1 h−1 |
82.17 |
319
|
PCN-250-Fe2Zn |
CO |
TIPA |
19.45 μmol g−1 h−1 |
82.30 |
319
|
PCN-250-Fe2Ni |
CO |
TIPA |
15.86 μmol g−1 h−1 |
81.84 |
319
|
PCN-250-Fe2Co |
CO |
TIPA |
14.01 μmol g−1 h−1 |
83.53 |
319
|
Ni-MOF(H2O) |
CO |
TEOA |
9.61 mmol h−1 g−1 |
95.24 |
322
|
Ni-MOL-100 |
CO |
TEOA |
569.00 mmol g−1/18 h |
100 |
323
|
Co-MOF/Cu2O |
CO |
None |
3.83 μmol g−1 h−1 |
∼100 |
324
|
SiW12@CdMOF |
CO |
TEOA |
4.35 mmolCO molCd−1 h−1 |
∼99 |
325
|
PW12@CdMOF |
CO |
TEOA |
3.6 mmolCO molCd−1 h−1 |
∼99 |
325
|
NH2-UiO-66-Fc |
CO |
TEOA |
90.65 μmol g−1 h−1 |
100 |
326
|
Fe-soc-O |
CO |
TIPA |
1804 μmol g−1 h−1 |
85.6 |
327
|
Fe-soc-M |
CO |
TIPA |
1594 μmol g−1 h−1 |
90.7 |
327
|
Fe-soc-C |
CO |
TIPA |
1550 μmol g−1 h−1 |
94.7 |
327
|
Ru@Cu-HHTP |
CO |
TEOA |
156 mmol gcat−1 h−1 |
92.9 |
328
|
FeSx@ZnS@CoSx(MIL-88A@ZIF-8@ZIF-67) |
CO/CH4 |
(Water vapor) |
69.90 /2.01 μmol g−1 h−1 |
N.R |
329
|
Ag/NH2-MIL125(Ti) (T3-Ag) |
CO/CH4 |
TEOA |
26.7/63.3 μmol g−1 h−1 |
90.5 (CH4) |
330
|
Ni@MOF/BVO(NTU-9) |
CO/CH4 |
TEOA |
44.5/0.625 μmol g−1 h−1 |
99.2 |
330
|
Cu-ZnTCPP/g-C3N4 |
CO/CH4 |
N.R |
92 /11.3 μmol g−1 h−1 |
N.R |
331
|
CuTCPP & UiO-66/TiO2 |
CO/CH4 |
N.R |
31.32 μmol g−1 h−1/0.148 μmol g−1 h−1 |
N.R |
332
|
Cu2O@Cu3(BTC)2/CM(copper mesh) |
CH4 |
N.R |
0.73 mmol/8 h |
N.R |
333
|
FeTCP-OH-Co |
HCOOH/CO |
None |
17.72/0.396 μmol g−1 h−1 |
97.8 |
320
|
FeTCP-Co |
HCOOH/CO |
None |
4.14/0.088 μmol g−1 h−1 |
N.R |
320
|
InTCP-Co |
HCOOH/CO |
None |
1.125/0.041 μmol g−1 h−1 |
N.R |
320
|
KGF-10 |
HCOOH |
BIH |
59 μmol, 4 mg, 5 h |
>99 |
318
|
PCN-136(Zr) |
HCOO− |
TIPA |
0.294 μmol g−1 h−1 |
N.R |
334
|
Cu SAs/UiO-66-NH2 |
Methanol/ethanol |
N.R |
5.33/4.22 μmol h−1 g−1 |
N.R |
335
|
3.3. Nitrogen (N2) fixation
Nitrogen is essential for life, constituting important components of amino acids, nucleotides and other essential biomolecules. Nitrogen fixation stands as one of the most important natural processes in the Earth's nitrogen cycle, converting atmospheric N2 into nitrogen-containing compounds accessible to organisms, including humans.336–340 Although nitrogen gas constitutes 78 vol% of the Earth's atmosphere, its high electron affinity and large activation energy barrier (941 kJ mol−1) prevent direct utilization. Effective storage and conversion into nitrogen-containing compounds, namely ammonia (NH3) and nitrate (HNO3), are crucial for promoting the nitrogen economy.341–345
Currently, the most extensively studied system is the Mo-dependent nitrogenase from the nitrogen-fixing bacterium Azotobacter vinelandii, consisting primarily of Fe protein and MoFe protein, where the MoFe protein contains two metal clusters (P-cluster and M-cluster).346,347 But relying solely on naturally occurring fixed nitrogen (primarily ammonia) generated through natural cycles is insufficient to meet the needs of industrial production and human life. The Haber–Bosch (HB) process, as one of the most significant inventions of the 20th century, greatly increased crop yields and the global economy. Until now, this remains the sole method for industrial ammonia production. The key chemical reaction of HB process can be expressed by the reaction N2 (g) + 3H2 (g) → NH3 (g).348–350 Each step of the reaction requires high temperatures and pressures (673–873 K, 15–25 MPa),351,352 resulting in significant energy consumption and greenhouse gas emissions.
Photocatalytic nitrogen fixation offers environmental friendliness and energy efficiency, making it an ideal approach in the context of carbon neutrality and environmental protection.353,354 Up to now, extensive research has been conducted to develop efficient heterogeneous photocatalysts for nitrogen fixation, ranging from inorganic to organic semiconductors, such as Fe2O3, CdS, ZnO, BiOBr and g-C3N4.355,356 However, these catalysts similarly suffer from issues such as low efficiency. The kinetic diameter of N2 (3.64 Å) is smaller than the pore sizes of multi-level porous MOFs (most of which are >4 Å), and MOFs possess an ultra-high surface area of up to 7000 m2 g−1, giving us reason to believe that MOFs can efficiently adsorb and store N2.357–361
This is the first example of N2 fixation driven by visible light using MOF materials. Ye et al. first reported a series of targeted visible-light-active MOF catalysts, namely CH3-MIL-125 (Ti), OH-MIL-125 (Ti) and NH2-MIL-125 (Ti), with visible-light-assisted photocatalytic N2 fixation Fig. 22A(a).362 The structural schematic of NH2-MIL-125 (Ti) is shown in Fig. 22A(b). Its 3D pseudocubic structure is composed of cyclic octameric inorganic subunits of edge and corner-sharing TiO5(OH) octahedra, connected by aminoterephthalate ligands. MOFs with different functional groups but the same structure demonstrated effective photocatalytic N2 fixation under visible light, thanks to the interaction between the Ti nodes and functionalized organic linkers. The results indicate that NH2-MIL-125 (Ti) exhibits the best light absorption performance at 550 nm, with the highest ammonia evolution rate of 12.3 μmol g−1 h−1. The Ti3+ sites generated by LMCT electron transfer act as active sites, with ligand functionalization extending the MOF light harvesting to the visible spectrum, facilitating visible-light-assisted photocatalytic N2 fixation.
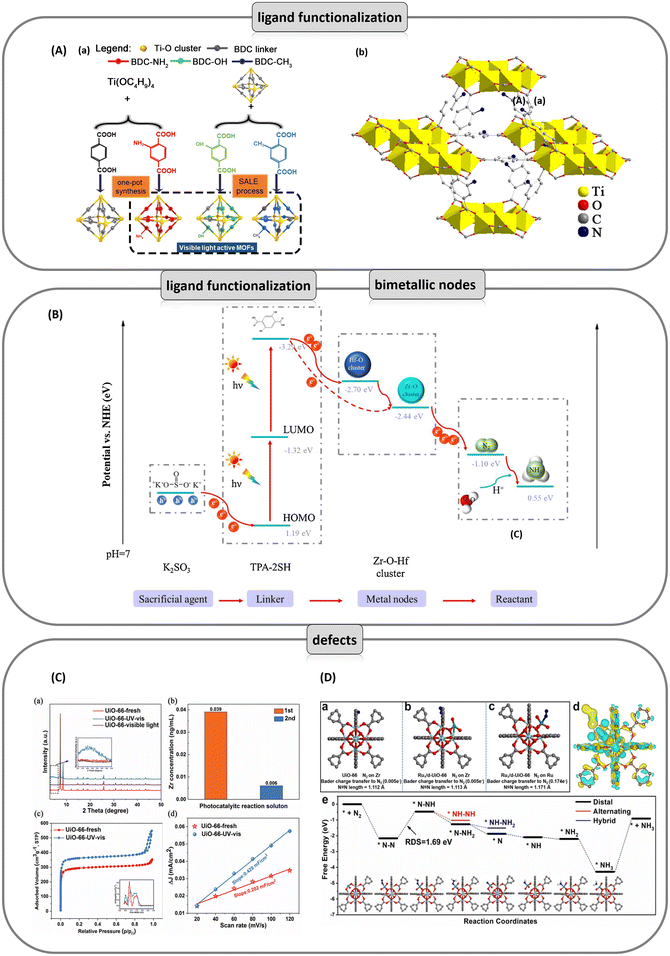 |
| Fig. 22 (A) (a) Schematic diagram of the targeted visible light active MOFs for N2 fixation, (b) the structure of NH2-MIL-125 (Ti) from ref. 362. Copyright 2020, Elsevier. (B) A proposed mechanism for a U(0.5Hf)-2SH sample in nitrogen reduction from ref. 363. Copyright 2021, Elsevier. (C) (a) XRD patterns of UiO-66 before and after UV-Vis light and visible light irradiation, (b) the concentrations of Zr in the first and second photocatalytic solutions determined by ICP-MS, (c) N2 adsorption–desorption isotherms for UiO-66-fresh and UiO-66-UV-vis, and (d) plot of the scan rates against the differences in the double-layer charging current of UiO-66-fresh and UiO-66-UV-vis from ref. 364. Copyright 2021, Royal Society of Chemistry. (D) (a–c) Structures of N2 adsorbed on different sites of catalysts, (d) difference charge density of Ru1/d-UiO-66 with the adsorption of N2, (e) free energy diagrams for N2 reduction on Ru1/d-UiO-66 through distal and alternating mechanisms with corresponding reaction energy from ref. 365. Copyright 2023, Wiley-VCH GmbH. | |
Inspired by nitrogenase, Jiang et al. designed a series of modular UIO-66-type metal–organic frameworks, consisting of bimetallic Zr–Hf nodes and MOF photocatalysts U(Zr–Hf)-X with various functional ligands.363 In the Zr–Hf bimetallic clusters, the elements zirconium (Zr) and hafnium (Hf) selectively adsorb nitrogen. Due to the potential difference between the Zr–O and Hf–O clusters, there was an MMET pathway from Hf species to Zr species, acting as an electron buffer optimizing electron transfer, and Zr species served as the catalytically active site, synergizing electron movement and utilization in photocatalysis. In the organic ligands, introducing targeted functional groups effectively generates electrons, enhancing the MOFs’ light absorption and utilization, with organic ligands containing two thiol (-SH) groups extending the absorption edge to the visible light region. The nitrogen fixation rate of the photocatalyst reaches up to 116.1 μmol g−1 h−1 under visible light, which is attributed to the LMMET pathway and Zr-based π-backbonding mechanism. By independently manipulating the metal nodes and organic ligands, this nitrogenase-inspired MOF material strategy paves a new pathway for designing dual synergistic catalysts for chemical, photochemical and biochemical conversions in energy and environmental fields (Fig. 22B).
Defects can capture electrons and then inject these electrons into antibonding π-orbitals, thereby weakening and activating the adsorbed N2. Li et al. reported the first instance of UiO-66 featuring photoexcited cluster defects and linked defects for photocatalytic nitrogen fixation.364 Linker defect-formed unsaturated metal nodes exhibited greater contribution to photocatalytic nitrogen fixation than cluster defects, which defined as the PSE process. Notably, in an air atmosphere and pure water without a sacrificial agent, the full-wavelength performance increased from 131 to 196 μmol h−1 g−1 compared with fresh UiO-66. UiO-66 was synthesized using a straightforward solvothermal method and various tests confirmed the presence of linker defects. Following UV-Vis irradiation, UiO-66 exhibited an increased Brunauer–Emmett–Teller (BET) specific surface area and pore volume. These results are attributed to the formation of defects, as demonstrated by the impact of illumination on the material electrochemical performance through electrochemical surface area (ECSA) measurements (Fig. 22C(a–c)). As shown in Fig. 22C(d), the Cdl of UiO-66-UV-vis (0.428 mF cm−2) is higher than the UiO-66-fresh Cdl (0.202 mF cm−2), indicating a higher ECSA for UiO-66-UV-vis. The larger BET surface area and ECSA provide more active sites, which is beneficial for enhancing the performance of photocatalytic nitrogen fixation. This paper presents a novel and effective strategy for designing high-performance nitrogen-fixing photocatalysts.
Similarly, Meng et al. proposed a photochemical strategy for creating defects,365 further depositing Ru single atoms on the UiO-66 (Zr) framework. After treatment with nitrogen gas and ultraviolet light, UiO-66 was centrifuged, washed, and vacuum-dried to yield defect-laden UiO-66 (d-UiO-66). Covalent bonding between the Ru single atoms and the support establishes electron-metal–support interactions (EMSI). EMSI facilitates accelerated charge transfer between Ru SAs and UiO-66, conducive to enhanced photocatalytic activity. After introducing defects to UiO-66, the photocatalytic yield of ammonia increased from 4.57 μmol g−1 h−1 to 16.28 μmol g−1 h−1, and following the loading of Ru SAs, it further rose to 53.28 μmol g−1 h−1. DFT calculations were employed to investigate the strong EMSI effect in Ru1/d-UiO-66 and further elucidate the impact of Ru single-atom anchoring on UiO-66 for local electronic structure tuning and NRR pathways. For the pristine UiO-66, N2 molecules are terminally adsorbed at Zr sites with a N–N bond length of 1.112 Å, closely approximating the bond length of free N2 molecules at 1.11 Å. Bader charge analysis shows that a transfer of 0.05 e− to N2 molecules makes their activation challenging, indicating the difficulty of NH3 formation on the pristine UiO-66 surface (Fig. 22D(a)). Subsequently, the adsorption results of N2 at different sites in Ru1/d-UiO-66 indicate a preference for adsorption at Ru sites on UiO-66 nodes. Bader charge analysis indicates that the 0.174 e− transferred to N2 molecules at Ru sites is 35 times that transferred at Zr sites (Fig. 22D(b and c)). Due to EMSI, electron mobility within active sites enhances the activity of single atom metal centers. Concurrently, the N–N bond length extends from 1.112 to 1.171 Å, showing an efficient activation of N2 molecules. Charge density differential analysis also reveals the formation of strong EMSI at the active sites of Ru1/d-UiO-66. Fig. 22D(d) showed visible charge accumulation around Ru, further evidencing the modulation of the electronic structure of the metal center following the introduction of Ru single atoms. Fig. 22D(e) displayed the free energy diagram for the reduction of N2 on Ru1/d-UiO-66 through a distal and corresponding reaction energy alternating mechanism. The summary of different MOF-based photocatalysts for N2 reduction is shown in Table 3.
Table 3 The summary of different MOF-based photocatalysts for N2 reduction
MOF |
Light source |
Raw materials |
Nitrogen fixation activity |
Scavenger |
Ref. |
abtc refers to 3,3′,5,5′-azomellitic acid (H4abtc). PSE refers to post-synthetic ligand exchange process. DFC3N4 refers to defected film C3N4. N.R = Not reported. |
NH2-MIL-125 (Ti) |
Xenon lamp (300 w) λ ≥400 nm |
N2/H2O |
12.3 μmol g−1 h−1 |
None |
362
|
OH-MIL-125 (Ti) |
Xenon lamp (300 w) λ ≥400 nm |
N2/H2O |
5.04 μmol g−1 h−1 |
None |
362
|
CH3-MIL-125 (Ti) |
Xenon lamp (300 w) λ ≥400 nm |
N2/H2O |
1.39 μmol g−1 h−1 |
None |
362
|
MIL-53(FeII/FeIII) |
Xenon lamp (300 w) λ ≥420 nm |
N2/H2O |
306 μmol g−1 h−1 |
K2SO3 |
366
|
4U(0.5Hf)-2SH (UiO-66) |
Xenon lamp (300 w) |
N2/ultrapure water |
116.1 μmol g−1 h−1 |
K2SO3 |
363
|
Fe-abtc |
Xenon lamp (300 w) |
N2/H2O |
49.8 μmol g−1 h−1 |
K2SO3 |
367
|
Zr-abtc |
Xenon lamp (300 w) |
N2/H2O |
35.7 μmol g−1 h−1 |
K2SO3 |
367
|
MIL-125(Ti)-250 (250 °C) |
Xenon lamp (300 w) |
N2/H2O |
156.9 μmol g−1 h−1 |
N.R |
368
|
Ru-MOF-74(Zn) |
Xenon lamp (300 w) λ >400 nm |
N2/H2O |
70.9 μmol g−1 h−1 |
None |
369
|
UiO-66-PSE |
Xenon lamp (300 w) |
N2/ultrapure water |
196 μmol g−1 h−1 |
None |
364
|
ZCS(ZnCdS)@CAU-100(Bi) |
Xenon lamp (300 w) |
N2/ultrapure water |
46.96 μmol g−1 h−1 |
None |
370
|
CAU-17@Bi2S3 |
Xenon lamp (300 w) |
N2/ultrapure water |
14.89 μmol g−1 h−1 |
None |
370
|
UiO-66(SH)2-200(200 °C) |
Xenon lamp (300 w) |
N2/H2O |
32.4 μmol g−1 h−1 |
None |
371
|
Ru1/d-UiO-66 |
Xenon lamp (300 w) |
N2/H2O |
53.28 μmol g−1 h−1 |
N.R |
365
|
CeZr5-UiO-66 |
Xenon lamp (300 w) |
N2/ultrapure water |
200.13 mmol g−1 h−1 |
N.R |
372
|
ZIF-67@PMo4V8 |
Xenon lamp (300 w) |
N2/H2O/ethanol |
149.0 μmol L−1 h−1 |
N.R |
373
|
ZIF-67@PMo112 |
Xenon lamp (300 w) |
N2/H2O/ethanol |
39.4 μmol L−1 h−1 |
N.R |
373
|
ZIF-67@PMo11V |
Xenon lamp (300 w) |
N2/H2O/ethanol |
70.0 μmol L−1 h−1 |
N.R |
373
|
ZIF-67@PMo10V2 |
Xenon lamp (300 w) |
N2/H2O/ethanol |
74.8 μmol L−1 h−1 |
N.R |
373
|
ZIF67@PMo9V3 |
Xenon lamp (300 w) |
N2/H2O/ethanol |
134.6 μmol L−1 h−1 |
N.R |
373
|
MOF-74(Zn)@DFC3N4 |
Xenon lamp (300 w) |
N2/H2O/methanol |
2.32 mmol g−1 h−1 |
Methanol |
374
|
3.4. Hydrogen (H2) production
The depletion of traditional fossil fuel resources has generated a substantial demand, prompting the exploration of sustainable fuels. In this context, hydrogen is regarded as the most promising emerging fuel for the future due to its zero carbon emissions and high energy density (142 MJ kg−1).375–378 Hydrogen energy is considered a green and renewable alternative to traditional energy sources. However, H2 production has been somewhat limited due to its high production costs.379 The hydrogen evolution reaction (HER) is significant in the field of photocatalysis as it converts solar energy into hydrogen. To enhance the efficiency of HER, researchers are developing efficient photocatalysts such as those based on MOFs and their composites.380 Due to their large porosity, which provides a substantial surface area and numerous active sites, MOFs have tremendous potential in hydrogen production. Currently, an increasing number of MOF-based photocatalysts are being used for hydrogen production.381–383 In 2009, the first instance of an MOF being used as an active site for photocatalytic water reduction to hydrogen molecules was reported by Wasuke Mori et al.384
Considering the remarkable efficiency of platinum photocatalysts in hydrogen evolution from water, growing research has diverted its focus towards investigating Pt and other noble metal catalysts.385 Lai et al. synthesized ultrathin Zr-TCPP(Pd) nanosheets.386 Uniform anchoring of Pt nanoparticles onto smaller nanoparticles enables the synthesis of 2% Pt/Zr-TCPP (Pd) hybrid nanosheets with a homogeneous distribution. Under visible light irradiation, the hydrogen production rate of 2% Pt/Zr-TCPP(Pd) reaches 3348 μmol h−1 g−1. The AQE of 2% Pt/Zr-TCPP(Pd) at 420 nm is approximately 1.56%. This outstanding photocatalytic H2 production performance is due to the ultrathin nanosheets’ ability to rapidly transfer long-lived electrons from pd-porphyrin photosensitizers to PtNPs, obviously enhancing the efficiency of photogenerated electron–hole separation.
In the early stages, noble metal co-catalysts (Pt, Pd, Au) were used to enhance photocatalytic hydrogen evolution; however, the scarcity and high cost of these metals limit the HER process. Therefore, developing high-density active sites and high-efficiency non-noble metal photocatalysts remains a significant challenge. In recent years, research on non-noble metals has increasingly grown.387 Huang et al. prepared a conductive MOF-based catalyst,388 Ni3(HITP)2/TiO2 (ligand = 2,3,6,7,10,11-hexaaminotriphenylene (HATP)), whose corresponding HER demonstrated a significant enhancement in photocatalytic performance when TiO2 was combined with Ni3(HITP)2. Systematic photoelectrochemical experiments indicated that Ni3(HITP)2 effectively facilitated charge transfer and reduced charge recombination. Furthermore, using the Ni-N4 active sites in Ni3(HITP)2 as the reaction centers, it successfully achieved noble-metal-free photocatalysis without relying on the typical co-catalyst Pt. This provides a new direction for subsequent MOFs in the field of non-noble metal photocatalytic production of H2.
Moreover, Shi et al. synthesized a series of trimetallic MOF-based photocatalysts containing uniformly dispersed photosensitive Cd-NS and photothermal Ni-NS single-sites at a molecular level.389 By balancing the photosensitivity and photothermal effect, the optimized Ho6-Cd0.76Ni0.24-NS (ligand = 3-fluoropyridine-4-carboxylic acid (HFNA)) exhibited an outstanding hydrogen evolution rate of 40.06 mmol g−1 h−1, with an AQE of 29.37% at 420 nm. Owing to the ultrashort distance from the uniformly dispersed Cd-NS single-sites to Ni-NS single-sites, combined with the photothermal effect, the transport of photoinduced electrons for proton reduction was greatly enhanced.
To investigate the impact of electrons on photocatalytic activity, Zhang et al. proposed a scheme to modulate the rate of electron transfer and the corresponding photocatalytic HER activity by adjusting the size of CdS quantum dots (QDs).390 Using a partial sulfidation strategy, they constructed a series of sensitized semiconductors composed of monodisperse CdS QDs with controlled sizes and cadmium tetra(4-carboxyphenyl)porphyrin (Cd-TCPP) nanosheets. By controlling the size of the CdS to adjust the bandgap, as shown in Fig. 23A, it was observed that as the average particle diameter increased from 3.3 nm to 8.1 nm, the bandgap of the CdS decreased from 2.72 eV to 2.40 eV. Larger CdS quantum dots exhibited lower conduction band positions, as shown in Fig. 23B. CdS/Cd-TCPP composites with larger CdS quantum dot sizes are expected to exhibit stronger photoresponsivity. A variety of experiments revealed that CdS/Cd-TCPP composites with medium-sized CdS QDs and optimal conduction band positions achieved the highest photocatalytic activity, with HER activity nearly five times that of pure CdS. This may be because larger CdS quantum dots facilitate electron injection from Cd-TCPP to CdS, although their synthesis consumes more Cd centers in the Cd-TCPP substrate during the sulfidation process. Thus, medium-sized CdS quantum dots exhibited the greatest photoresponse and photocatalytic activity. Fig. 23C illustrates the electron transfer process within its heterostructure.
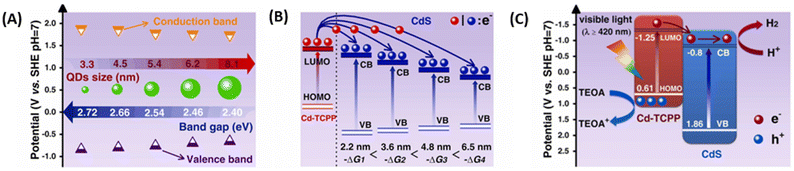 |
| Fig. 23 (A) Size-dependent bandgaps and energy-band positions of CdS QDs with various sizes. (B) Schematic diagram of the CB position in relation to −ΔG. The spheres denote photoinduced electrons. (C) The exciton dissociation and transfer in the CdS/Cd-TCPP. The spheres denote photoinduced electrons and holes, respectively from ref. 390. Copyright 2022, Elsevier B.V. | |
In addition to hydrogen production via water electrolysis, hydrogen can also be generated from hydrogen storage compounds, such as acetic acid and formic acid. Formic acid is widely regarded as a highly advantageous liquid organic hydrogen carrier, primarily due to its facile synthesis from CO2 and its notable solubility in water, eliminating the need to recover byproducts that deplete H2. Hermenegildo Garcia et al. used a titanium-based MOF, MIP-177-LT (ligand = 3,3′,5,5′-tetracarboxydiphenylmethane (H4mdip), LT = low temperature).391 MIP-177-LT was an efficient photocatalyst that catalyzed the release of H2 from formic acid without the need for neutralizing acidity, or the additional use of sacrificial agents or noble metals. Notably, the photocatalytic H2 release has a quantum efficiency of 22%, which is an order of magnitude higher than the decomposition activity of benchmark MOF photocatalysts such as MIL-125(Ti) and UiO-66(Zr) and over 30 times that of TiO2P25. This is particularly significant for a non-toxic, precious metal-free photocatalyst.
Moreover, Jiang et al. first reported on the coupling of photocatalytic hydrogen production with a value-added oxidation reaction. The photogenerated hydrogen production of MOF composite materials can be combined with selective benzylamine oxidation under light irradiation.392 Using PCN-777 (formulated Zr6O4(OH)10(H2O)6(TATB)2) with a conjugated H3TATB (4,4′,4′′-s-triazine-2,4,6-triyl-tribenzoic acid) ligand, upon MOF photoexcitation, electrons quickly reduced protons to generate H2, while holes promoted the oxidation of benzylamine (Fig. 24). Li et al. successfully synthesized three types of MOF: Ni–Ni PMOF, Zr PMOF, and Zr–Ni PMOF; all of them were based on TCPP using the solvothermal method.393 The study found that among these MOFs, Zr–Ni PMOF exhibited excellent photocatalytic coupling properties, which can couple the photoreaction of aromatic alcohols with enhanced H2 evolution (or CO2 reduction).
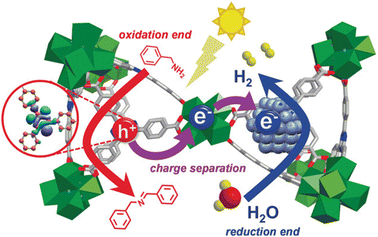 |
| Fig. 24 Illustration of simultaneous proton reduction and selective benzylamine oxidation over Pt/PCN-777 by photocatalysis from ref. 392. Copyright 2018, Wiley-VCH Verlag GmbH & Co. KGaA, Weinheim. | |
We summarized examples of photocatalytic hydrogen production in the past five years, as shown in Table 4.
Table 4 Examples of photocatalytic hydrogen production in the past five years
Catalyst |
H2 production rate |
Sacrificial reductant |
Light source |
H2 source |
Ref. |
TEOA refers to triethanolamine. PMOF refers to porphyrin-based MOF. ZSTU refers to Zhejiang Sci-Tech University. TCPP(Pd) refers to Pd-metalized tetra(4-carboxyphenyl)porphyrin. mPT refers to mixed phenanthroline dibenzoate. MIP refers to Materials from Institute of Porous Materials of Paris. LT refers to low temperature. BIH refers to 1,3-dimethyl-2-phenyl-2,3-dihydro-1H-benzo[d]imidazole. |
Co0.74Fe0.26-MOF-74 |
1214.32 μmol h−1 g−1 |
TEOA |
Visible |
H2O |
394
|
PMOF |
8.52 mmol g−1 h−1 |
Ascorbic acid |
Visible |
H2O |
395
|
MoO3/MIL-125-NH2 |
399.0 μmol h−1 g−1 |
Triethylamine |
Visible |
H2O |
396
|
V2O5/MIL-125-NH2 |
298.6 μmol h−1 g−1 |
Triethylamine |
Visible |
H2O |
396
|
Ni3(HITP)2/TiO2 |
350 μmol h−1 g−1 |
Ethylene glycol |
UV–vis |
H2O |
388
|
Zn 0.5 Cd 0.5S/M-TiO2 |
180.4 mmol g−1 h−1 |
NaS·9H2O and Na2SO3 |
Visible |
H2O |
397
|
0.5(Co/Zr)-UiO-66-NH2 |
29.94 μmol h−1 g−1 |
CH3 OH |
Visible |
H2O |
398
|
NH2-ZSTU-2 |
431.45 μmol h−1 g−1 |
TEOA |
Visible |
H2O |
399
|
2% Pt/Zr-TCPP(Pd) |
3348 μmol h−1 g−1 |
Ascorbic acid |
Visible |
H2O |
386
|
Au@NH2-UiO-66/CdS |
664.9 μmol h−1 g−1 |
Lactic acid acetonitrile |
Visible |
H2O |
400
|
In-MOF |
777.65 μmol h−1 g−1 |
TEOA |
UV–vis |
H2O |
401
|
ZnIn2S4-NiO@MOF |
4970.9 μmol h−1 g−1 |
TEOA |
Visible |
H2O |
402
|
CdS/Cd-TCPP |
3150 μmol h−1 g−1 |
TEOA |
Visible |
H2O |
390
|
mPT-Cu/Co |
TON: 18 700 |
BIH |
Visible |
Acetic acid |
403
|
MIP-177-LT |
650 μmol h−1 g−1 |
— |
Solar |
Formic acid |
391
|
3.5. Organic transformations
Presently, there is a substantial interest in MOFs as solid catalysts for organic reactions. The attractiveness of photocatalytic reactions lies in their ability to proceed under mild conditions, aligning well with the principles of green chemistry and sustainability for environmental conservation. MOFs offer a combination of heterogeneous catalysis advantages, such as easy recovery and high stability, along with homogeneous catalysis benefits, including selectivity, controllability, efficiency and mild reaction conditions. In recent times, significant progress has been made in the research on MOFs for photocatalytic organic transformations.404,405 We provide a review of the types of photocatalytic reaction emerging in the past five years as shown in Fig. 25 and below is a summary of typical photocatalytic organic reactions.
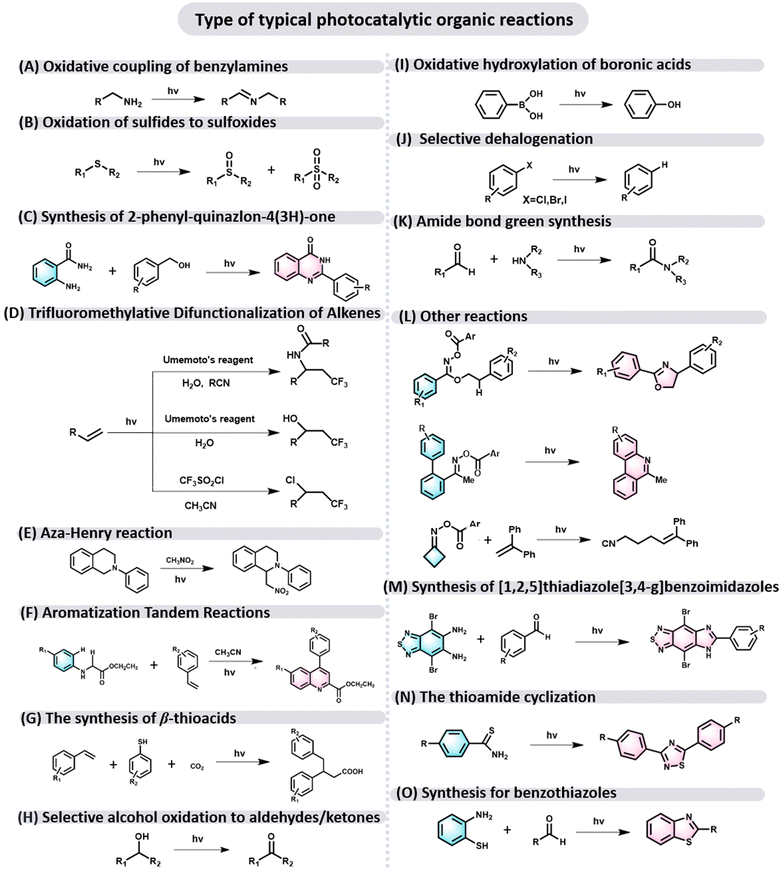 |
| Fig. 25 Scheme of the photocatalytic oxidation reaction with MOF. | |
3.5.1. Oxidative coupling of benzylamines.
Considering the inherent properties of ketones or aldehydes, the conventional approach to imine generation via amine and carbonyl dehydration condensation necessitates expensive dehydration reagents and often presents challenges in product control. The amines-to-imines photocatalytic oxidative coupling represents a significant functional group transformation. This method offers not harsh conditions, environmental friendliness and economic efficiency, circumventing the excessive use of Lewis acids, toxic catalysts and expensive dehydrants typical in conventional thermal catalysis processes. Considering the significance and the straightforwardness of the reaction, the photocatalytic oxidation coupling of benzylamine has emerged as a prevalent basic reaction for assessing novel photoactive MOFs and COFs in the field of photocatalysis.406
Li et al. synthesized a hybrid structure comprising ultra-small Pd nanocrystals and MOFs, evaluating its photocatalytic performance using benzylamine coupling as a model reaction.407 The findings revealed that the Pd/MIL-125-NH2 composite material demonstrated a notably enhanced photocatalytic performance compared with MIL-125-NH2, attaining a conversion efficiency of 94.08% and an impressive benzylamine conversion rate of 3136 μmol h−1 gcat−1. This enhancement is attributed to the Pd/MIL-125-NH2 superior molecular activation capability, with ˙O2− and 1O2 identified as the primary oxidizing species. Thus, the Pd/MIL-125-NH2 structure facilitates the transfer and separation of photogenerated electrons, providing catalytic sites for molecular activation and thereby enhancing the activity of the reaction.
Li et al. synthesized a series of UiO-68 (ligand = 4,4′-(benzo[c][1,2,5]thiadiazole-4,7-diyl)dibenzoic (H2L)) with different metals to study the impact of various metals on catalytic activity.408 They incorporated an excellent photosensitizer, D–A–D type H2L, into MOFs, which has been proved to significantly promote charge separation. Four 3D MOFs were synthesized (isostructural 1-Co/1-Zn with Co2/Zn2 units) and 2-Gd/2-Tb (Gd/Tb cluster chains) as photocatalysts. The structure–performance relationship indicated that the photocatalytic activity of benzylamines and phenylsulfides can be influenced by various transition metal ions, but not by lanthanide ions. Among them, 1-Zn is a robust multiphase catalyst with excellent performance, effectively oxidizing and removing benzylamines and phenylsulfides at room temperature (Fig. 26A).
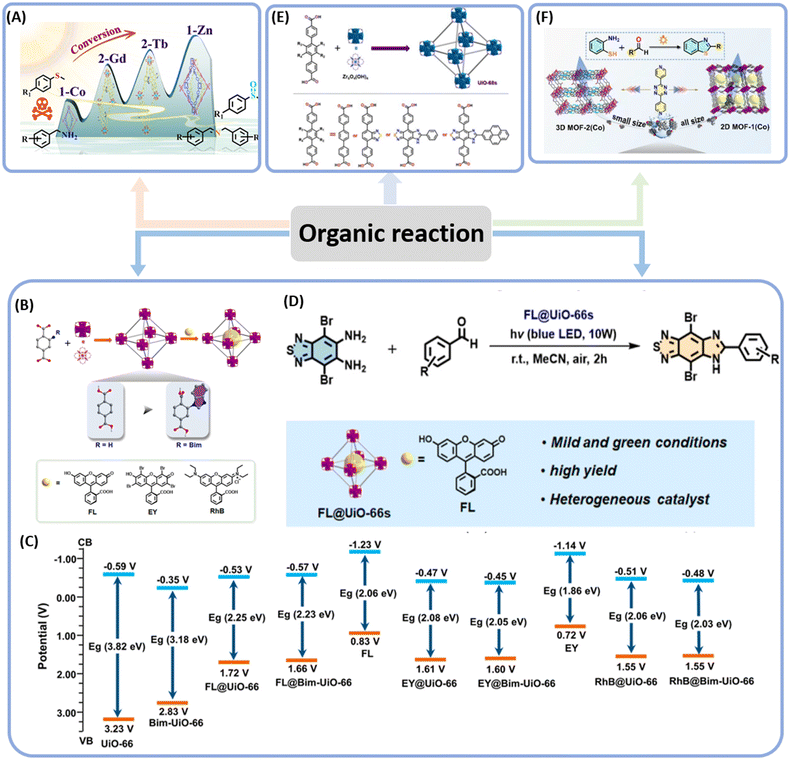 |
| Fig. 26 (A) Schematic showing the synthesis of UiO-68s from ref. 408. Copyright 2024, Royal Society of Chemistry. (B–D) The synthesis for UiO-66s and dye@UiO-66s; band structure figure of dyes, UiO-66s and dye@UiO-66s; our work for synthesis of [1,2,5]thiadiazole[3,4-g]benzoimidazoles from ref. 435. Copyright 2023, American Chemical Society. (E) MOFs with different metals catalyze the oxidation and removal of harmful benzylamines and phenylsulfides under visible light from ref. 436. Copyright 2023, American Chemical Society. (F) Schematic diagram of 3D MOF-2(Co) and 2D-MOF-1(Co) photocatalytic organic transformation from ref. 437. Copyright 2023, Elsevier B.V. | |
Zhang et al. synthesized a multifunctional heterostructure photocatalyst Cu-NH2-MIL-125/TpPa-2-COF, with Cu-NH2-MIL-125/TpPa-2-COF (4
:
6) exhibiting a high rate of visible light-driven hydrogen evolution. This photocatalyst demonstrated both high conversion (91.2%) and selectivity (>99%), effectively oxidizing amines to imines.406 Meanwhile, Jiang et al. utilized porphyrin MOF (PCN-222) to promote the visible-light-catalyzed oxidative coupling of various amines.409 Research has shown that PCN-222 exhibited excellent photocatalytic activity, which is attributed to the synergistic interactions between energy transfer-generated 1O2 and charge transfer-induced electrons and holes that promote the reaction.
3.5.2. Oxidation of sulfides to sulfoxides.
Sulfoxides serve as widely used synthetic reagents in both industrial production and pharmaceutical applications. Oxidation of thioether stands as a common method for producing corresponding sulfoxides. Conventional approaches often rely on heavy metal ions or harsh oxidants like tert-butyl hydroperoxide, hydrogen peroxide and trifluoroacetic acid, which can lead to environmental pollution. Conversely, the photocatalytic oxidation of sulfides utilizing visible light and oxygen exclusively as oxidants presents a promising opportunity for environmentally friendly sulfoxide synthesis, characterized by its simplicity, cost-effectiveness, mild reaction conditions and high efficiency and stability. Typically, sulfoxides emerge as primary products, with overoxidation products as byproducts. Notably, the photocatalytic oxidative degradation of chemical warfare agents exemplified a significant application of sulfide oxidation, addressing the persistent threat posed by such agents in global communities over decades. Among these agents, bis (2-chloroethyl) sulfide (mustard gas) (HD) stands out as one of the most potent, and notorious for its blistering effects. Initially, HD and its less toxic analogue, 2-chloroethyl ethyl sulfide (CEES), were studied due to CEES's reduced toxicity compared with HD while maintaining the sulfide portion.410 Researchers have developed various porous photocatalysts, including MOFs, to detoxify HD analogues such as CEES owing to their exceptional capacity to generate reactive oxygen species (ROS).411
Tang et al. prepared sandwich-like nanocomposites of UiO-66@Au25@UiO-66 by sandwiching Au25(Capt)18 (abbreviated as Au25, Capt = captopril) between UiO-66.412 The composite material exhibited notably improved catalytic efficiency and exceptional stability during the selective photocatalytic process of oxidizing sulfides to sulfoxides in an aerobic environment. Omar K. Farha et al. synthesized a microporous MOF, NU-400, which serves as an efficient photosensitizer, facilitating the production of 1O2 and subsequent oxidative degradation of chemical warfare agents.413 The remarkable activity of NU-400 facilitates the photocatalytic conversion of the CEES into non-toxic sulfoxide derivatives in air, with a half-life of less than 15 minutes.
Most research on the detoxification of CEES and the role of defects in MOFs during detoxification has been conducted in the liquid phase. Teresa J. Bandosz et al. reported on studies dealing with gas–solid interactions. They synthesized UiO-66 composites with nanographite or oxidized graphitic carbon nitride nanospheres, using them for the purification of gaseous CEES.414 The interactions between CEES vapor and UiO-66, its defective composites, nanographite, and oxidized graphitic carbon nitride nanospheres under ambient conditions were evaluated. The results indicate that these materials significantly enhance the detoxification capability of CEES vapor in terms of adsorption and surface reactivity. The composites can be used for the adsorptive degradation of gaseous CEES.
3.5.3. Synthesis of 2-phenyl-quinazlon-4(3H)-one.
Quinazolin-4(3H)-one derivatives are among the most significant heterocyclic compounds containing nitrogen, widely used in biological and clinical applications. They exhibit biological activities including antibacterial, antiviral, antidiabetic, phosphorylation inhibition, anti-inflammatory and anticancer properties.415 Therefore, besides the extraction of widespread present natural derivatives of quinazolinone, synthetic quinazolinones also hold significant research importance. As of now, there have been abundant reports documenting the synthesis of quinazolinone pharmacophores through reactions of alcohols with 2-aminobenzamide in catalytic systems such as Ru/Xantphos/Crotononitrile, Ir, and Pt under harsh conditions,416 a-MnO2/tert-butyl hydroperoxide (TBHP), Ru/Xantphos/Crotononitrile and Fe(BTC)/TBHP/DMSO,417 and Ni(II) complexes/NaOtBu/xylene. However, these systems suffer from drawbacks such as high catalyst costs, difficult catalyst recovery, catalyst deactivation, the use of additional additives and complex reaction conditions. Therefore, there is an urgent need to introduce catalysts that are low-cost, recyclable, efficient and environmentally friendly.
Ali Reza Oveisi et al. synthesized and cluster-metalized a novel multifunctional MOF Fe@PCN-222(Fe) by PSM of a bioinspired Fe(III) porphyrinic Zr-MOF (PCN-222(Fe)) with FeCl3.418 This solid displayed catalytic activity when exposed to visible light in the presence of air or oxygen, all without the need for any additives, synthesizing quinazolin-4(3H)-one via an oxidation–cyclization–oxidation reaction in a one-pot tandem process with alcohols and 2-aminobenzamide. Moreover, its catalytic performance surpasses that of bare PCN-222(Fe) and comparable heterogeneous catalysts such as UiO-66, Fe(BTC) and mixtures of UiO-66 with FeTCPPCl and FeCl3. This is the instance of using an MOF under an O2 atmosphere with visible light (or sunlight) to synthesize quinazolin-4(3H)-ones under mild and environmentally sustainable conditions.
3.5.4. Trifluoromethylative difunctionalization of alkenes.
Given the importance of CF3 groups in small-molecule drugs, evaluating the catalytic performance of photoreactions through the trifluoromethylative difunctionalization of alkenes serves as a model reaction, holding profound research significance. Lin et al. reported a bifunctional metal–organic layer (MOL) Hf-EY-Fe constructed from Fe-TPY (TPY = 4′-(4-carboxyphenyl)[2,2′:6′,2′′-terpyridine]-5,5′′-dicarboxylate) ligands and Eosin Y (EY)-Hf6 secondary building units (SBUs).419 The organic dye EY, serving as a photosensitizer, with TPY-Fe(OTf)2 acting as the catalytic core, enables Hf-EY-Fe to efficiently catalyze aminotrifluoromethylation, hydroxytrifluoromethylation and chlorotrifluoromethylation reactions of alkenes. Hf-EY-Fe can also catalyze the synthesis of CF3-substituted derivatives of biologically active molecules, such as estrone.
3.5.5. aza-Henry reaction.
The aza-Henry reaction stands out as one of the most prominently utilized photocatalytic model reactions, widely used to assess the photooxidoreductive activity of various catalytic systems. This reaction involves the functionalization of N-aryltetrahydroisoquinolines (THIQs) alongside other tertiary amines using various nucleophilic reagents. Many nucleophiles can act as coupling agents with oxygen serving as the terminal oxidant. Youngmee Kim et al. encapsulated the cations [Ru(bpy)3]2+ (bpy = 2,2′-bipyridine),420 [Ru(phen)3]2+ (phen = 1,10-phenanthroline) and [Ru(bpz)3]2+ (bpz = 2,2′-bipyrazine) within the mesopores of 3D InBTB MOF (ligand = 1,3,5-benzenetribenzoic acid) to prepare three new types of RuL3@InBTB MOF. The photocatalytic activity of RuL3@InBTB MOF was assessed using the aza-Henry reaction. Concurrently, the research explores both the hydrogenation of dimethyl maleate and the visible-light-induced decomposition of methyl orange at ambient temperature. The results indicated that RuL3@InBTB MOF exhibited high stability and recyclability.
3.5.6. Aromatization tandem reactions of glycine esters and styrenes.
For materials with high stability and excellent performance, their photocatalytic activity can also be evaluated through the photooxidative cross-dehydrogenative coupling (CDC)/aromatization tandem reactions of N-(4-methoxyphenyl)glycine and styrene. Chen et al. developed a highly delocalized interpenetrated 3D MOF Zn-TACPA (ligand = tris(3-carboxybiphenyl)amine (H3TACPA)) photocatalyst based on vinyl-functionalized triphenylamine and bipyridine ligands.421 It possesses high stability and delocalization, serving as an ROS generator to catalyze the photooxidative CDC/aromatization tandem reaction of glycine esters and styrenes. The introduction of functional vinyl double bonds enhanced the conjugation of the MOF semiconductor, charge carrier separation and charge transfer efficiency, significantly increasing its catalytic yield.
3.5.7. The synthesis of β-thioacids.
The synthesis of β-thioacids has garnered significant interest. However, traditional methods for synthesizing β-thioacids have numerous drawbacks, such as the need to use toxic H2S, low yields and cumbersome multi-step processes. Therefore, developing a photoassisted redox method using stable and recyclable photocatalysts, without the need for additional co-catalysts/alkali, for synthesizing thio-carboxylate olefins with CO2 into β-thioacids is of significant importance for green and economical synthesis. MOFs, with their aromatic donors and high oxidation state metals, facilitate photoinduced charge separation, and their distinctive porous structure and exceptionally high surface area have broad applications in photocatalysis.422
Suman L. Jain et al. reported an efficient, simple and economically feasible photoassisted redox-mediated method,422 which utilizes the porous functionalized Fe-MIL-101-NH2 as a photocatalyst, to synthesize important β-thioacids via the carboxylation of olefins with CO2 and thiols at room temperature.
3.5.8. Selective alcohol oxidation to aldehydes/ketones.
In industrial synthesis and laboratories, the selective oxidation of alcohols to aldehydes or ketones is a vital step in organic compound synthesis, as aldehyde or ketone derivatives are valuable fine chemical intermediates with wide applications in fields like medicine and the food industry.423 In the oxidation process of aromatic alcohols, the use of additional oxidizing agents (ClO−, KMnO4 and CrO3−) is often expensive and dangerous, causing environmental pollution and resource wasting. In contrast, visible light photocatalysis is an effective strategy that facilitates the selective oxidation of alcohols to benzaldehyde under mild conditions, without the need for additional co-catalysts.424
In the presence of Bi(NO3)3, Yin et al. successfully synthesized BFO/MIL-53(Fe) (BFO = bismuth ferrite) nanocomposites at room temperature by partially etching the peripheral structure of MIL-53(Fe).425 The prepared composites provided more active sites for photocatalytic reactions. To evaluate the photocatalytic performance of the composites, Shuang-Feng Yin studied the photocatalytic activity of BFO/MIL-53(Fe) nanocomposites for the partial oxidation of aromatic alcohols under visible light. The study found that the highest conversion rate reached 58.5% when the mass ratio of BFO to MIL-53(Fe) was 2.0.
3.5.9. Oxidative hydroxylation of boronic acids.
Phenol is a crucial raw material in the synthesis of fine chemicals. Traditional phenol synthesis involves complex and costly synthetic steps. Therefore, there is a need to develop green and efficient methods for producing phenol, particularly in synthesizing various multifunctional group-substituted phenols. Arylboronic acids and aromatic/heteroaromatic compounds are essential in organic synthesis.426 Many MOFs have been proved to be effective photocatalysts in the conversion of arylboronic acids to phenol. Generally speaking, phenol is the sole product of the oxidation of arylboronic acids. Additionally, using MOF photocatalysis for phenol production offers advantages such as good substrate adaptability, high yield, environmental friendliness and selectivity, with a well-defined reaction mechanism that can serve as a model reaction to preliminarily evaluate the photocatalytic activity of most MOFs catalysts.427
Li et al. reported the synthesis of a robust microporous Zn-MOF (JNU-204) with a pyrazole−benzothiadiazole−pyrazole (D–A–D type) photosensitizer as the organic linker, featuring a wuk topology.428 Using JNU-204 as a catalyst, three aerobic oxidation reactions were carried out via different oxygenation pathways, such as the oxidation of phenylboronic acid, demonstrating JNU-204's excellent photocatalytic activity. Recyclability tests confirmed its heterogeneous nature and structural integrity, with no significant loss of photocatalytic activity after three runs. The synthetic application of JNU-204 was further extended to the straightforward construction of N-heterocyclic derivatives. The pyrrolo[2,1-a]isoquinoline-containing heterocycles, a core framework of a series of marine natural products, can be synthesized via a series of photocatalytic oxidation/[3 + 2] cycloaddition/oxidative aromatizations.
3.5.10. Selective dehalogenation.
Organic halides constitute a group of chemicals that are commonly employed as intermediates or solvents in the process of organic synthesis.429 Nonetheless, the majority of organic halides are classified as persistent organic pollutants, which pose significant threats to both environmental well-being and human health.430 A highly efficient and environmentally friendly solution is selective dehalogenation via photocatalysis. Previous studies have used transition metal complexes to catalyze the aforementioned reactions, but due to limitations in conditions, stability and reusability, these efforts have not successfully resolved the issues. However, employing MOFs as catalysts for these reactions can effectively overcome these problems.
Niu et al. synthesized an NDI-based polymer using POMs and amine catalysts, designing a novel MOF, CoW-DPNDI−PYI (DPNDI = N,N′-bis(4-pyridylmethyl)-naphthalenediimide, PYI = pyrrolidine-2-yl-imidazole).431 Without the addition of any co-catalysts, a heterogeneous reduction of various aryl bromides and chlorides was achieved along with a significant impact on CO2 cycloaddition reactions under mild temperatures and sub-atmospheric pressure.
3.5.11. Amide bond green synthesis.
The formation of amide bonds is a crucial step in many pharmaceutical chemical synthesis processes. Therefore, the synthesis of amide compounds is a central issue in the synthesis of natural products and drug development. Traditionally, amide bonds are constructed by condensing carboxylic acids or their derivatives with amines, requiring condensing agents and relatively harsh reaction conditions; furthermore, this synthetic method generates a large amount of by-products. Consequently, oxidative amidation reactions of aldehydes or alcohols with amines have been developed to replace traditional amide bond synthesis routes. Utilizing MOFs for the photocatalytic conversion of aldehydes or alcohols to amide bonds offers a promising approach.432
Cheng et al. synthesized three distinct Fe–O clusters, Lewis acid sites and morphologies of Fe-MOF through coordination structure engineering.433 The findings suggest that Lewis acid sites play a crucial role in photocatalytic performance. Pd/MIL-101(Fe) demonstrates outstanding photocatalytic performance, catalyzing the conversion of various aldehydes, alcohols and toluene with amine compounds into amide compounds at ambient temperature.
3.5.12. Other reactions.
He et al. proposed embedding a copper(I) bimetallic photosensitizer in an economical and scalable zinc-based columnar layered MOF, for reactions involving both intra- and intermolecular radical-mediated N-acyloxy imidates and O-acyl oximes.434 Research has shown that anchoring a BINAP-containing copper photosensitizer onto the pillar-layered MOF supports significantly enhances its turnover frequency and recyclability in photocatalysis.
3.5.13. Synthesis of [1,2,5]thiadiazole[3,4-g]benzoimidazoles.
Introducing dye molecules is a promising method to effectively enhance the activity of MOF photocatalysts. In 2023, Li et al. reported an efficient strategy to confine and stabilize photosensitive organic dyes within MOF frameworks,435 developing a series of dye-loaded photocatalysts, dye@UiO-66s (dye = fluorescein (FL)/rhodamine B (RhB)/Eosin Y (EY), UiO-66s = UiO-66 and Bim-UiO-66) (Fig. 26B). The results demonstrated that regardless of the functionalization of the MOF ligands, the encapsulated dyes effectively sensitize the MOF matrix, dominating the band structure and photocatalytic activity of dye@UiO-66s (Fig. 26C). Photocatalytic experiments indicated that dye@UiO-66s show enhanced activity compared with free dyes, with FL@Bim-UiO-66 efficiently catalyzing the green synthesis of novel carbon-bridged cycles [1,2,5]thiadiazole[3,4-g]benzoimidazoles at room temperature, achieving yields up to 98% with exhibited excellent stability and reusability (Fig. 26D).
3.5.14. The thioamide cyclization.
Linker engineering is an effective strategy for imparting specific properties or new functionalities to targeted MOFs. Li et al. incorporated various benzothiadiazole units into the ligands to systematically regulate the activity of the MOF (UiO-68, UiO-68-Bs, UiO-68-Ph, UiO-68-Py) photocatalysts, and the light absorption and charge separation efficiency can be improved. Among these photocatalysts, UiO-68-Py composed of a fully conjugated benzothiadiazole acceptor and pyrene donor was proved to be an efficient and recyclable photocatalyst in facilitating the cyclization of thioamides under mild conditions (Fig. 26E).436
3.5.15. Synthesis for benzothiazoles.
Highly active and selective visible light heterogeneous photocatalysts are crucial for the advancement of organic transformations, yet they remain a formidable challenge. Herein, Li et al. have developed a simple yet effective strategy to integrate tetrazine parts into a robust MOF.437 These include 2D MOF-1(M), (M = Co, Mn, Zn) and 3D MOF-2(Co). The MOF-1 series consisted of amorphous 2D porous frameworks, while MOF-2(Co) features a 3D porous structure. Due to the oxidative activity of ˙O2− species, these MOFs exhibited significantly enhanced photocatalytic activity in the direct condensation of o-aminothiophenol and aromatic aldehydes under visible white light irradiation. Notably, MOF-1(Co) demonstrated superior catalytic activity, exhibiting broad substrate adaptability, excellent steric hindrance tolerance, remarkable reusability, and outstanding stability (Fig. 26F).
4. Summary and outlook
In recent years, considering the non-renewability of fossil fuels and environmental pollution issues, new photocatalytic materials, MOFs, have gradually come into view. MOFs have highly ordered porous structures, large specific surface areas and abundant active and adsorption sites. Their inherent narrow bandgaps and thermal stability endow them with superior catalytic activity. Then, due to the structural diversity and design flexibility of MOFs, the catalytic reaction sites can be adjusted by designing bandgaps, controlling channels and regulating pore sizes. This review systematically summarises the optimization and application of visible light photocatalytic MOFs, focusing on the basic reaction types and specific reactions of photocatalytic organic transformations. It aims to provide a systematic guide for future researchers, from the design of MOFs to performance improvement and specific photocatalytic organic transformations. However, despite recent significant progress in MOF photocatalytic organic transformations, the following points may require further research to address the design challenges and performance enhancement of MOF-based catalysts, paving the way for future applications:
(1) The synthesis of MOFs typically involves complex conditions such as high temperatures, specific pH values, or the use of organic solvents, which limit their large-scale production and application. Therefore, optimizing the structure of ligands and central metal clusters, simplifying the synthesis process of MOFs and developing room temperature synthesis routes, aqueous synthesis methods, or green solvent systems, are currently important research directions.
(2) In many applications, the size and morphology of MOFs significantly affect their performance. Currently, precisely controlling the size and morphology of MOF crystals remains a technical challenge. By improving synthesis conditions, using templates, or adding growth regulators, the morphology and size of MOFs can be controlled to some extent, but further research is needed to achieve more precise control.
(3) Environmental factors, such as humidity, temperature and chemical corrosion, impact the stability of MOFs markedly, and the pore size of MOFs also significantly affects the stability.438 With the enlargement of pore size, its chemical stability and thermal stability usually decline. Developing MOF materials with high environmental stability and precision regulation of the pore structure of MOFs is crucial, and in-depth research on the correlation between the porosity and stability of MOFs, coupled with the continuous optimization of material design, will further expand the application prospects of MOFs in photocatalysis.439
(4) In practical applications the recyclability of MOFs has become an important factor influencing their photocatalytic performance and economic feasibility. To achieve MOFs with recyclable properties, future innovations in material selection, structural design, and post-treatment techniques can be explored, thereby reducing environmental pollution and achieving sustainable development.
(5) The mechanisms behind the formation of MOFs under various synthesis conditions are not yet fully understood. It is crucial to deeply understand the formation and catalytic mechanisms of MOFs and their composite materials. Therefore, conducting an in-depth study of their formation mechanisms through in situ characterization and DFT results is significant for future research on photocatalytic mechanisms.
(6) Although MOFs demonstrate numerous excellent properties, their commercial prospects are limited by high production costs and resource consumption. Finding more cost-effective materials, simplifying manufacturing processes and enhancing the multifunctionality of materials are key strategies to reduce costs and increase efficiency.
(7) The manufacture of functional materials with multifunctional applications has attracted increasing research interest. Although different types of MOF photocatalyst have rapidly developed applications in single fields such as HER, OER, NRR and CO2RR, MOF-based composite materials as bifunctional or trifunctional photocatalysts have been rarely reported. Therefore, developing and designing bifunctional or trifunctional photocatalysts based on MOFs and their composites presents significant challenges.
(8) Meanwhile, the poor separation efficiency of photogenerated e−–h+ in photocatalysis severely limits the photocatalytic efficiency. How to improve photocatalytic efficiency is a hot topic among researchers. To cut down the recombination rate of photogenerated charge carriers, creating an internal electric field as a driving force in order to separate the charges has been considered an effective strategy. Therefore, using piezoelectric materials for piezo-photocatalysis, which simultaneously undergo light irradiation and mechanical stress, is of great significance.440
(9) In recent years, artificial intelligence technology has developed rapidly; AI can predict and design new MOFs with specific properties by learning the relationships between the structures and properties of existing materials, allowing us to bypass the experimental process and directly obtain results. In the future, with the continuous development of AI technology and the improvement of material databases, the application of AI in MOF research will become more extensive and in-depth, holding great potential.
In response to the challenges mentioned above, future research should focus on material design, synthesis optimization, application exploration and mechanism studies. Developing new synthesis methods, such as using biosynthesis or green chemistry approaches, can not only simplify the production process but also reduce the environmental impact. In application development, using AI technology to predict the structure and performance of MOFs, guiding their controlled design and synthesis and exploring their development in organic transformation will be an important direction for future research. Through these integrated efforts, the research and application prospects of visible-light MOFs will become even broader.
Author contributions
Xu-Sheng Li and Yu-Jie He: contributed equally; writing – original draft. Jiao Chen: writing – review & editing. Quan-Quan Li: writing – review & editing. Ping Liu: supervision, project administration. Jian-Li Li: supervision, project administration.
Data availability
No primary research results, software or code have been included and no new data were generated or analysed as part of this review.
Conflicts of interest
There are no conflicts to declare.
Acknowledgements
This work was supported by the National Natural Science Foundation of China (No. 22077099, 22171223 and 22307102), the Innovation Capability Support Program of Shaanxi (No. 2023-CX-TD-75 and 2024ZC-KJXX-040), the Technology Innovation Leading Program of Shaanxi (No. 2023KXJ-209 and 2024QCY-KXJ-142), the Key Research and Development Program of Shaanxi (No. 2024GH-ZDXM-22), the Natural Science Basic Research Program of Shaanxi (No. 2023-JC-YB-141), and Young Talent Fund of Association for Science and Technology in Shaanxi, China (No. SWYY202206), the Shaanxi Fundamental Science Research Project for Chemistry & Biology (No. 22JHZ010, 22JHQ080, and 23JHQ039), the Yan'an City Science and Technology Project (No. 2022SLZDCY-002), and the Key Laboratory of Hexi Corridor Resources Utilization (No. ZDSYS-2023-2).
References
- Z. Qian, R. Zhang, Y. Xiao, H. Huang, Y. Sun, Y. Chen, T. Ma and X. Sun, Trace to the Source: Self-Tuning of MOF Photocatalysts, Adv. Energy Mater., 2023, 13, 2300086 CrossRef CAS.
- X. Meng, L. Liu, S. Ouyang, H. Xu, D. Wang, N. Zhao and J. Ye, Nanometals for Solar-to-Chemical Energy Conversion: From Semiconductor-Based Photocatalysis to Plasmon-Mediated Photocatalysis and Photo-Thermocatalysis, Adv. Mater., 2016, 28, 6781–6803 CrossRef CAS PubMed.
- D. G. Nocera, Solar Fuels and Solar Chemicals Industry, Acc. Chem. Res., 2017, 50, 616–619 CrossRef CAS PubMed.
- J. D. Xiao, R. Li and H. L. Jiang, Metal-Organic Framework-Based Photocatalysis for Solar Fuel Production, Small Methods, 2023, 7, 2201258 CrossRef CAS PubMed.
- J. Qiu, X. Zhang, Y. Feng, X. Zhang, H. Wang and J. Yao, Modified metal-organic frameworks as photocatalysts, Appl. Catal., B, 2018, 231, 317–342 CrossRef CAS.
- K. Hashimoto, H. Irie and A. Fujishima, TiO2 Photocatalysis: A Historical Overview and Future Prospects, Jpn. J. Appl. Phys., 2005, 44, 8269–8285 CrossRef CAS.
- V. G. Dileepkumar, K. R. Balaji, R. Vishwanatha, B. M. Basavaraja, S. Ashoka, I. M. Al-Akraa, M. S. Santosh and S. Rtimi, CoSe2 grafted on 2D gC3N4: A promising material for wastewater treatment, electrocatalysis and energy storage, Chem. Eng. J., 2022, 446, 137023 CrossRef CAS.
- P. Jiménez-Calvo, V. Caps and V. Keller, Plasmonic Au-based junctions onto TiO2, gC3N4, and TiO2-gC3N4 systems for photocatalytic hydrogen production: Fundamentals and challenges, Renewable Sustainable Energy Rev., 2021, 149, 111095 CrossRef.
- P. Jiménez-Calvo, V. Caps, M. N. Ghazzal, C. Colbeau-Justin and V. Keller, Au/TiO2(P25)-gC3N4 composites with low gC3N4 content enhance TiO2 sensitization for remarkable H2 production from water under visible-light irradiation, Nano Energy, 2020, 75, 104888 CrossRef.
- D. Gopalakrishnan, D. Damien and M. M. Shaijumon, MoS2 Quantum Dot-Interspersed Exfoliated MoS2 Nanosheets, ACS Nano, 2014, 8, 5297–5303 CrossRef CAS PubMed.
- C. Zhu, C. a. Liu, Y. Fu, J. Gao, H. Huang, Y. Liu and Z. Kang, Construction of CDs/CdS photocatalysts for stable and efficient hydrogen production in water and seawater, Appl. Catal., B, 2019, 242, 178–185 CrossRef CAS.
- Y.-l. Li, X.-j. Liu, Y.-b. Wang, Y. Liu, R.-h. Liu, H.-y. Mu, Y.-j. Hao, X.-j. Wang and F.-t. Li, Constructing interfacial electric field and Zn vacancy modulated ohmic junctions ZnS/NiS for photocatalytic H2 evolution, Green Energy Environ., 2024 DOI:10.1016/j.gee.2023.12.007.
- H. X. Lu, H. Liu, Z. Z. Fu, Y. Y. Chen, H. Q. Dai, Z. Hu, W. L. Zhang and R. Q. Guo, Rational design of AgGaS/ZnS/ZnS quantum dots with a near-unity photoluminescence quantum yield via double shelling scheme, J. Mater. Sci. Technol., 2024, 169, 235–242 CrossRef CAS.
- A. Naldoni, M. Altomare, G. Zoppellaro, N. Liu, Š. Kment, R. Zbořil and P. Schmuki, Photocatalysis with Reduced TiO2: From Black TiO2 to Cocatalyst-Free Hydrogen Production, ACS Catal., 2018, 9, 345–364 CrossRef PubMed.
- M. J. Valero-Romero, J. G. Santaclara, L. Oar-Arteta, L. van Koppen, D. Y. Osadchii, J. Gascon and F. Kapteijn, Photocatalytic properties of TiO2 and Fe-doped TiO2 prepared by metal organic framework-mediated synthesis, Chem. Eng. J., 2019, 360, 75–88 CrossRef CAS.
- Z. Jiao, M. Shang, J. Liu, G. Lu, X. Wang and Y. Bi, The charge transfer mechanism of Bi modified TiO2 nanotube arrays: TiO2 serving as a “charge-transfer-bridge”, Nano Energy, 2017, 31, 96–104 CrossRef CAS.
- H. Wang, B. Jin, H. Wang, N. Ma, W. Liu, D. Weng, X. Wu and S. Liu, Study of Ag promoted Fe2O3@CeO2 as superior soot oxidation catalysts: The role of Fe2O3 crystal plane and tandem oxygen delivery, Appl. Catal., B, 2018, 237, 251–262 CrossRef CAS.
- P. Zheng, Y. Zhang, Z. Dai, Y. Zheng, K. N. Dinh, J. Yang, R. Dangol, X. Liu and Q. Yan, Constructing Multifunctional Heterostructure of Fe2O3@Ni3Se4 Nanotubes, Small, 2018, 14, 1704065 CrossRef PubMed.
- D. Jiang, P. Xu, H. Wang, G. Zeng, D. Huang, M. Chen, C. Lai, C. Zhang, J. Wan and W. Xue, Strategies to improve metal organic frameworks photocatalyst's performance for degradation of organic pollutants, Coord. Chem. Rev., 2018, 376, 449–466 CrossRef CAS.
- A. Dhakshinamoorthy, A. M. Asiri and H. Garcia, Metall–organische Gerüstverbindungen: Photokatalysatoren für Redoxreaktion und die Produktion von Solarbrennstoffen, Angew. Chem., 2016, 128, 5504–5535 CrossRef.
- P. Valvekens, F. Vermoortele and D. De Vos, Metal–organic frameworks as catalysts: the role of metal active sites, Catal. Sci. Technol., 2013, 3, 1435–1445 RSC.
- M. Alvaro, E. Carbonell, B. Ferrer, F. X. Llabrés i Xamena and H. Garcia, Semiconductor Behavior of a Metal-Organic Framework (MOF), Chem. – Eur. J., 2007, 13, 5106–5112 CrossRef CAS PubMed.
- Y. Fu, D. Sun, Y. Chen, R. Huang, Z. Ding, X. Fu and Z. Li, An Amine-Functionalized Titanium Metal-Organic Framework Photocatalyst with Visible-Light-Induced Activity for CO2 Reduction, Angew. Chem., Int. Ed., 2012, 51, 3364–3367 CrossRef CAS PubMed.
- M. Xu, P. Zhu, Q. Cai, M. Bu, C. Zhang, H. Wu, Y. He, M. Fu, S. Li and X. Liu,
In situ fabrication of TiO2/NH2-MIL-125(Ti) via MOF-driven strategy to promote efficient interfacial effects for enhancing photocatalytic NO removal activity, Chin. Chem. Lett., 2024, 35, 109524 CrossRef CAS.
- J.-D. Xiao and H.-L. Jiang, Metal–Organic Frameworks for Photocatalysis and Photothermal Catalysis, Acc. Chem. Res., 2019, 52, 356–366 CrossRef CAS PubMed.
- C. Mellot-Draznieks, J. Dutour and G. Férey, Hybrid Organic-Inorganic Frameworks: Routes for Computational Design and Structure Prediction, Angew. Chem., Int. Ed., 2004, 43, 6290–6296 CrossRef CAS PubMed.
- G. Férey, Hybrid porous solids: past, present, future, Chem. Soc. Rev., 2008, 37, 191–214 RSC.
- D. J. Tranchemontagne, J. L. Mendoza-Cortés, M. O'Keeffe and O. M. Yaghi, Secondary building units, nets and bonding in the chemistry of metal–organic frameworks, Chem. Soc. Rev., 2009, 38, 1257–1283 RSC.
- H. Furukawa, K. E. Cordova, M. O'Keeffe and O. M. Yaghi, The Chemistry and Applications of Metal-Organic Frameworks, Science, 2013, 341, 1230444 CrossRef PubMed.
- Z. Jiang, X. Xu, Y. Ma, H. S. Cho, D. Ding, C. Wang, J. Wu, P. Oleynikov, M. Jia, J. Cheng, Y. Zhou, O. Terasaki, T. Peng, L. Zan and H. Deng, Filling metal-organic framework mesopores with TiO2 for CO2 photoreduction, Nature, 2020, 586, 549–554 CrossRef CAS PubMed.
- C. G. Silva, I. Luz, F. X. Llabrés i Xamena, A. Corma and H. García, Water Stable Zr–Benzenedicarboxylate Metal–Organic Frameworks as Photocatalysts for Hydrogen Generation, Chem. – Eur. J., 2010, 16, 11133–11138 CrossRef CAS PubMed.
- Q. Wang and D. Astruc, State of the Art and Prospects in Metal–Organic Framework (MOF)-Based and MOF-Derived Nanocatalysis, Chem. Rev., 2019, 120, 1438–1511 CrossRef PubMed.
- W. Wang, D. Chen, F. Li, X. Xiao and Q. Xu, Metal-organic-framework-based materials as platforms for energy applications, Chem, 2024, 10, 86–133 CAS.
- T. Xia, Y. Lin, W. Li and M. Ju, Photocatalytic degradation of organic pollutants by MOFs based materials: A review, Chin. Chem. Lett., 2021, 32, 2975–2984 CrossRef CAS.
- Y. Ma, H.-X. Fang, R. Chen, Q. Chen, S.-J. Liu, K. Zhang and H.-J. Li, 2D-MOF/2D-MOF heterojunctions with strong hetero-interface interaction for enhanced photocatalytic hydrogen evolution, Rare Met., 2023, 42, 3993–4004 CrossRef CAS.
- P. Cheng, C. Wang, Y. V. Kaneti, M. Eguchi, J. Lin, Y. Yamauchi and J. Na, Practical MOF Nanoarchitectonics: New Strategies for Enhancing the Processability of MOFs for Practical Applications, Langmuir, 2020, 36, 4231–4249 CrossRef CAS PubMed.
- W. Sheng, X. Wang, Y. Wang, S. Chen and X. Lang, Integrating TEMPO into a Metal–Organic Framework for Cooperative Photocatalysis: Selective Aerobic Oxidation of Sulfides, ACS Catal., 2022, 12, 11078–11088 CrossRef CAS.
- O. M. Yaghi, G. Li and H. Li, Selective binding and removal of guests in a microporous metal–organic framework, Nature, 1995, 378, 703–706 CrossRef CAS.
- J. Huang, K. Li, L. Wang, H. She and Q. Wang, In situ conversion builds MIL-101@NiFe-LDH heterojunction structures to enhance the oxygen evolution reaction, Chin. Chem. Lett., 2022, 33, 3787–3791 CrossRef CAS.
- Y. Su, M. Lu, R. Su, W. Zhou, X. Xu and Q. Li, A 3D MIL-101@rGO composite as catalyst for efficient conversion of straw cellulose into valuable organic acid, Chin. Chem. Lett., 2022, 33, 2573–2578 CrossRef CAS.
- T. Hidalgo, M. Giménez-Marqués, E. Bellido, J. Avila, M. C. Asensio, F. Salles, M. V. Lozano, M. Guillevic, R. Simón-Vázquez, A. González-Fernández, C. Serre, M. J. Alonso and P. Horcajada, Chitosan-coated mesoporous MIL-100(Fe) nanoparticles as improved bio-compatible oral nanocarriers, Sci. Rep., 2017, 7, 43099 CrossRef CAS PubMed.
- Z.-F. Yu, Y. Yang, H.-F. Zhuang, S.-D. Shan, M.-S. Beldean-Galea, Q.-Q. Xue, X.-F. Shen and S.-J. Li,
In situ growth of MIL-53 (Fe) on charcoal sponge as a highly efficient and recyclable photocatalyst for removal of Cr(VI), Rare Met., 2024, 43, 4344–4355 CrossRef CAS.
- A. Dhakshinamoorthy, Z. Li, S. Yang and H. Garcia, Metal–organic framework heterojunctions for photocatalysis, Chem. Soc. Rev., 2024, 53, 3002–3035 RSC.
- Z. Xue, M. Yan, Y. Zhang, J. Xu, X. Gao and Y. Wu, Understanding the injection process of hydrogen on Pt1-TiO2 surface for photocatalytic hydrogen evolution, Appl. Catal., B, 2023, 325, 122303 CrossRef CAS.
- Y. Huang, Z. Li, K. Yao, C. Chen, C. Deng, Y. Fang, R. Li and H. Tian, Suppressing toxic intermediates during photocatalytic degradation of glyphosate by controlling adsorption modes, Appl. Catal., B, 2021, 299, 120671 CrossRef CAS.
- Y. Yang, Z. Chen, H. Huang, Y. Liu, J. Zou, S. Shen, J. Yan, J. Zhang, Z. Zhuang, Z. Luo, C. Yang, Y. Yu and Z. Zou, Synergistic surface activation during photocatalysis on perovskite derivative sites in heterojunction, Appl. Catal., B, 2023, 323, 122146 CrossRef CAS.
- Y. Zhiyong, M. Bensimon, V. Sarria, I. Stolitchnov, W. Jardim, D. Laub, E. Mielczarski, J. Mielczarski, L. Kiwi-Minsker and J. Kiwi, ZnSO4−TiO2 doped catalyst with higher activity in photocatalytic processes, Appl. Catal., B, 2007, 76, 185–195 CrossRef CAS.
- J. Byun, K. Landfester and K. A. I. Zhang, Conjugated Polymer Hydrogel Photocatalysts with Expandable Photoactive Sites in Water, Chem. Mater., 2019, 31, 3381–3387 CrossRef CAS.
- Y. Pan, R. Abazari, J. Yao and J. Gao, Recent progress in 2D metal-organic framework photocatalysts: synthesis, photocatalytic mechanism and applications, J. Phys.: Energy, 2021, 3, 032010 CAS.
- G. Zhang, G. Li, Z. A. Lan, L. Lin, A. Savateev, T. Heil, S. Zafeiratos, X. Wang and M. Antonietti, Optimizing Optical Absorption, Exciton Dissociation, and Charge Transfer of a Polymeric Carbon Nitride with Ultrahigh Solar Hydrogen Production Activity, Angew. Chem., Int. Ed., 2017, 56, 13445–13449 CrossRef CAS PubMed.
- A. Meng, L. Zhang, B. Cheng and J. Yu, Dual Cocatalysts in TiO2 Photocatalysis, Adv. Mater., 2019, 31, 1807660 CrossRef PubMed.
- Y. Nosaka and A. Y. Nosaka, Generation and Detection of Reactive Oxygen Species in Photocatalysis, Chem. Rev., 2017, 117, 11302–11336 CrossRef CAS PubMed.
- K. Hendrickx, D. E. P. Vanpoucke, K. Leus, K. Lejaeghere, A. Van Yperen-De Deyne, V. Van Speybroeck, P. Van Der Voort and K. Hemelsoet, Understanding Intrinsic Light Absorption Properties of UiO-66 Frameworks: A Combined Theoretical and Experimental Study, Inorg. Chem., 2015, 54, 10701–10710 CrossRef CAS PubMed.
- L. Shen, R. Liang, M. Luo, F. Jing and L. Wu, Electronic effects of ligand substitution on metal–organic framework photocatalysts: the case study of UiO-66, Phys. Chem. Chem. Phys., 2015, 17, 117–121 RSC.
- K. Zhang, F. Chu, Y. Hu, X. Huang, G. Zhao and G. Wang, Ce-doped MIL-125-NH2 coupled Ce4+/Ce3+ and Ti4+/Ti3+ redox mediators for thermo-enhanced photocatalytic oxidative desulfurization, Chin. Chem. Lett., 2023, 34, 107766 CrossRef CAS.
- Y. Liu, S. Pujals, P. J. M. Stals, T. Paulöhrl, S. I. Presolski, E. W. Meijer, L. Albertazzi and A. R. A. Palmans, Catalytically Active Single-Chain Polymeric Nanoparticles: Exploring Their Functions in Complex Biological Media, J. Am. Chem. Soc., 2018, 140, 3423–3433 CrossRef CAS PubMed.
- L. Y. Wu, Y. F. Mu, X. X. Guo, W. Zhang, Z. M. Zhang, M. Zhang and T. B. Lu, Encapsulating Perovskite Quantum Dots in Iron–Based Metal–Organic Frameworks (MOFs) for Efficient Photocatalytic CO2 Reduction, Angew. Chem., Int. Ed., 2019, 58, 9491–9495 CrossRef CAS PubMed.
- J. A. Johnson, J. Luo, X. Zhang, Y.-S. Chen, M. D. Morton, E. Echeverría, F. E. Torres and J. Zhang, Porphyrin-Metalation-Mediated Tuning of Photoredox Catalytic Properties in Metal–Organic Frameworks, ACS Catal., 2015, 5, 5283–5291 CrossRef CAS.
- T. Araya, M. Jia, J. Yang, P. Zhao, K. Cai, W. Ma and Y. Huang, Resin modified MIL-53 (Fe) MOF for improvement of photocatalytic performance, Appl. Catal., B, 2017, 203, 768–777 CrossRef CAS.
- Q. Wu, M. S. Siddique, Y. Yang, M. Wu, L. Kang and H. Yang, Facile and scalable synthesis of Fe-based metal organic frameworks for highly efficient photo-Fenton degradation of organic contaminants, J. Cleaner Prod., 2022, 374, 134033 CrossRef CAS.
- L. Li, Z.-B. Fang, W. Deng, J.-D. Yi, R. Wang and T.-F. Liu, Precise Construction of Stable Bimetallic Metal–Organic Frameworks with Single-Site Ti(IV) Incorporation in Nodes for Efficient Photocatalytic Oxygen Evolution, CCS Chem., 2022, 4, 2782–2792 CrossRef CAS.
- Y. J. Zhang, F. X. Mao, Y. W. Liu, X. F. Wu, C. F. Wen, S. Dai, P. F. Liu and H. G. Yang, Installation of high-valence tungsten in MIL-125(Ti) for boosted photocatalytic hydrogen evolution, Sci. China Mater., 2022, 65, 1237–1244 CrossRef CAS.
- C. Xu, Y. Pan, G. Wan, H. Liu, L. Wang, H. Zhou, S.-H. Yu and H.-L. Jiang, Turning on Visible-Light Photocatalytic C−H Oxidation over Metal–Organic Frameworks by Introducing Metal-to-Cluster Charge Transfer, J. Am. Chem. Soc., 2019, 141, 19110–19117 CrossRef CAS PubMed.
- R. Li, T. Chen, J. Lu, H. Hu, H. Zheng, P. Zhu and X. Pan, Metal–organic frameworks doped with metal ions for efficient sterilization: Enhanced photocatalytic activity and photothermal effect, Water Res., 2023, 229, 119366 CrossRef CAS PubMed.
- J. Li, H. Huang, W. Xue, K. Sun, X. Song, C. Wu, L. Nie, Y. Li, C. Liu, Y. Pan, H.-L. Jiang, D. Mei and C. Zhong, Self-adaptive dual-metal-site pairs in metal-organic frameworks for selective CO2 photoreduction to CH4, Nat. Catal., 2021, 4, 719–729 CrossRef CAS.
- J. Zhang, Y. Wang, H. Wang, D. Zhong and T. Lu, Enhancing photocatalytic performance of metal-organic frameworks for CO2 reduction by a bimetallic strategy, Chin. Chem. Lett., 2022, 33, 2065–2068 CrossRef CAS.
- Y.-L. Dong, H.-R. Liu, S.-M. Wang, G.-W. Guan and Q.-Y. Yang, Immobilizing Isatin-Schiff Base Complexes in NH2-UiO-66 for Highly Photocatalytic CO2 Reduction, ACS Catal., 2023, 13, 2547–2554 CrossRef CAS.
- A. Kuila and P. Saravanan, Intramolecular orbital engineered hetero bimetallic Ce-Fe MOF with reduced transition energy and enhanced visible light property, Appl. Organomet. Chem., 2020, 34, e5728 CrossRef CAS.
- X. Zhang, X. Ma, Y. Ye, C. Guo, X. Xu, J. Zhou and B. Wang, Enhanced photocatalytic hydrogen evolution with a Mixed-Valence iron Metal-Organic framework, Chem. Eng. J., 2023, 456, 140939 CrossRef CAS.
- Z. Wei, S. Song, H. Gu, Y. Li, Q. Sun, N. Ding, H. Tang, L. Zheng, S. Liu, Z. Li, W. Chen, S. Li and S. Pang, Enhancing the Photocatalytic Activity of Zirconium–Based Metal–Organic Frameworks Through the Formation of Mixed–Valence Centers, Adv. Sci., 2023, 10, 2303206 CrossRef CAS PubMed.
- G. Yang, D. Wang, Y. Wang, W. Hu, S. Hu, J. Jiang, J. Huang and H.-L. Jiang, Modulating the Primary and Secondary Coordination Spheres of Single Ni(II) Sites in Metal–Organic Frameworks for Boosting Photocatalysis, J. Am. Chem. Soc., 2024, 146, 10798–10805 CrossRef CAS PubMed.
- X. Y. Dong, Y. B. Si, J. S. Yang, C. Zhang, Z. Han, P. Luo, Z. Y. Wang, S. Q. Zang and T. C. W. Mak, Ligand engineering to achieve enhanced ratiometric oxygen sensing in a silver cluster-based metal-organic framework, Nat. Commun., 2020, 11, 3678–3686 CrossRef CAS PubMed.
- J. Liang, H. Yu, J. Shi, B. Li, L. Wu and M. Wang, Dislocated Bilayer MOF Enables High-Selectivity Photocatalytic Reduction of CO2 to CO, Adv. Mater., 2023, 35, 2209814 CrossRef CAS PubMed.
- W. Wang, H. Liu, Z. Huang, F. Fu, W. Wang, L. Wu, Y. Huang, C. Wu and X. Pan, The effect of organic ligand modification on protein corona formation of nanoscale metal organic frameworks, Chin. Chem. Lett., 2022, 33, 4185–4190 CrossRef CAS.
- S. P. Tripathy, S. Subudhi, A. Ray, P. Behera, J. Panda, S. Dash and K. Parida, Hydrolytically stable mixed ditopic linker based zirconium metal organic framework as a robust photocatalyst towards Tetracycline Hydrochloride degradation and hydrogen evolution, J. Colloid Interface Sci., 2023, 629, 705–718 CrossRef CAS PubMed.
- S. Naghdi, A. Cherevan, A. Giesriegl, R. Guillet-Nicolas, S. Biswas, T. Gupta, J. Wang, T. Haunold, B. C. Bayer, G. Rupprechter, M. C. Toroker, F. Kleitz and D. Eder, Selective ligand removal to improve accessibility of active sites in hierarchical MOFs for heterogeneous photocatalysis, Nat. Commun., 2022, 13, 282–293 CrossRef CAS PubMed.
- H. Zhao, Q. Xia, H. Xing, D. Chen and H. Wang, Construction of Pillared-Layer MOF as Efficient Visible-Light Photocatalysts for Aqueous Cr(VI) Reduction and Dye Degradation, ACS Sustainable Chem. Eng., 2017, 5, 4449–4456 CrossRef CAS.
- F. Leng, H. Liu, M. Ding, Q.-P. Lin and H.-L. Jiang, Boosting Photocatalytic Hydrogen Production of Porphyrinic MOFs: The Metal Location in Metalloporphyrin Matters, ACS Catal., 2018, 8, 4583–4590 CrossRef CAS.
- Z.-B. Fang, T.-T. Liu, J. Liu, S. Jin, X.-P. Wu, X.-Q. Gong, K. Wang, Q. Yin, T.-F. Liu, R. Cao and H.-C. Zhou, Boosting Interfacial Charge-Transfer Kinetics for Efficient Overall CO2 Photoreduction via Rational Design of Coordination Spheres on Metal–Organic Frameworks, J. Am. Chem. Soc., 2020, 142, 12515–12523 CrossRef CAS PubMed.
- D. Bai, J. Qiu, J. Li, S. Zhou, X. Cui, X. Tang, Y. Tang, W. Liu and B. Chen, Mesoporous Mixed-Metal–Organic Framework Incorporating a [Ru(Phen)3]2+ Photosensitizer for Highly Efficient Aerobic Photocatalytic Oxidative Coupling of Amines, ACS Appl. Mater. Interfaces, 2023, 15, 30320–30331 CrossRef CAS PubMed.
- F. Mohammadnezhad, S. Kampouri, S. K. Wolff, Y. Xu, M. Feyzi, J.-H. Lee, X. Ji and K. C. Stylianou, Tuning the Optoelectronic Properties of Hybrid Functionalized MIL-125-NH2 for Photocatalytic Hydrogen Evolution, ACS Appl. Mater. Interfaces, 2021, 13, 5044–5051 CrossRef CAS PubMed.
- Y. Yin, R. Lv, W. Zhang, J. Lu, Y. Ren, X. Li, L. Lv, M. Hua and B. Pan, Exploring mechanisms of different active species formation in heterogeneous Fenton systems by regulating iron chemical environment, Appl. Catal., B, 2021, 295, 120282 CrossRef CAS.
- F. Vermoortele, M. Vandichel, B. Van de Voorde, R. Ameloot, M. Waroquier, V. Van Speybroeck and D. E. De Vos, Electronic Effects of Linker Substitution on Lewis Acid Catalysis with Metal–Organic Frameworks, Angew. Chem., Int. Ed., 2012, 51, 4887–4890 CrossRef CAS PubMed.
- Y.-P. Zhu, J. Yin, E. Abou-Hamad, X. Liu, W. Chen, T. Yao, O. F. Mohammed and H. N. Alshareef, Highly Stable Phosphonate-Based MOFs with Engineered Bandgaps for Efficient Photocatalytic Hydrogen Production, Adv. Mater., 2020, 32, 1906368 CrossRef CAS PubMed.
- T. Wang, Y. Wang, M. Sun, A. Hanif, H. Wu, Q. Gu, Y. S. Ok, D. C. W. Tsang, J. Li, J. Yu and J. Shang, Thermally treated zeolitic imidazolate framework-8 (ZIF-8) for visible light photocatalytic degradation of gaseous formaldehyde, Chem. Sci., 2020, 11, 6670–6681 RSC.
- Y. Pan, J. Wang, S. Chen, W. Yang, C. Ding, A. Waseem and H.-L. Jiang, Linker engineering in metal–organic frameworks for dark photocatalysis, Chem. Sci., 2022, 13, 6696–6703 RSC.
- J. Chen, C. Qin, Y. Mou, Y. Cao, H. Chen, X. Yuan and H. Wang, Linker regulation of iron-based MOFs for highly effective Fenton-like degradation of refractory organic contaminants, Chem. Eng. J., 2023, 459, 141588 CrossRef CAS.
- R. Liang, R. Huang, X. Wang, S. Ying, G. Yan and L. Wu, Functionalized MIL-68(In) for the photocatalytic treatment of Cr(VI)-containing simulation wastewater: Electronic effects of ligand substitution, Appl. Surf. Sci., 2019, 464, 396–403 CrossRef CAS.
- S. Wang, Z. Ai, X. Niu, W. Yang, R. Kang, Z. Lin, A. Waseem, L. Jiao and H. L. Jiang, Linker Engineering of Sandwich–Structured Metal–Organic Framework Composites for Optimized Photocatalytic H2 Production, Adv. Mater., 2023, 35, 2302512 CrossRef CAS PubMed.
- C. Liu, H. Liu, J. C. Yu, L. Wu and Z. Li, Strategies to engineer metal-organic frameworks for efficient photocatalysis, Chin. J. Catal., 2023, 55, 1–19 CrossRef CAS.
- S. Mao, Y. Zou, G. Sun, L. Zeng, Z. Wang, D. Ma, Y. Guo, Y. Cheng, C. Wang and J.-W. Shi, Thio linkage between CdS quantum dots and UiO-66-type MOFs as an effective transfer bridge of charge carriers boosting visible-light-driven photocatalytic hydrogen production, J. Colloid Interface Sci., 2021, 581, 1–10 CrossRef CAS PubMed.
- S. Mao, J.-W. Shi, G. Sun, Y. Zhang, X. Ji, Y. Lv, B. Wang, Y. Xu and Y. Cheng, Cu(II) decorated thiol-functionalized MOF as an efficient transfer medium of charge carriers promoting photocatalytic hydrogen evolution, Chem. Eng. J., 2021, 404, 126533 CrossRef CAS.
- K. Wu, X.-Y. Liu, P.-W. Cheng, Y.-L. Huang, J. Zheng, M. Xie, W. Lu and D. Li, Linker Engineering for Reactive Oxygen Species Generation Efficiency in Ultra-Stable Nickel-Based Metal–Organic Frameworks, J. Am. Chem. Soc., 2023, 145, 18931–18938 CrossRef CAS PubMed.
- W.-J. Xu, B.-X. Huang, G. Li, F. Yang, W. Lin, J.-X. Gu, H.-G. Deng, Z.-G. Gu and H.-G. Jin, Donor–Acceptor Mixed-Naphthalene Diimide-Porphyrin MOF for Boosting Photocatalytic Oxidative Coupling of Amines, ACS Catal., 2023, 13, 5723–5732 CrossRef CAS.
- H. A. Beejapur, Q. Zhang, K. Hu, L. Zhu, J. Wang and Z. Ye, TEMPO in Chemical Transformations: From Homogeneous to Heterogeneous, ACS Catal., 2019, 9, 2777–2830 CrossRef CAS.
- Z.-H. Wang, P.-S. Gao, X. Wang, J.-Q. Gao, X.-T. Xu, Z. He, C. Ma and T.-S. Mei, TEMPO-Enabled Electrochemical Enantioselective Oxidative Coupling of Secondary Acyclic Amines with Ketones, J. Am. Chem. Soc., 2021, 143, 15599–15605 CrossRef CAS PubMed.
- N. O. Balayeva, N. Zheng, R. Dillert and D. W. Bahnemann, Visible-Light-Mediated Photocatalytic Aerobic Dehydrogenation of N-heterocycles by Surface-Grafted TiO2 and 4-amino-TEMPO, ACS Catal., 2019, 9, 10694–10704 CrossRef CAS.
- P. Asselin and P. D. Harvey, Visible-Light-Driven Production of Solar Fuels Catalyzed by Nanosized Porphyrin-Based Metal–Organic Frameworks and Covalent–Organic Frameworks: A Review, ACS Appl. Nano Mater., 2022, 5, 6055–6082 CrossRef CAS.
- S. Shang, W. Xiong, C. Yang, B. Johannessen, R. Liu, H.-Y. Hsu, Q. Gu, M. K. H. Leung and J. Shang, Atomically Dispersed Iron Metal Site in a Porphyrin-Based Metal–Organic Framework for Photocatalytic Nitrogen Fixation, ACS Nano, 2021, 15, 9670–9678 CrossRef CAS PubMed.
- Z. Wang, Z. Liu, J. Huang, Y. Chen, R. Su, J. He, G. Lv, B. Gao, W. Zhou, Y. Wang, Z. Wang and Q. Li, Zr6O8-porphyrinic MOFs as promising catalysts for the boosting photocatalytic degradation of contaminants in high salinity wastewater, Chem. Eng. J., 2022, 440, 135883 CrossRef CAS.
- C. Chen, Q. Mo, J. Fu, Q. Yang, L. Zhang and C.-Y. Su, PtCu@Ir-PCN-222: Synergistic Catalysis of Bimetallic PtCu Nanowires in Hydrosilane-Concentrated Interspaces of an Iridium(III)–Porphyrin-Based Metal–Organic Framework, ACS Catal., 2022, 12, 3604–3614 CrossRef CAS.
- X. J. Kong, T. He, J. Zhou, C. Zhao, T. C. Li, X. Q. Wu, K. Wang and J. R. Li, In Situ Porphyrin Substitution in a Zr(IV)–MOF for Stability Enhancement and Photocatalytic CO2 Reduction, Small, 2021, 17, 2005357 CrossRef CAS PubMed.
- T. Ma, K. Li, J. Hu, Y. Xin, J. Cao, J. He and Z. Xu, Carbazole-Equipped Metal–Organic Framework for Stability, Photocatalysis, and Fluorescence Detection, Inorg. Chem., 2022, 61, 14352–14360 CrossRef CAS PubMed.
- J. Bai, J. Wang, H. Zheng, X. Zhao, P. Wu, L. Pei and J. Wang, Modulating Photoinduced Electron Transfer between Photosensitive MOF and Co(II) Proton Reduction Sites for Boosting Photocatalytic Hydrogen Production, Small, 2023, 19, 2305024 CrossRef CAS PubMed.
- S.-D. Wang, C.-L. Lai, Y.-X. Zhang, S.-T. Bao, K.-L. Lv and L.-L. Wen, Effective charge and energy transfers within a metal–organic framework for efficient photocatalytic oxidation of amines and sulfides, J. Mater. Chem. A, 2022, 10, 20975–20983 RSC.
- X. Xin, Z. Zhao, Y. Chen, J. Tan, Y. Shi, H. Ren, D. Yang and Z. Jiang, Dual-Ligand Ti-MOFs with Push–Pull Effect for Photocatalytic H2 Production, ACS Appl. Mater. Interfaces, 2022, 15, 1053–1062 CrossRef PubMed.
- L. Zeng, X. Guo, C. He and C. Duan, Metal–Organic Frameworks: Versatile Materials for Heterogeneous Photocatalysis, ACS Catal., 2016, 6, 7935–7947 CrossRef CAS.
- Y. Chen, W. Lu, M. Schröder and S. Yang, Analysis and Refinement of Host–Guest Interactions in Metal–Organic Frameworks, Acc. Chem. Res., 2023, 56, 2569–2581 CrossRef CAS PubMed.
- X. Yang, T. Liang, J. Sun, M. J. Zaworotko, Y. Chen, P. Cheng and Z. Zhang, Template-Directed Synthesis of Photocatalyst-Encapsulating Metal–Organic Frameworks with Boosted Photocatalytic Activity, ACS Catal., 2019, 9, 7486–7493 CrossRef CAS.
- A. M. Idris, X. Jiang, J. Tan, Z. Cai, X. Lou, J. Wang and Z. Li, Dye-Sensitized Fe-MOF nanosheets as Visible-Light driven photocatalyst for high efficient photocatalytic CO2 reduction, J. Colloid Interface Sci., 2022, 607, 1180–1188 CrossRef PubMed.
- J. Jin, S. Wan, S. Lee, C. Oh, G. Y. Jang, K. Zhang, Z. Lu and J. H. Park, Tailoring the Nanoporosity and Photoactivity of Metal–Organic Frameworks With Rigid Dye Modulators for Toluene Purification, Small, 2023, 19, 2302776 CrossRef CAS PubMed.
- L. Zhao, Z. Du, G. Ji, Y. Wang, W. Cai, C. He and C. Duan, Eosin Y-Containing Metal–Organic Framework as a Heterogeneous Catalyst for Direct Photoactivation of Inert C–H Bonds, Inorg. Chem., 2022, 61, 7256–7265 CrossRef CAS PubMed.
- J. Low, J. Yu, M. Jaroniec, S. Wageh and A. A. Al-Ghamdi, Heterojunction Photocatalysts, Adv. Mater., 2017, 29, 1601694 CrossRef PubMed.
- C. Pan, Z. Mao, X. Yuan, H. Zhang, L. Mei and X. Ji, Heterojunction Nanomedicine, Adv. Sci., 2022, 9, 2105747 CrossRef PubMed.
- M. Daniel, G. Mathew, M. Anpo and B. Neppolian, MOF based electrochemical sensors for the detection of physiologically relevant biomolecules: An overview, Coord. Chem. Rev., 2022, 468, 214627 CrossRef CAS.
- P. H. M. Andrade, H. Palhares, C. Volkringer, T. Loiseau, M. Hureau, E. Nunes and A. Moissette, State of the art in visible-light photocatalysis of aqueous pollutants using metal-organic frameworks, J. Photochem. Photobiol., C, 2023, 57, 100635 CrossRef CAS.
- H. Wang, L. Zhang, Z. Chen, J. Hu, S. Li, Z. Wang, J. Liu and X. Wang, Semiconductor heterojunction photocatalysts: design, construction, and photocatalytic performances, Chem. Soc. Rev., 2014, 43, 5234–5244 RSC.
- S. J. A. Moniz, S. A. Shevlin, D. J. Martin, Z.-X. Guo and J. Tang, Visible-light driven heterojunction photocatalysts for water splitting – a critical review, Energy Environ. Sci., 2015, 8, 731–759 RSC.
- Z. Guo, S. Ni, H. Wu, J. Wen, X. Li, T. Tang, M. Li and M. Liu, Designing nitrogen and phosphorus co-doped graphene quantum dots/g-C3N4 heterojunction composites to enhance visible and ultraviolet photocatalytic activity, Appl. Surf. Sci., 2021, 548, 149211 CrossRef CAS.
- L. Zhang and M. Jaroniec, Toward designing semiconductor-semiconductor heterojunctions for photocatalytic applications, Appl. Surf. Sci., 2018, 430, 2–17 CrossRef CAS.
- F. Guo, W. Shi, H. Wang, M. Han, W. Guan, H. Huang, Y. Liu and Z. Kang, Study on highly enhanced photocatalytic tetracycline degradation of type II AgI/CuBi2O4 and Z-scheme AgBr/CuBi2O4 heterojunction photocatalysts, J. Hazard. Mater., 2018, 349, 111–118 CrossRef CAS PubMed.
- J. K. Hyun, S. Zhang and L. J. Lauhon, Nanowire Heterostructures, Annu. Rev. Mater. Res., 2013, 43, 451–479 CrossRef CAS.
- L. Wang, J. Mao, G. Huang, Y. Zhang, J. Huang, H. She, C. Liu, H. Liu and Q. Wang, Configuration of hetero-framework via integrating MOF and triazine-containing COF for charge-transfer promotion in photocatalytic CO2 reduction, Chem. Eng. J., 2022, 446, 137011 CrossRef CAS.
- H. Zhao, Z. Xing, S. Su, S. Song, Z. Li and W. Zhou, Gear-shaped mesoporous NH2-MIL-53(Al)/CdS P-N heterojunctions as efficient visible-light-driven photocatalysts, Appl. Catal., B, 2021, 291, 120106 CrossRef CAS.
- A. J. Bard, Photoelectrochemistry and heterogeneous photo-catalysis at semiconductors, J. Photochem., 1979, 10, 59–75 CrossRef CAS.
- H. Tada, T. Mitsui, T. Kiyonaga, T. Akita and K. Tanaka, All-solid-state Z-scheme in CdS–Au–TiO2 three-component nanojunction system, Nat. Mater., 2006, 5, 782–786 CrossRef CAS PubMed.
- X.-L. Yin, J. Liu, W.-J. Jiang, X. Zhang, J.-S. Hu and L.-J. Wan, Urchin-like Au@CdS/WO3 micro/nano heterostructure as a visible-light driven photocatalyst for efficient hydrogen generation, Chem. Commun., 2015, 51, 13842–13845 RSC.
- J. Yu, S. Wang, J. Low and W. Xiao, Enhanced photocatalytic performance of direct Z-scheme g-C3N4–TiO2 photocatalysts for the decomposition of formaldehyde in air, Phys. Chem. Chem. Phys., 2013, 15, 16883–16890 RSC.
- X. Dai, S. Feng, C. Ma, L. Xu, L. Fan, Z. Ye and Y. Wang, Direct z-scheme ZnCo2S4/MOF-199 constructed by bimetallic sulfide modified MOF for photocatalytic hydrogen evolution, Appl. Surf. Sci., 2023, 639, 158142 CrossRef CAS.
- B. Wu, T. Sun, N. Liu, L. Lu, R. Zhang, W. Shi and P. Cheng, Modulation of Z-Scheme Heterojunction Interface between Ultrathin C3N5 Nanosheets and Metal–Organic Framework for Boosting Photocatalysis, ACS Appl. Mater. Interfaces, 2022, 14, 26742–26751 CrossRef CAS PubMed.
- Q. Xu, L. Zhang, B. Cheng, J. Fan and J. Yu, S-Scheme Heterojunction Photocatalyst, Chem, 2020, 6, 1543–1559 CAS.
- B. Zhu, J. Sun, Y. Zhao, L. Zhang and J. Yu, Construction of 2D S–Scheme Heterojunction Photocatalyst, Adv. Mater., 2023, 36, 2310600 CrossRef PubMed.
- Y. Zhang, P. Wei, Z. Li, Y. Sun, Y. Liu and S. Huang, Advancements in rare earth metal-organic frameworks: Harnessing the power of photonics and beyond, Coord. Chem. Rev., 2024, 514, 215905 CrossRef CAS.
- J. S. Jeyaprakash, M. Rajamani, C. L. Bianchi, M. Ashokkumar and B. Neppolian, Highly efficient ultrasound-driven Cu-MOF/ZnWO4 heterostructure: An efficient visible-light photocatalyst with robust stability for complete degradation of tetracycline, Ultrason. Sonochem., 2023, 100, 106624 CrossRef CAS PubMed.
- B. Liu, J. Cai, J. Zhang, H. Tan, B. Cheng and J. Xu, Simultaneous benzyl alcohol oxidation and H2 generation over MOF/CdS S-scheme photocatalysts and mechanism study, Chin. J. Catal., 2023, 51, 204–215 CrossRef CAS.
- Y. Yang, H. Wei, X. Wang, D. Sun, L. Yu, B. Bai, X. Jing, S. Qin and H. Qian, MOF/COF heterostructure hybrid composite-based molecularly imprinted photoelectrochemical sensing platform for determination of dibutyl phthalate: A further expansion for MOF/COF application, Biosens. Bioelectron., 2023, 223, 115017 CrossRef CAS PubMed.
- Q. Niu, S. Dong, J. Tian, G. Huang, J. Bi and L. Wu, Rational Design of Novel COF/MOF S-Scheme Heterojunction Photocatalyst for Boosting CO2 Reduction at Gas–Solid Interface, ACS Appl. Mater. Interfaces, 2022, 14, 24299–24308 CrossRef CAS PubMed.
- H.-Q. Xu, S. Yang, X. Ma, J. Huang and H.-L. Jiang, Unveiling Charge-Separation Dynamics in CdS/Metal–Organic Framework Composites for Enhanced Photocatalysis, ACS Catal., 2018, 8, 11615–11621 CrossRef CAS.
- X. Li, Q. Deng, L. Zhang, J. Wang, R. Wang, Z. Zeng and S. Deng, Highly efficient hydrogenative ring-rearrangement of furanic aldehydes to cyclopentanone compounds catalyzed by noble metals/MIL-MOFs, Appl. Catal., A, 2019, 575, 152–158 CrossRef CAS.
- L. Jiao and H.-L. Jiang, Metal-Organic-Framework-Based Single-Atom Catalysts for Energy Applications, Chem, 2019, 5, 786–804 CAS.
- M. Xu, D. Li, K. Sun, L. Jiao, C. Xie, C. Ding and H. L. Jiang, Interfacial Microenvironment Modulation Boosting Electron Transfer between Metal Nanoparticles and MOFs for Enhanced Photocatalysis, Angew. Chem., Int. Ed., 2021, 60, 16372–16376 CrossRef CAS PubMed.
- J. D. Xiao, Q. Shang, Y. Xiong, Q. Zhang, Y. Luo, S. H. Yu and H. L. Jiang, Boosting Photocatalytic Hydrogen Production of a Metal-Organic Framework Decorated with Platinum Nanoparticles: The Platinum Location Matters, Angew. Chem., Int. Ed., 2016, 55, 9389–9393 CrossRef CAS PubMed.
- L. Li, Y. Zhao, Q. Wang, Z.-Y. Liu, X.-G. Wang, E.-C. Yang and X.-J. Zhao, Boosting photocatalytic hydrogen production activity by a microporous CuII-MOF nanoribbon decorated with Pt nanoparticles, Inorg. Chem. Front., 2021, 8, 3556–3565 RSC.
- M. Cabrero-Antonino, B. Ferrer, H. G. Baldoví and S. Navalón, Toward solar-driven photocatalytic CO2 methanation under continuous flow operation using benchmark MIL-125(Ti)–NH2 supported ruthenium nanoparticles, Chem. Eng. J., 2022, 445, 136426 CrossRef CAS.
- Z. X. Sun, K. Sun, M. L. Gao, Ö. Metin and H. L. Jiang, Optimizing Pt Electronic States through Formation of a Schottky Junction on Non–reducible Metal-Organic Frameworks for Enhanced Photocatalysis, Angew. Chem., Int. Ed., 2022, 61, e202206108 CrossRef CAS PubMed.
- M. Cao, F. Yang, Q. Zhang, J. Zhang, L. Zhang, L. Li, X. Wang and W.-L. Dai, Facile construction of highly efficient MOF-based Pd@UiO-66-NH2@ZnIn2S4 flower-like nanocomposites for visible-light-driven photocatalytic hydrogen production, J. Mater. Sci. Technol., 2021, 76, 189–199 CrossRef CAS.
- J. D. Xiao, L. Han, J. Luo, S. H. Yu and H. L. Jiang, Integration of Plasmonic Effects and Schottky Junctions into Metal–Organic Framework Composites: Steering Charge Flow for Enhanced Visible–Light Photocatalysis, Angew. Chem., Int. Ed., 2018, 57, 1103–1107 CrossRef CAS PubMed.
- D. Li, S. H. Yu and H. L. Jiang, From UV to Near–Infrared Light–Responsive Metal–Organic Framework Composites: Plasmon and Upconversion Enhanced Photocatalysis, Adv. Mater., 2018, 30, 1707377 CrossRef PubMed.
- L.-W. Chen, Y.-C. Hao, Y. Guo, Q. Zhang, J. Li, W.-Y. Gao, L. Ren, X. Su, L. Hu, N. Zhang, S. Li, X. Feng, L. Gu, Y.-W. Zhang, A.-X. Yin and B. Wang, Metal–Organic Framework Membranes Encapsulating Gold Nanoparticles for Direct Plasmonic Photocatalytic Nitrogen Fixation, J. Am. Chem. Soc., 2021, 143, 5727–5736 CrossRef CAS PubMed.
- Y. Pan, Y. Qian, X. Zheng, S.-Q. Chu, Y. Yang, C. Ding, X. Wang, S.-H. Yu and H.-L. Jiang, Precise fabrication of single-atom alloy co-catalyst with optimal charge state for enhanced photocatalysis, Natl. Sci. Rev., 2021, 8, nwaa224 CrossRef CAS PubMed.
- G. Xu, Q.-L. Hong, Y. Sun, M. Liu, H.-X. Zhang and J. Zhang, Anchoring metal ions in amine-functionalized boron imidazolate framework for photocatalytic reduction of CO2, Chin. Chem. Lett., 2022, 33, 2915–2918 CrossRef CAS.
- X. Wang, X. Zhang, R. Pandharkar, J. Lyu, D. Ray, Y. Yang, S. Kato, J. Liu, M. C. Wasson, T. Islamoglu, Z. Li, J. T. Hupp, C. J. Cramer, L. Gagliardi and O. K. Farha, Insights into the Structure–Activity Relationships in Metal–Organic Framework-Supported Nickel Catalysts for Ethylene Hydrogenation, ACS Catal., 2020, 10, 8995–9005 CrossRef CAS.
- X. Fang, Q. Shang, Y. Wang, L. Jiao, T. Yao, Y. Li, Q. Zhang, Y. Luo and H. L. Jiang, Single Pt Atoms Confined into a Metal–Organic Framework for Efficient Photocatalysis, Adv. Mater., 2018, 30, 1705112 CrossRef PubMed.
- L. Ma, J. Xu, Y. Liu, Y. An, Z. Pan, B. Yang, L. Li, T. Hu and B. Lai, Improved degradation of tetracycline by Cu-doped MIL-101(Fe) in a coupled photocatalytic and persulfate oxidation system: Efficiency, mechanism, and degradation pathway, Sep. Purif. Technol., 2023, 305, 122450 CrossRef CAS.
- L. Yin, D. Wang, X. Li, Y. He, X. Liu, Y. Xu and H. Chen, One-pot synthesis of oxygen-vacancy-rich Cu-doped UiO-66 for collaborative adsorption and photocatalytic degradation of ciprofloxacin, Sci. Total Environ., 2022, 815, 151962 CrossRef CAS PubMed.
- X. Ma, H. Liu, W. Yang, G. Mao, L. Zheng and H.-L. Jiang, Modulating Coordination Environment of Single-Atom Catalysts and Their Proximity to Photosensitive Units for Boosting MOF Photocatalysis, J. Am. Chem. Soc., 2021, 143, 12220–12229 CrossRef CAS PubMed.
- H. Wang, X. Zhang, W. Zhang, M. Zhou and H. L. Jiang, Heteroatom–Doped Ag25 Nanoclusters Encapsulated in Metal–Organic Frameworks for Photocatalytic Hydrogen Production, Angew. Chem., Int. Ed., 2024, 63, e202401443 CrossRef CAS PubMed.
- H. Wang, Y. Wu, T. Xiao, X. Yuan, G. Zeng, W. Tu, S. Wu, H. Y. Lee, Y. Z. Tan and J. W. Chew, Formation of quasi-core-shell In2S3/anatase TiO2@metallic Ti3C2Tx hybrids with favorable charge transfer channels for excellent visible-light-photocatalytic performance, Appl. Catal., B, 2018, 233, 213–225 CrossRef CAS.
- Z. Liang, C. Qu, W. Guo, R. Zou and Q. Xu, Pristine Metal–Organic Frameworks and their Composites for Energy Storage and Conversion, Adv. Mater., 2017, 30, 1702891 CrossRef PubMed.
- X. Yang, A. Wolcott, G. Wang, A. Sobo, R. C. Fitzmorris, F. Qian, J. Z. Zhang and Y. Li, Nitrogen-Doped ZnO Nanowire Arrays for Photoelectrochemical Water Splitting, Nano Lett., 2009, 9, 2331–2336 CrossRef CAS PubMed.
- T. Xu, L. Zhang, H. Cheng and Y. Zhu, Significantly enhanced photocatalytic performance of ZnO via graphene hybridization and the mechanism study, Appl. Catal., B, 2011, 101, 382–387 CrossRef CAS.
- S. Chakrabarti and B. Dutta, Photocatalytic degradation of model textile dyes in wastewater using ZnO as semiconductor catalyst, J. Hazard. Mater., 2004, 112, 269–278 CrossRef CAS PubMed.
- D.-L. Huang, C. Wang, P. Xu, G.-M. Zeng, B.-A. Lu, N.-J. Li, C. Huang, C. Lai, M.-H. Zhao, J.-J. Xu and X.-Y. Luo, A coupled photocatalytic-biological process for phenol degradation in the Phanerochaete chrysosporium-oxalate-Fe3O4 system, Int. Biodeterior. Biodegrad., 2015, 97, 115–123 CrossRef CAS.
- S. Subudhi, S. P. Tripathy and K. Parida, Metal oxide integrated metal organic frameworks (MO@MOF): rational design, fabrication strategy, characterization and emerging photocatalytic applications, Inorg. Chem. Front., 2021, 8, 1619–1636 RSC.
- Y. Liu, Z. Liu, D. Huang, M. Cheng, G. Zeng, C. Lai, C. Zhang, C. Zhou, W. Wang, D. Jiang, H. Wang and B. Shao, Metal or metal-containing nanoparticle@MOF nanocomposites as a promising type of photocatalyst, Coord. Chem. Rev., 2019, 388, 63–78 CrossRef CAS.
- J. Qiu, M. Li, J. Xu, X.-F. Zhang and J. Yao, Bismuth sulfide bridged hierarchical Bi2S3/BiOCl@ZnIn2S4 for efficient photocatalytic Cr(VI) reduction, J. Hazard. Mater., 2020, 389, 121858 CrossRef CAS PubMed.
- D. Dai, J. Qiu, G. Xia, Y. Tang, Z. Wu and J. Yao, Competitive coordination initiated one-pot synthesis of core–shell Bi-MOF@BiOX (X = I, Br and Cl) heterostructures for photocatalytic elimination of mixed pollutants, Sep. Purif. Technol., 2023, 316, 123819 CrossRef CAS.
- H. Yang, L. Jia, Q. Zhang, S. Yuan, T. Ohno and B. Xu, Efficient Exciton Dissociation on Ceria Chelated Cerium–Based MOF Isogenous S–Scheme Photocatalyst for Acetaldehyde Purification, Small, 2023, 20, 2308743 CrossRef PubMed.
- L. Cheng, Q. Xiang, Y. Liao and H. Zhang, CdS-Based photocatalysts, Energy Environ. Sci., 2018, 11, 1362–1391 RSC.
- W. Ma, J. Sun, S. Yao, Y. Wang, G. Chen, G. Fan and Y. Li, Synergistic Interplay of Dual–Active–Sites on Metallic Ni–MOFs Loaded with Pt for Thermal–Photocatalytic Conversion of Atmospheric CO2 under Infrared Light Irradiation, Angew. Chem., Int. Ed., 2023, 62, e202313784 CrossRef CAS PubMed.
- Z. Chen, S. Li, Q. Mo, L. Zhang and C.-Y. Su, Dual-functional photocatalysis boosted by electrostatic assembly of porphyrinic metal-organic framework heterojunction composites with CdS quantum dots, Chin. Chem. Lett., 2023, 34, 108196 CrossRef CAS.
- Y. Ren, Y. Li, G. Pan, N. Wang, Y. Xing and Z. Zhang, Recent progress in CdS-based S-scheme photocatalysts, J. Mater. Sci. Technol., 2024, 171, 162–184 CrossRef CAS.
- S. Zhang, X. Ou, Q. Xiang, S. A. C. Carabineiro, J. Fan and K. Lv, Research progress in metal sulfides for photocatalysis: From activity to stability, Chemosphere, 2022, 303, 135085 CrossRef CAS PubMed.
- M.-Y. Qi, Q. Lin, Z.-R. Tang and Y.-J. Xu, Photoredox coupling of benzyl alcohol oxidation with CO2 reduction over CdS/TiO2 heterostructure under visible light irradiation, Appl. Catal., B, 2022, 307, 121158 CrossRef CAS.
- N. Zhang, Z. Xing, Z. Li and W. Zhou, Sulfur vacancy engineering of metal sulfide photocatalysts for solar energy conversion, Chem Catal., 2023, 3, 100375 CrossRef CAS.
- K. Gao, H. J. Li, Q. Meng, J. Wu and H. W. Hou, Efficient and Selective Visible-Light-Driven Oxidative Coupling of Amines to Imines in Air over CdS@Zr-MOFs, ACS Appl. Mater. Interfaces, 2021, 13, 2779–2787 CrossRef CAS PubMed.
- S. Mao, J.-W. Shi, G. Sun, Y. Zhang, D. Ma, K. Song, Y. Lv, J. Zhou, H. Wang and Y. Cheng, PdS Quantum Dots as a Hole Attractor Encapsulated into the MOF@Cd0.5Zn0.5S Heterostructure for Boosting Photocatalytic Hydrogen Evolution under Visible Light, ACS Appl. Mater. Interfaces, 2022, 14, 48770–48779 CrossRef CAS PubMed.
- M. Xu, C. Sun, X. Zhao, H. Jiang, H. Wang and P. Huo, Fabricated hierarchical CdS/Ni-MOF heterostructure for promoting photocatalytic reduction of CO2, Appl. Surf. Sci., 2022, 576, 151792 CAS.
- W.-Q. Chen, L.-Y. Li, L. Li, W.-H. Qiu, L. Tang, L. Xu, K.-J. Xu and M.-H. Wu, MoS2/ZIF-8 Hybrid Materials for Environmental Catalysis: Solar-Driven Antibiotic-Degradation Engineering, Engineering, 2019, 5, 755–767 CAS.
- D. Roy, S. Neogi and S. De, Mechanistic investigation of photocatalytic degradation of Bisphenol-A using MIL-88A(Fe)/MoS2 Z-scheme heterojunction composite assisted peroxymonosulfate activation, Chem. Eng. J., 2022, 428, 131028 CrossRef CAS.
- S. Z. Butler, S. M. Hollen, L. Cao, Y. Cui, J. A. Gupta, H. R. Gutiérrez, T. F. Heinz, S. S. Hong, J. Huang, A. F. Ismach, E. Johnston-Halperin, M. Kuno, V. V. Plashnitsa, R. D. Robinson, R. S. Ruoff, S. Salahuddin, J. Shan, L. Shi, M. G. Spencer, M. Terrones, W. Windl and J. E. Goldberger, Progress, Challenges, and Opportunities in Two-Dimensional Materials Beyond Graphene, ACS Nano, 2013, 7, 2898–2926 CrossRef CAS PubMed.
- C.-F. Zhang, L.-G. Qiu, F. Ke, Y.-J. Zhu, Y.-P. Yuan, G.-S. Xu and X. Jiang, A novel magnetic recyclable photocatalyst based on a core–shell metal–organic framework Fe3O4@MIL-100(Fe) for the decolorization of methylene blue dye, J. Mater. Chem. A, 2013, 1, 14329–14334 RSC.
- M. Govarthanan, R. Mythili, W. Kim, S. Alfarraj and S. A. Alharbi, Facile fabrication of (2D/2D) MoS2@MIL-88(Fe) interface-driven catalyst for efficient degradation of organic pollutants under visible light irradiation, J. Hazard. Mater., 2021, 414, 125522 CrossRef CAS PubMed.
- K. Sun, M. Liu, J. Pei, D. Li, C. Ding, K. Wu and H. L. Jiang, Incorporating Transition–Metal Phosphides Into Metal–Organic Frameworks for Enhanced Photocatalysis, Angew. Chem., Int. Ed., 2020, 59, 22749–22755 CrossRef CAS PubMed.
- Y.-C. Zhou, P. Wang, H. Fu, C. Zhao and C.-C. Wang, Ternary Ag/Ag3PO4/MIL-125-NH2 Z-scheme heterojunction for boosted photocatalytic Cr(VI) cleanup under visible light, Chin. Chem. Lett., 2020, 31, 2645–2650 CrossRef CAS.
- S. Kampouri, T. N. Nguyen, C. P. Ireland, B. Valizadeh, F. M. Ebrahim, G. Capano, D. Ongari, A. Mace, N. Guijarro, K. Sivula, A. Sienkiewicz, L. Forró, B. Smit and K. C. Stylianou, Photocatalytic hydrogen generation from a visible-light responsive metal–organic framework system: the impact of nickel phosphide nanoparticles, J. Mater. Chem. A, 2018, 6, 2476–2481 RSC.
- A. X. Wang, L. H. Zhang, X. L. Li, Y. Q. Gao, N. Li, G. W. Lu and L. Ge, Synthesis of ternary Ni2P@UiO–66−NH2/Zn0.5Cd0.5S composite materials with significantly improved photocatalytic H2 production performance, Chin. J. Catal., 2022, 43, 1295–1305 CrossRef CAS.
- B. Antil, L. Kumar, M. R. Das and S. Deka, Incorporating NiCoP Cocatalyst into Hollow Rings of ZnCo-Metal–Organic Frameworks to Deliver Pt Cocatalyst like Visible Light Driven Hydrogen Evolution Activity, ACS Appl. Energy Mater., 2022, 5, 11113–11121 CrossRef CAS.
- T. Li and Z. Jin, Unique ternary Ni-MOF-74/Ni2P/MoSx composite for efficient photocatalytic hydrogen production: Role of Ni2P for accelerating separation of photogenerated carriers, J. Colloid Interface Sci., 2022, 605, 385–397 CrossRef CAS PubMed.
- D. L. Long, R. Tsunashima and L. Cronin, Polyoxometalates: Building Blocks for Functional Nanoscale Systems, Angew. Chem., Int. Ed., 2010, 49, 1736–1758 CrossRef CAS PubMed.
- Z. Zhang, Z. Li, Z. Dong, F. Yu, Y. Wang, Y. Wang, X. Cao, Y. Liu and Y. Liu, Synergy of photocatalytic reduction and adsorption for boosting uranium removal with PMo12/UiO-66 heterojunction, Chin. Chem. Lett., 2022, 33, 3577–3580 CrossRef CAS.
- A. Solé-Daura, Y. Benseghir, M.-H. Ha-Thi, M. Fontecave, P. Mialane, A. Dolbecq and C. Mellot-Draznieks, Origin of the Boosting Effect of Polyoxometalates in Photocatalysis: The Case of CO2 Reduction by a Rh-Containing Metal–Organic Framework, ACS Catal., 2022, 12, 9244–9255 CrossRef.
- M. Samaniyan, M. Mirzaei, R. Khajavian, H. Eshtiagh-Hosseini and C. Streb, Heterogeneous Catalysis by Polyoxometalates in Metal–Organic Frameworks, ACS Catal., 2019, 9, 10174–10191 CrossRef CAS.
- X. Yan, J. Xu, T. Zhang, C. Si, J. Jiao, J. Li and Q. Han, Designing polyoxometalate-based metal-organic framework for oxidation of styrene and cycloaddition of CO2 with epoxides, Chin. Chem. Lett., 2023, 34, 107851 CrossRef CAS.
- X. Ma, Q. Ou, J. Yuan, J. Yang, S. Xu and X. Zhang, Multifunctional Fe-doped carbon dots and metal-organic frameworks nanoreactor for cascade degradation and detection of organophosphorus pesticides, Chem. Eng. J., 2023, 464, 142480 CrossRef CAS.
- S. Tao, S. Lu, Y. Geng, S. Zhu, S. A. T. Redfern, Y. Song, T. Feng, W. Xu and B. Yang, Design of Metal–Free Polymer Carbon Dots: A New Class of Room–Temperature Phosphorescent Materials, Angew. Chem., Int. Ed., 2018, 57, 2393–2398 CrossRef CAS PubMed.
- H. Liu, X. Zhong, Q. Pan, Y. Zhang, W. Deng, G. Zou, H. Hou and X. Ji, A review of carbon dots in synthesis strategy, Coord. Chem. Rev., 2024, 498, 215468 CrossRef CAS.
- N. Wang, Y. Wang, T. Guo, T. Yang, M. Chen and J. Wang, Green preparation of carbon dots with papaya as carbon source for effective fluorescent sensing of Iron(III) and Escherichia coli, Biosens. Bioelectron., 2016, 85, 68–75 CrossRef CAS PubMed.
- L. Cao, S. Sahu, P. Anilkumar, C. E. Bunker, J. Xu, K. A. S. Fernando, P. Wang, E. A. Guliants, K. N. Tackett and Y.-P. Sun, Carbon Nanoparticles as Visible-Light Photocatalysts for Efficient CO2 Conversion and Beyond, J. Am. Chem. Soc., 2011, 133, 4754–4757 CrossRef CAS PubMed.
- Y. Yu, Q. Zeng, S. Tao, C. Xia, C. Liu, P. Liu and B. Yang, Carbon Dots Based Photoinduced Reactions: Advances and Perspective, Adv. Sci., 2023, 10, e2207621 CrossRef PubMed.
- J. Qin, Y. Dou, J. Zhou, D. Zhao, T. Orlander, H. R. Andersen, C. Hélix-Nielsen and W. Zhang, Encapsulation of carbon-nanodots into metal-organic frameworks for boosting photocatalytic upcycling of polyvinyl chloride plastic, Appl. Catal., B, 2024, 341, 123355 CrossRef CAS.
- S. Li, K. Ji, M. Zhang, C. He, J. Wang and Z. Li, Boosting the photocatalytic CO2 reduction of metal–organic frameworks by encapsulating carbon dots, Nanoscale, 2020, 12, 9533–9540 RSC.
- C. Zheng, X. Qiu, J. Han, Y. Wu and S. Liu, Zero-Dimensional-g-CNQD-Coordinated Two-Dimensional Porphyrin MOF Hybrids for Boosting Photocatalytic CO2 Reduction, ACS Appl. Mater. Interfaces, 2019, 11, 42243–42249 CrossRef CAS PubMed.
- Y. Zhang, M. Sun, M. Peng, E. Du, X. Xu and C.-C. Wang, The fabrication strategies and enhanced performances of metal-organic frameworks and carbon dots composites: State of the art review, Chin. Chem. Lett., 2023, 34, 107478 CrossRef CAS.
- J. Kim, H. Lee, J.-Y. Lee, K.-H. Park, W. Kim, J. H. Lee, H.-J. Kang, S. W. Hong, H.-J. Park, S. Lee, J.-H. Lee, H.-D. Park, J. Y. Kim, Y. W. Jeong and J. Lee, Photosensitized Production of Singlet Oxygen via C60 Fullerene Covalently Attached to Functionalized Silica-coated Stainless-Steel Mesh: Remote Bacterial and Viral Inactivation, Appl. Catal., B, 2020, 270, 118862 CrossRef CAS.
- L. Liu, H. Meng, Y. Chai, X. Chen, J. Xu, X. Liu, W. Liu, D. M. Guldi and Y. Zhu, Enhancing Built–in Electric Fields for Efficient Photocatalytic Hydrogen Evolution by Encapsulating C60 Fullerene into Zirconium–Based Metal–Organic Frameworks, Angew. Chem., 2023, 135, e202217897 CrossRef.
- X. Wang, K. Maeda, A. Thomas, K. Takanabe, G. Xin, J. M. Carlsson, K. Domen and M. Antonietti, A metal-free polymeric photocatalyst for hydrogen production from water under visible light, Nat. Mater., 2008, 8, 76–80 CrossRef PubMed.
- L. K. Putri, B.-J. Ng, C.-C. Er, W.-J. Ong, W. S. Chang, A. R. Mohamed and S.-P. Chai, Insights on the impact of doping levels in oxygen-doped gC3N4 and its effects on photocatalytic activity, Appl. Surf. Sci., 2020, 504, 144427 CrossRef CAS.
- Y. Zhang, J. Zhou, Q. Feng, X. Chen and Z. Hu, Visible light photocatalytic degradation of MB using UiO-66/g-C3N4 heterojunction nanocatalyst, Chemosphere, 2018, 212, 523–532 CrossRef CAS PubMed.
- H. Sudrajat and S. Hartuti, Structural properties and catalytic activity of a novel ternary CuO/gC3N4/Bi2O3 photocatalyst, J. Colloid Interface Sci., 2018, 524, 227–235 CrossRef CAS PubMed.
- H. Wan, G. F. Guan, R. J. Chen, H. T. Yin, L. Wang, Z. Q. Zhang, J. Ding and J. F. Zhang, Enwrapping g-C3N4 on In2O3 hollow hexagonal tubular for photocatalytic CO2 conversion: Construction, characterization, and Z-scheme mechanism insight, J. Colloid Interface Sci., 2023, 631, 122–132 CrossRef PubMed.
- Z. Wang, M. He, H. Jiang, H. He, J. Qi and J. Ma, Photocatalytic MOF membranes with two-dimensional heterostructure for the enhanced removal of agricultural pollutants in water, Chem. Eng. J., 2022, 435, 133870 CrossRef CAS.
- L. Zhang, X. Zhou, S. Liu, H. Liu, S. Zhu, Y. Mao, Q. Yang, S. Zhu, C. Zhang, T. Wang and C. Wang, Two birds, one stone: Rational design of Bi-MOF/g-C3N4 photocatalyst for effective nitrogen fixation and pollutants degradation, J. Cleaner Prod., 2023, 425, 138912 CrossRef CAS.
- V. Muelas-Ramos, M. J. Sampaio, C. G. Silva, J. Bedia, J. J. Rodriguez, J. L. Faria and C. Belver, Degradation of diclofenac in water under LED irradiation using combined g-C3N4/NH2-MIL-125 photocatalysts, J. Hazard. Mater., 2021, 416, 126199 CrossRef CAS PubMed.
- V. Georgakilas, J. A. Perman, J. Tucek and R. Zboril, Broad Family of Carbon Nanoallotropes: Classification, Chemistry, and Applications of Fullerenes, Carbon Dots, Nanotubes, Graphene, Nanodiamonds, and Combined Superstructures, Chem. Rev., 2015, 115, 4744–4822 CrossRef CAS PubMed.
- N. Liu, W. Huang, X. Zhang, L. Tang, L. Wang, Y. Wang and M. Wu, Ultrathin graphene oxide encapsulated in uniform MIL-88A(Fe) for enhanced visible light-driven photodegradation of RhB, Appl. Catal., B, 2018, 221, 119–128 CrossRef CAS.
- C. Yang, X. You, J. Cheng, H. Zheng and Y. Chen, A novel visible-light-driven In-based MOF/graphene oxide composite photocatalyst with enhanced photocatalytic activity toward the degradation of amoxicillin, Appl. Catal., B, 2017, 200, 673–680 CrossRef CAS.
- W. S. Hummers and R. E. Offeman, Preparation of Graphitic Oxide, J. Am. Chem. Soc., 2002, 80, 1339–1339 CrossRef.
- K. Jayaramulu, S. Mukherjee, D. M. Morales, D. P. Dubal, A. K. Nanjundan, A. Schneemann, J. Masa, S. Kment, W. Schuhmann, M. Otyepka, R. Zbořil and R. A. Fischer, Graphene-Based Metal–Organic Framework Hybrids for Applications in Catalysis, Environmental, and Energy Technologies, Chem. Rev., 2022, 122, 17241–17338 CrossRef CAS PubMed.
- Q. Wu, Y. Liu, H. Jing, H. Yu, Y. Lu, M. Huo and H. Huo, Peculiar synergetic effect of γ-Fe2O3 nanoparticles and graphene oxide on MIL-53 (Fe) for boosting photocatalysis, Chem. Eng. J., 2020, 390, 124615 CrossRef CAS.
- F. Liu, C. Huang, C. X. Liu, R. Shi and Y. Chen, Black Phosphorus–Based Semiconductor Heterojunctions for Photocatalytic Water Splitting, Chem. – Eur. J., 2020, 26, 4449–4460 CrossRef CAS PubMed.
- M. Zhu, S. Kim, L. Mao, M. Fujitsuka, J. Zhang, X. Wang and T. Majima, Metal-Free Photocatalyst for H2 Evolution in Visible to Near-Infrared Region: Black Phosphorus/Graphitic Carbon Nitride, J. Am. Chem. Soc., 2017, 139, 13234–13242 CrossRef CAS PubMed.
- M. Zhu, X. Cai, M. Fujitsuka, J. Zhang and T. Majima, Au/La2Ti2O7 Nanostructures Sensitized with Black Phosphorus for Plasmon–Enhanced Photocatalytic Hydrogen Production in Visible and Near–Infrared Light, Angew. Chem., Int. Ed., 2017, 56, 2064–2068 CrossRef CAS PubMed.
- X. Liu, P. Fan, L. Xiao, J. Weng, Q. Xu and J. Xu, Reduced Ti-MOFs encapsulated black phosphorus with high stability and enhanced photocatalytic activity, J. Energy Chem., 2021, 53, 185–191 CrossRef CAS.
- M. Li, X. Liu, L. Tan, Z. Cui, X. Yang, Z. Li, Y. Zheng, K. W. K. Yeung, P. K. Chu and S. Wu, Noninvasive rapid bacteria-killing and acceleration of wound healing through photothermal/photodynamic/copper ion synergistic action of a hybrid hydrogel, Biomater. Sci., 2018, 6, 2110–2121 RSC.
- X. Xiao, L. Zou, H. Pang and Q. Xu, Synthesis of micro/nanoscaled metal–organic frameworks and their direct electrochemical applications, Chem. Soc. Rev., 2020, 49, 301–331 RSC.
- Y. Zhang, S. Gong, A. R. M. Shaheer, R. Cao and T. Liu, Plasmon-enhanced photocatalytic oxidative coupling of amines in the air using a delicate Ag nanowire@NH2-UiO-66 core-shell nanostructures, Chin. Chem. Lett., 2024, 35, 108587 CrossRef CAS.
- W. Gu, Q. Li, H. Zhu and L. Zou, Facile interface engineering of hierarchical flower spherical-like Bi-metal–organic framework microsphere/Bi2MoO6 heterostructure for high-performance visible–light photocatalytic tetracycline hydrochloride degradation, J. Colloid Interface Sci., 2022, 606, 1998–2010 CrossRef CAS PubMed.
- Y. Yang, Z. Xing, W. Kong, C. Wu, H. Peng, Z. Li and W. Zhou, Metal–organic framework (MOF)-5/CuO@ZnIn2S4 core–shell Z-scheme tandem heterojunctions for improved charge separation and enhanced photocatalytic performance, Nanoscale, 2022, 14, 14741–14749 RSC.
- H. Fan, Y. Jin, K. Liu and W. Liu, One-Step MOF-Templated Strategy to Fabrication of Ce-Doped ZnIn2S4 Tetrakaidecahedron Hollow Nanocages as an Efficient Photocatalyst for Hydrogen Evolution, Adv. Sci., 2022, 9, 2104579 CrossRef CAS PubMed.
- J. Zhang, X. Xing, J. Chu, Z. Li, Q. Zhang, R. Wang and S. Wu, Synthesize ordered macroporous MnO2 and their application as curing agent for polysulfide polymers, J. Nanopart. Res., 2019, 21, 58 CrossRef.
- S. Wu, X. Xing, D. Wang, J. Zhang, J. Chu, C. Yu, Z. Wei, M. Hu, X. Zhang and Z. Li, Highly Ordered Hierarchically Macroporous MIL-125 with High Specific Surface Area for Photocatalytic CO2 Fixation, ACS Sustainable Chem. Eng., 2019, 8, 148–153 CrossRef.
- X. Deng, L. Yang, H. Huang, Y. Yang, S. Feng, M. Zeng, Q. Li and D. Xu, Shape–Defined Hollow Structural Co–MOF–74 and Metal Nanoparticles@Co–MOF–74 Composite through a Transformation Strategy for Enhanced Photocatalysis Performance, Small, 2019, 15, 1902287 CrossRef PubMed.
- R. Bibi, H. Huang, M. Kalulu, Q. Shen, L. Wei, O. Oderinde, N. Li and J. Zhou, Synthesis of Amino-Functionalized Ti-MOF Derived Yolk–Shell and Hollow Heterostructures for Enhanced Photocatalytic Hydrogen Production under Visible Light, ACS Sustainable Chem. Eng., 2018, 7, 4868–4877 CrossRef.
- M. Y. Zhang, J. K. Li, R. Wang, S. N. Zhao, S. Q. Zang and T. C. W. Mak, Construction of Core–Shell MOF@COF Hybrids with Controllable Morphology Adjustment of COF Shell as a Novel Platform for Photocatalytic Cascade Reactions, Adv. Sci., 2021, 8, 2101884 CrossRef CAS PubMed.
- H. Sheng, D. Chen, N. Li, Q. Xu, H. Li, J. He and J. Lu, Urchin-Inspired TiO2@MIL-101 Double-Shell Hollow Particles: Adsorption and Highly Efficient Photocatalytic Degradation of Hydrogen Sulfide, Chem. Mater., 2017, 29, 5612–5616 CrossRef CAS.
- Y. Jiao, Y. Chen, W. Han, S. Liang, W. Li and G. Tian, Multi-channel charge transfer of hierarchical TiO2 nanosheets encapsulated MIL-125(Ti) hollow nanodisks sensitized by ZnSe for efficient CO2 photoreduction, J. Colloid Interface Sci., 2022, 627, 492–502 CrossRef CAS PubMed.
- S. Dissegna, K. Epp, W. R. Heinz, G. Kieslich and R. A. Fischer, Defective Metal–Organic Frameworks, Adv. Mater., 2018, 30, 1704501 CrossRef PubMed.
- M. Taddei, G. M. Schukraft, M. E. A. Warwick, D. Tiana, M. J. McPherson, D. R. Jones and C. Petit, Band gap modulation in zirconium-based metal–organic frameworks by defect engineering, J. Mater. Chem. A, 2019, 7, 23781–23786 RSC.
- Z. Xue, K. Liu, Q. Liu, Y. Li, M. Li, C.-Y. Su, N. Ogiwara, H. Kobayashi, H. Kitagawa, M. Liu and G. Li, Missing-linker metal-organic frameworks for oxygen evolution reaction, Nat. Commun., 2019, 10, 5048 CrossRef PubMed.
- M. Yaseen, J. Li, H. Jiang, M. A. Ahmad, I. Khan, L. Tang, C. Wu, A. Ali and Q. Liu, Efficient structure tuning over the defective modulated zirconium metal organic framework with active coordinate surface for photocatalyst CO2 reduction, J. Colloid Interface Sci., 2024, 653, 370–379 CrossRef CAS PubMed.
- X. Ma, L. Wang, Q. Zhang and H. L. Jiang, Switching on the Photocatalysis of Metal–Organic Frameworks by Engineering Structural Defects, Angew. Chem., Int. Ed., 2019, 58, 12175–12179 CrossRef CAS PubMed.
- K. Baruah, R. Dutta, S. Doley and S. K. Dolui, Grafted polymeric organogel using low molecular weight gelator as an effective medium for expulsion and purification of cationic dyes and organic pollutants from contaminated surface water, Eur. Polym. J., 2023, 195, 112213 CrossRef CAS.
- V. Vinayagam, K. N. Palani, S. Ganesh, S. Rajesh, V. V. Akula, R. Avoodaiappan, O. S. Kushwaha and A. Pugazhendhi, Recent developments on advanced oxidation processes for degradation of pollutants from wastewater with focus on antibiotics and organic dyes, Environ. Res., 2024, 240, 117500 CrossRef CAS PubMed.
- W. Chen, W. Wang, H. Xing, W. Li, H. He, W. Li, P. K. Chu, G. Song, H. Wang and P. Li, Interfacial self-assembled dual-functional nanocomposite films for SERS monitoring of visible-light photocatalytic degradation of organic dye pollutants, Surf. Interfaces, 2023, 38, 102808 CrossRef CAS.
- J. Li, Y. Li, M. Zhu, Q. Mei, X. Tang, Y. Wu, S. Yue, Y. Tang and Q. Wang, Constructing aloe-emodin/FeOOH organic-inorganic heterojunction for synergetic photocatalysis-Fenton eliminating antibiotic pollutants, J. Environ. Chem. Eng., 2023, 11, 109775 CrossRef CAS.
- C. Yu, J. Armengaud, R. A. Blaustein, K. Chen, Z. Ye, F. Xu, J.-C. Gaillard, Z. Qin, Y. Fu, E. M. Hartmann and C. Shen, Antibiotic tolerance and degradation capacity of the organic pollutant-degrading bacterium Rhodococcus biphenylivorans TG9T, J. Hazard. Mater., 2022, 424, 127712 CrossRef CAS PubMed.
- X. Chen, Z. Wang, X. Shen, Y. Zhang, Y. Lou, C. Pan, Y. Zhu and J. Xu, A plasmonic Z-scheme Ag@AgCl/PDI photocatalyst for the efficient elimination of organic pollutants, antibiotic resistant bacteria and antibiotic resistance genes, Appl. Catal., B, 2023, 324, 122220 CrossRef CAS.
- M. Kumar, N. S. Bolan, S. A. Hoang, A. D. Sawarkar, T. Jasemizad, B. Gao, S. Keerthanan, L. P. Padhye, L. Singh, S. Kumar, M. Vithanage, Y. Li, M. Zhang, M. B. Kirkham, A. Vinu and J. Rinklebe, Remediation of soils and sediments polluted with polycyclic aromatic hydrocarbons: To immobilize, mobilize, or degrade?, J. Hazard. Mater., 2021, 420, 126534 CrossRef CAS PubMed.
- H. Schroeder, Developmental Brain and Behavior Toxicity of Air Pollutants: A Focus on the Effects of Polycyclic Aromatic Hydrocarbons (PAHs), Crit. Rev. Environ. Sci. Technol., 2011, 41, 2026–2047 CrossRef CAS.
- R. Han, Q. Yu, Y. Zheng, H. Li, Y. Shi, X. Lin and D. Li, Enhanced reduction of Cr(VI) in UV/EKR system by organic acids: Focus on Cr(VI) desorption and Fe(III) catalysis, Sep. Purif. Technol., 2024, 334, 126006 CrossRef CAS.
- Y.-K. Zhang, Y.-Z. Zhang, C.-X. Jia, F. Wang, X. Zhang, Y. Wu, Z. Liu, H. Hu, D.-S. Zhang, L. Geng, J. Xu and H. Huang, A stable Zn-MOF with anthracene-based linker for Cr(VI) photocatalytic reduction under sunlight irradiation, Chin. Chem. Lett., 2024, 109756, DOI:10.1016/j.cclet.2024.109756.
- M.-C. Danner, A. Robertson, V. Behrends and J. Reiss, Antibiotic pollution in surface fresh waters: Occurrence and effects, Sci. Total Environ., 2019, 664, 793–804 CrossRef CAS PubMed.
- J. Cao, Z.-h. Yang, W.-p. Xiong, Y.-y. Zhou, Y.-r. Peng, X. Li, C.-y. Zhou, R. Xu and Y.-r. Zhang, One-step synthesis of Co-doped UiO-66 nanoparticle with enhanced removal efficiency of tetracycline: Simultaneous adsorption and photocatalysis, Chem. Eng. J., 2018, 353, 126–137 CrossRef CAS.
- O. Baaloudj, I. Assadi, N. Nasrallah, A. El Jery, L. Khezami and A. A. Assadi, Simultaneous removal of antibiotics and inactivation of antibiotic-resistant bacteria by photocatalysis: A review, J. Water Process Eng., 2021, 42, 102089 CrossRef.
- R. Sivakumar and N. Y. Lee, Adsorptive removal of organic pollutant methylene blue using polysaccharide-based composite hydrogels, Chemosphere, 2022, 286, 131890 CrossRef CAS PubMed.
- Y. Chen, R. Sun, W. Yan, M. Wu, Y. Zhou and C. Gao, Antibacterial polyvinyl alcohol nanofiltration membrane incorporated with Cu(OH)2 nanowires for dye/salt wastewater treatment, Sci. Total Environ., 2022, 817, 152897 CrossRef CAS PubMed.
- T. Jiang, B. Wang, B. Gao, N. Cheng, Q. Feng, M. Chen and S. Wang, Degradation of organic pollutants from water by biochar-assisted advanced oxidation processes: Mechanisms and applications, J. Hazard. Mater., 2023, 442, 130075 CrossRef CAS PubMed.
- C. Yuan, W. Cui, Y. Sun, J. Wang, R. Chen, J. Zhang, Y. Zhang and F. Dong, Inhibition of the toxic byproduct during photocatalytic NO oxidation via La doping in ZnO, Chin. Chem. Lett., 2020, 31, 751–754 CrossRef CAS.
- Y. Gong, Y. Ding, Q. Tang, F. Lian, C. Bai, R. Xie, H. Xie and X. Zhao, Plasmonic Ag nanoparticles decorated MIL-101(Fe) for enhanced photocatalytic degradation of bisphenol A with peroxymonosulfate under visible-light irradiation, Chin. Chem. Lett., 2024, 35, 108475 CrossRef CAS.
- L. Shi, X. Zou, T. Wang, D. Wang, M. Fan and Z. Gong, Sunlight photocatalytic degradation of ofloxacin using UiO-66/wood composite photocatalysts, Chin. Chem. Lett., 2022, 33, 442–446 CrossRef CAS.
- S. Tu, A. Liu, H. Zhang, L. Sun, M. Luo, S. Huang, T. Huang and H. Peng, Oxygen vacancy regulating transition mode of MIL-125 to facilitate singlet oxygen generation for photocatalytic degradation of antibiotics, Chin. Chem. Lett., 2024, 109761, DOI:10.1016/j.cclet.2024.109761.
- Q. Liu, H. Qie, Z. Sun, Y. Zhen, L. Wu, Y. Zhao and J. Ma, Elevated degradation of di-n-butyl phthalate by activating peroxymonosulfate over GO CoFe2O4 composites: Synergistic effects and mechanisms, Chin. Chem. Lett., 2023, 34, 108397 CrossRef CAS.
- Y. Li, M. Huang, W.-D. Oh, X. Wu and T. Zhou, Efficient activation of sulfite for reductive-oxidative degradation of chloramphenicol by carbon-supported cobalt ferrite catalysts, Chin. Chem. Lett., 2023, 34, 108247 CrossRef CAS.
- F. Sun, X. Yang, F. Shao, F. Li, Z. Pan, L. Qiao, Z. Xiao and W. Liu,
In situ construction of Co(OH)2 nanoparticles decorated biochar for highly efficient degradation of tetracycline hydrochloride via peracetic acid activation, Chin. Chem. Lett., 2023, 34, 108563 CrossRef CAS.
- M. A. Fox and M. T. Dulay, Heterogeneous photocatalysis, Chem. Rev., 2002, 93, 341–357 CrossRef.
- A. Ibhadon and P. Fitzpatrick, Heterogeneous Photocatalysis: Recent Advances and Applications, Catalysts, 2013, 3, 189–218 CrossRef CAS.
- E. M. Dias and C. Petit, Towards the use of metal–organic frameworks for water reuse: a review of the recent advances in the field of organic pollutants removal and degradation and the next steps in the field, J. Mater. Chem. A, 2015, 3, 22484–22506 RSC.
- C.-C. Wang, J.-R. Li, X.-L. Lv, Y.-Q. Zhang and G. Guo, Photocatalytic organic pollutants degradation in metal–organic frameworks, Energy Environ. Sci., 2014, 7, 2831–2867 RSC.
- T. M. Al-Jadir and F. R. Siperstein, The influence of the pore size in Metal−Organic Frameworks in adsorption and separation of hydrogen sulphide: A molecular simulation study, Microporous Mesoporous Mater., 2018, 271, 160–168 CrossRef CAS.
- S. S. Chen, C. Hu, C.-H. Liu, Y.-H. Chen, T. Ahamad, S. M. Alshehri, P.-H. Huang and K. C. W. Wu, De Novo synthesis of platinum-nanoparticle-encapsulated UiO-66-NH2 for photocatalytic thin film fabrication with enhanced performance of phenol degradation, J. Hazard. Mater., 2020, 397, 122431 CrossRef CAS PubMed.
- C.-C. Chueh, C.-I. Chen, Y.-A. Su, H. Konnerth, Y.-J. Gu, C.-W. Kung and K. C. W. Wu, Harnessing MOF materials in photovoltaic devices: recent advances, challenges, and perspectives, J. Mater. Chem. A, 2019, 7, 17079–17095 RSC.
- J.-X. Tan, Z.-Y. Chen, C. H. Chen, M.-F. Hsieh, A. Y.-C. Lin, S. S. Chen and K. C. W. Wu, Efficient adsorption and photocatalytic degradation of water emerging contaminants through nanoarchitectonics of pore sizes and optical properties of zirconium-based MOFs, J. Hazard. Mater., 2023, 451, 131113 CrossRef CAS PubMed.
- J. He, J. Yang, F. Jiang, P. Liu and M. Zhu, Photo-assisted peroxymonosulfate activation via 2D/2D heterostructure of Ti3C2/g-C3N4 for degradation of diclofenac, Chemosphere, 2020, 258, 127339 CrossRef CAS PubMed.
- Y. Cao, L. Yue, Z. Li, Y. Han, J. Lian, H. Qin and S. He, Construction of Sn-Bi-MOF/Ti3C2 Schottky junction for photocatalysis of tetracycline: Performance and degradation mechanism, Appl. Surf. Sci., 2023, 609, 155191 CrossRef CAS.
- C. Liu, L. Lin, Q. Sun, J. Wang, R. Huang, W. Chen, S. Li, J. Wan, J. Zou and C. Yu, Site-specific growth of MOF-on-MOF heterostructures with controllable nano-architectures: beyond the combination of MOF analogues, Chem. Sci., 2020, 11, 3680–3686 RSC.
- X. G. Wang, L. Xu, M. J. Li and X. Z. Zhang, Construction of Flexible-on-Rigid Hybrid-Phase Metal-Organic Frameworks for Controllable Multi-Drug Delivery, Angew. Chem., Int. Ed., 2020, 59, 18078–18086 CrossRef CAS PubMed.
- Y. Zhao, Y. Zhang, X. Cao, J. Li and X. Hou, Synthesis of MOF on MOF photocatalysts using PCN-134 as seed through epitaxial growth strategy towards nizatidine degradation, Chem. Eng. J., 2023, 465, 143000 CrossRef CAS.
- M. Lin, W. Jiang, T. Zhang, B. Yang, Z. Zhuang and Y. Yu, Ordered CoIII-MOF@CoII-MOF Heterojunction for Highly Efficient Photocatalytic Syngas Production, Small Sci., 2023, 3, 2200085 CrossRef CAS.
- P. Zhang and X. W. Lou, Design of Heterostructured Hollow Photocatalysts for Solar-to-Chemical Energy Conversion, Adv. Mater., 2019, 31, 1900281 CrossRef PubMed.
- D. Dong, C. Yan, J. Huang, N. Lu, P. Wu, J. Wang and Z. Zhang, An electron-donating strategy to guide the construction of MOF photocatalysts toward co-catalyst-free highly efficient photocatalytic H2 evolution, J. Mater. Chem. A, 2019, 7, 24180–24185 RSC.
- J. Wang, W. Liu, G. Luo, Z. Li, C. Zhao, H. Zhang, M. Zhu, Q. Xu, X. Wang, C. Zhao, Y. Qu, Z. Yang, T. Yao, Y. Li, Y. Lin, Y. Wu and Y. Li, Synergistic effect of well-defined dual sites boosting the oxygen reduction reaction, Energy Environ. Sci., 2018, 11, 3375–3379 RSC.
- S. He, C. Zhai, M. Fujitsuka, S. Kim, M. Zhu, R. Yin, L. Zeng and T. Majima, Femtosecond time-resolved diffuse reflectance study on facet engineered charge–carrier dynamics in Ag3PO4 for antibiotics photodegradation, Appl. Catal., B, 2021, 281, 119479 CrossRef CAS.
- C. Dong, W. Fang, Q. Yi and J. Zhang, A comprehensive review on reactive oxygen species (ROS) in advanced oxidation processes (AOPs), Chemosphere, 2022, 308, 136205 CrossRef CAS PubMed.
- H. Sepehrmansourie, H. Alamgholiloo, N. N. Pesyan and M. A. Zolfigol, A MOF-on-MOF strategy to construct double Z-scheme heterojunction for high-performance photocatalytic degradation, Appl. Catal., B, 2023, 321, 122082 CrossRef CAS.
- M. Cheng, G. Zeng, D. Huang, C. Lai, P. Xu, C. Zhang and Y. Liu, Hydroxyl radicals based advanced oxidation processes (AOPs) for remediation of soils contaminated with organic compounds: A review, Chem. Eng. J., 2016, 284, 582–598 CrossRef CAS.
- L. W. Matzek and K. E. Carter, Activated persulfate for organic chemical degradation: A review, Chemosphere, 2016, 151, 178–188 CrossRef CAS PubMed.
- Y. Zhang, J. Zhou, X. Chen, L. Wang and W. Cai, Coupling of heterogeneous advanced oxidation processes and photocatalysis in efficient degradation of tetracycline hydrochloride by Fe-based MOFs: Synergistic effect and degradation pathway, Chem. Eng. J., 2019, 369, 745–757 CrossRef CAS.
- Y. Lin, Y. Zhang and G. Li, Promotion of sulfameter degradation by coupling persulfate and photocatalytic advanced oxidation processes with Fe-doped MOFs, Sep. Purif. Technol., 2022, 282, 119632 CrossRef CAS.
- M. Qian, L. Yang, X. Chen, K. Li, W. Xue, Y. Li, H. Zhao, G. Cao, X. Guan and G. Shen, The treatment of veterinary antibiotics in swine wastewater by biodegradation and Fenton-like oxidation, Sci. Total Environ., 2020, 710, 136299 CrossRef CAS PubMed.
- Y. Qin, L. Zhang and T. An, Hydrothermal Carbon-Mediated Fenton-Like Reaction Mechanism in the Degradation of Alachlor: Direct Electron Transfer from Hydrothermal Carbon to Fe(III), ACS Appl. Mater. Interfaces, 2017, 9, 17115–17124 CrossRef CAS PubMed.
- Z. Fang, Y. Liu, J. Qi, Z.-F. Xu, T. Qi and L. Wang, Establishing a high-speed electron transfer channel via CuS/MIL-Fe heterojunction catalyst for photo-Fenton degradation of acetaminophen, Appl. Catal., B, 2023, 320, 121979 CrossRef CAS.
- K. Vinothkumar and R. G. Balakrishna, One-pot synthesis of NH2-MIL-101(Fe) and α – Fe2O3 composite as efficient heterojunction for multifunctional
photocatalytic membranes: Towards zero waste generation, Appl. Catal., B, 2024, 340, 123199 CrossRef CAS.
- Y. Li, Y. Fang, Z. Cao, N. Li, D. Chen, Q. Xu and J. Lu, Construction of g-C3N4/PDI@MOF heterojunctions for the highly efficient visible light-driven degradation of pharmaceutical and phenolic micropollutants, Appl. Catal., B, 2019, 250, 150–162 CrossRef CAS.
- X.-H. Sun, S.-H. Chen, Q.-Z. Guo, Z.-C. Shen, J. Wu and Z.-X. Du, Convenient recycling and efficient photocatalytic degradation of disinfection by-products by in situ growth MOF on PET, Sep. Purif. Technol., 2023, 324, 124506 CrossRef CAS.
- M. Ahmad, S. Chen, F. Ye, X. Quan, S. Afzal, H. Yu and X. Zhao, Efficient photo-Fenton activity in mesoporous MIL-100(Fe) decorated with ZnO nanosphere for pollutants degradation, Appl. Catal., B, 2019, 245, 428–438 CrossRef CAS.
- F. Zhao, Y. Liu, S. B. Hammouda, B. Doshi, N. Guijarro, X. Min, C.-J. Tang, M. Sillanpää, K. Sivula and S. Wang, MIL-101(Fe)/g-C3N4 for enhanced visible-light-driven photocatalysis toward simultaneous reduction of Cr(VI) and oxidation of bisphenol A in aqueous media, Appl. Catal., B, 2020, 272, 119033 CrossRef CAS.
- K. Vinothkumar, M. S. Jyothi, C. Lavanya, M. Sakar, S. Valiyaveettil and R. G. Balakrishna, Strongly co-ordinated MOF-PSF matrix for selective adsorption, separation and photodegradation of dyes, Chem. Eng. J., 2022, 428, 132561 CrossRef CAS.
- N. Kitchamsetti, C. S. Chakra, A. L. F. De Barros and D. Kim, Development of MOF Based Recyclable Photocatalyst for the Removal of Different Organic Dye Pollutants, Nanomaterials, 2023, 13, 336 CrossRef CAS PubMed.
- S. C. Peter, Reduction of CO2 to Chemicals and Fuels: A Solution to Global Warming and Energy Crisis, ACS Energy Lett., 2018, 3, 1557–1561 CrossRef CAS.
- W. Gao, S. Liang, R. Wang, Q. Jiang, Y. Zhang, Q. Zheng, B. Xie, C. Y. Toe, X. Zhu, J. Wang, L. Huang, Y. Gao, Z. Wang, C. Jo, Q. Wang, L. Wang, Y. Liu, B. Louis, J. Scott, A.-C. Roger, R. Amal, H. He and S.-E. Park, Industrial carbon dioxide capture and utilization: state of the art and future challenges, Chem. Soc. Rev., 2020, 49, 8584–8686 RSC.
- M. D. Doherty, D. C. Grills, J. T. Muckerman, D. E. Polyansky and E. Fujita, Toward more efficient photochemical CO2 reduction: Use of scCO2 or photogenerated hydrides, Coord. Chem. Rev., 2010, 254, 2472–2482 CrossRef CAS.
- K. Sun, Y. Qian and H. L. Jiang, Metal–Organic Frameworks for Photocatalytic Water Splitting and CO2 Reduction, Angew. Chem., Int. Ed., 2023, 62, e202217565 CrossRef CAS PubMed.
- N. Elgrishi, M. B. Chambers, X. Wang and M. Fontecave, Molecular polypyridine-based metal complexes as catalysts for the reduction of CO-2, Chem. Soc. Rev., 2017, 46, 761–796 RSC.
- Y. Yamazaki, H. Takeda and O. Ishitani, Photocatalytic reduction of CO2 using metal complexes, J. Photochem. Photobiol., C, 2015, 25, 106–137 CrossRef CAS.
- Y.-H. Luo, L.-Z. Dong, J. Liu, S.-L. Li and Y.-Q. Lan, From molecular metal complex to metal-organic framework: The CO2 reduction photocatalysts with clear and tunable structure, Coord. Chem. Rev., 2019, 390, 86–126 CrossRef CAS.
- K. Bienkowski, R. Solarska, L. Trinh, J. Widera-Kalinowska, B. Al-Anesi, M. Liu, G. K. Grandhi, P. Vivo, B. Oral, B. Yilmaz and R. Yildirim, Halide Perovskites for Photoelectrochemical Water Splitting and CO(2) Reduction: Challenges and Opportunities, ACS Catal., 2024, 14, 6603–6622 CrossRef CAS PubMed.
- F. Fang, J. Zhang, L. Xiang, C. Chen, N. Feng, Y. Lv, K. Chang and J. Huang, Ordering Bimetallic Cu-Pd Catalysts onto Orderly Mesoporous SrTiO(3)-Crystal Nanotubular Networks for Efficient Carbon Dioxide Photoreduction, Angew. Chem., Int. Ed., 2024, 63, e202405807 CrossRef CAS PubMed.
- S. Wang, J. Wang, Y. Wang, X. Sui, S. Wu, W. Dai, Z. Zhang, Z. Ding and J. Long, Insight into the Selectivity-Determining Step of Various Photocatalytic CO2 Reduction Products by Inorganic Semiconductors, ACS Catal., 2024, 14, 10760–10788 CrossRef CAS.
- Q. Huang, R. Wang, X. Li, T. Liu, A. R. M. Shaheer and R. Cao, The effects of local environment towards yield and selectivity on photocatalytic CO2 reduction catalyzed by metal-organic framework, Chin. Chem. Lett., 2023, 34, 108517 CrossRef CAS.
- S. Yoshino, T. Takayama, Y. Yamaguchi, A. Iwase and A. Kudo, CO2 Reduction Using Water as an Electron Donor over Heterogeneous Photocatalysts Aiming at Artificial Photosynthesis, Acc. Chem. Res., 2022, 55, 966–977 CrossRef CAS PubMed.
- M. E. Mahmoud, H. Audi, A. Assoud, T. H. Ghaddar and M. Hmadeh, Metal–Organic Framework Photocatalyst Incorporating Bis(4′-(4-carboxyphenyl)-terpyridine)ruthenium(II) for Visible-Light-Driven Carbon Dioxide Reduction, J. Am. Chem. Soc., 2019, 141, 7115–7121 CrossRef PubMed.
- D. Mateo, A. Santiago-Portillo, J. Albero, S. Navalón, M. Alvaro and H. García, Long-Term Photostability in Terephthalate Metal-Organic Frameworks, Angew. Chem., Int. Ed., 2019, 58, 17843–17848 CrossRef CAS PubMed.
- F. Guo, Y.-P. Wei, S.-Q. Wang, X.-Y. Zhang, F.-M. Wang and W.-Y. Sun, Pt nanoparticles embedded in flowerlike NH2-UiO-68 for enhanced photocatalytic carbon dioxide reduction, J. Mater. Chem. A, 2019, 7, 26490–26495 RSC.
- C. Candia-Onfray, S. Rojas, M. V. B. Zanoni and R. Salazar, An updated review of metal–organic framework materials in photo(electro)catalytic applications: From CO2 reduction to wastewater treatments, Curr. Opin. Electrochem., 2021, 26, 100669 CrossRef CAS.
- D. Li, M. Kassymova, X. Cai, S.-Q. Zang and H.-L. Jiang, Photocatalytic CO2 reduction over metal-organic framework-based materials, Coord. Chem. Rev., 2020, 412, 213262 CrossRef CAS.
- Y. Xi, X. Zhang, Y. Shen, W. Dong, Z. Fan, K. Wang, S. Zhong and S. Bai, Aspect ratio dependent photocatalytic enhancement of CsPbBr3 in CO2 reduction with two-dimensional metal organic framework as a cocatalyst, Appl. Catal., B, 2021, 297, 120411 CrossRef CAS.
- Y. Kamakura, C. Suppaso, I. Yamamoto, R. Mizuochi, Y. Asai, T. Motohashi, D. Tanaka and K. Maeda, Tin(II)–Based Metal–Organic Frameworks Enabling Efficient, Selective Reduction of CO2 to Formate under Visible Light, Angew. Chem., Int. Ed., 2023, 62, e202305923 CrossRef CAS PubMed.
- H. Dong, X. Zhang, Y. Lu, Y. Yang, Y.-P. Zhang, H.-L. Tang, F.-M. Zhang, Z.-D. Yang, X. Sun and Y. Feng, Regulation of metal ions in smart metal-cluster nodes of metal-organic frameworks with open metal sites for improved photocatalytic CO2 reduction reaction, Appl. Catal., B, 2020, 276, 119173 CrossRef CAS.
- E. X. Chen, M. Qiu, Y. F. Zhang, L. He, Y. Y. Sun, H. L. Zheng, X. Wu, J. Zhang and Q. Lin, Energy Band Alignment and Redox–Active Sites in Metalloporphyrin–Spaced Metal–Catechol Frameworks for Enhanced CO2 Photoreduction, Angew. Chem., Int. Ed., 2021, 61, e202111622 CrossRef PubMed.
- S. Karmakar, S. Barman, F. A. Rahimi and T. K. Maji, Covalent grafting of molecular photosensitizer and catalyst on MOF-808: effect of pore confinement toward visible light-driven CO2 reduction in water, Energy Environ. Sci., 2021, 14, 2429–2440 RSC.
- K. Song, S. Liang, X. Zhong, M. Wang, X. Mo, X. Lei and Z. Lin, Tailoring the crystal forms of the Ni-MOF catalysts for enhanced photocatalytic CO2-to-CO performance, Appl. Catal., B, 2022, 309, 121232 CrossRef CAS.
- W. Yang, H. J. Wang, R. R. Liu, J. W. Wang, C. Zhang, C. Li, D. C. Zhong and T. B. Lu, Tailoring Crystal Facets of Metal–Organic Layers to Enhance Photocatalytic Activity for CO2 Reduction, Angew. Chem., Int. Ed., 2020, 60, 409–414 CrossRef PubMed.
- W.-W. Dong, J. Jia, Y. Wang, J.-R. An, O.-Y. Yang, X.-J. Gao, Y.-L. Liu, J. Zhao and D.-S. Li, Visible-light-driven solvent-free photocatalytic CO2 reduction to CO by Co-MOF/Cu2O heterojunction with superior selectivity, Chem. Eng. J., 2022, 438, 135622 CrossRef CAS.
- J. Du, Y.-Y. Ma, W.-J. Cui, S.-M. Zhang, Z.-G. Han, R.-H. Li, X.-Q. Han, W. Guan, Y.-H. Wang, Y.-Q. Li, Y. Liu, F.-Y. Yu, K.-Q. Wei, H.-Q. Tan, Z.-H. Kang and Y.-G. Li, Unraveling photocatalytic electron transfer mechanism in polyoxometalate-encapsulated metal-organic frameworks for high-efficient CO2 reduction reaction, Appl. Catal., B, 2022, 318, 121812 CrossRef CAS.
- L. Xia, W. Zhou, Y. Xu, Z. Xia, X. Wang, Q. Yang, G. Xie, S. Chen and S. Gao, Ferrocene-boosting Zr-MOFs for efficient photocatalytic CO2 reduction: A trade-off between enhancing LMCT and frustrating Lewis acid, Chem. Eng. J., 2023, 451, 138747 CrossRef CAS.
- X.-Y. Zhang, P. Wang, Y. Zhang, X.-M. Cheng and W.-Y. Sun, Facet-Dependent Photocatalytic Behavior of Fe-soc-MOF for Carbon Dioxide Reduction, ACS Appl. Mater. Interfaces, 2023, 15, 3348–3356 CrossRef CAS PubMed.
- N.-Y. Huang, H. He, S. Liu, H.-L. Zhu, Y.-J. Li, J. Xu, J.-R. Huang, X. Wang, P.-Q. Liao and X.-M. Chen, Electrostatic Attraction-Driven Assembly of a Metal–Organic Framework with a Photosensitizer Boosts Photocatalytic CO2 Reduction to CO, J. Am. Chem. Soc., 2021, 143, 17424–17430 CrossRef CAS PubMed.
- L. Huang, S. Mo, X. Zhao, J. Zhou, X. Zhou, Y. Zhang, M. Fu, Y. Fan, Q. Xie, D. Ye and Y. Chen, Designing multi-layered MOF-on-MOF-transformed core double-shell FeSx@ZnS@CoSx heterojunction for enhanced CO2 photoreduction with water vapor, Chem. Eng. J., 2023, 474, 145740 CrossRef CAS.
- X.-M. Cheng, P. Wang, S.-Q. Wang, J. Zhao and W.-Y. Sun, Ti(IV)-MOF with Specific Facet–Ag Nanoparticle Composites for Enhancing the Photocatalytic Activity and Selectivity of CO2 Reduction, ACS Appl. Mater. Interfaces, 2022, 14, 32350–32359 CrossRef CAS PubMed.
- S. Xie, C. Deng, Q. Huang, C. Zhang, C. Chen, J. Zhao and H. Sheng, Facilitated Photocatalytic CO2 Reduction in Aerobic Environment on a Copper–Porphyrin Metal–Organic Framework, Angew. Chem., Int. Ed., 2023, 62, e202216717 CrossRef CAS PubMed.
- L. Wang, P. Jin, S. Duan, H. She, J. Huang and Q. Wang,
In situ incorporation of Copper(II) porphyrin functionalized zirconium MOF and TiO2 for efficient photocatalytic CO2 reduction, Sci. Bull., 2019, 64, 926–933 CrossRef CAS PubMed.
- H. Wu, X. Y. Kong, X. Wen, S. P. Chai, E. C. Lovell, J. Tang and Y. H. Ng, Metal–Organic Framework Decorated Cuprous Oxide Nanowires for Long-lived Charges Applied in Selective Photocatalytic CO2 Reduction to CH4, Angew. Chem., Int. Ed., 2021, 60, 8455–8459 CrossRef CAS PubMed.
- J.-S. Qin, S. Yuan, L. Zhang, B. Li, D.-Y. Du, N. Huang, W. Guan, H. F. Drake, J. Pang, Y.-Q. Lan, A. Alsalme and H.-C. Zhou, Creating Well-Defined Hexabenzocoronene in Zirconium Metal–Organic Framework by Postsynthetic Annulation, J. Am. Chem. Soc., 2019, 141, 2054–2060 CrossRef CAS PubMed.
- G. Wang, C.-T. He, R. Huang, J. Mao, D. Wang and Y. Li, Photoinduction of Cu Single Atoms Decorated on UiO-66-NH2 for Enhanced Photocatalytic Reduction of CO2 to Liquid Fuels, J. Am. Chem. Soc., 2020, 142, 19339–19345 CrossRef CAS PubMed.
- D. E. Canfield, A. N. Glazer and P. G. Falkowski, The Evolution and Future of Earth's Nitrogen Cycle, Science, 2010, 330, 192–196 CrossRef CAS PubMed.
- V. Rosca, M. Duca, M. T. de Groot and M. T. M. Koper, Nitrogen Cycle Electrocatalysis, Chem. Rev., 2009, 109, 2209–2244 CrossRef CAS PubMed.
- B. M. Hoffman, D. Lukoyanov, Z.-Y. Yang, D. R. Dean and L. C. Seefeldt, Mechanism of Nitrogen Fixation by Nitrogenase: The Next Stage, Chem. Rev., 2014, 114, 4041–4062 CrossRef CAS PubMed.
- Y. Tang and G. Wang, NIR light-responsive nanocarriers for controlled release, J. Photochem. Photobiol., C, 2021, 47, 100420 CrossRef CAS.
- Q. Liu, T. Xu, Y. Luo, Q. Kong, T. Li, S. Lu, A. A. Alshehri, K. A. Alzahrani and X. Sun, Recent advances in strategies for highly selective electrocatalytic N2 reduction toward ambient NH3 synthesis, Curr. Opin. Electrochem., 2021, 29, 100766 CrossRef CAS.
- X. Chen, J.-Y. Li, Z.-R. Tang and Y.-J. Xu, Surface-defect-engineered photocatalyst for nitrogen fixation into value-added chemical feedstocks, Catal. Sci. Technol., 2020, 10, 6098–6110 RSC.
- C. Yao, R. Wang, Z. Wang, H. Lei, X. Dong and C. He, Highly dispersive and stable Fe3+ active sites on 2D graphitic carbon nitride nanosheets for efficient visible-light photocatalytic nitrogen fixation, J. Mater. Chem. A, 2019, 7, 27547–27559 RSC.
- H. Liu, P. Wu, H. Li, Z. Chen, L. Wang, X. Zeng, Y. Zhu, Y. Jiang, X. Liao, B. S. Haynes, J. Ye, C. Stampfl and J. Huang, Unravelling the effects of layered supports on Ru nanoparticles for enhancing N2 reduction in photocatalytic ammonia synthesis, Appl. Catal., B, 2019, 259, 118026 CrossRef CAS.
- R. Shi, Y. Zhao, G. I. N. Waterhouse, S. Zhang and T. Zhang, Defect Engineering in Photocatalytic Nitrogen Fixation, ACS Catal., 2019, 9, 9739–9750 CrossRef CAS.
- J. Armijo and C. Philibert, Flexible production of green hydrogen and ammonia from variable solar and wind energy: Case study of Chile and Argentina, Int. J. Hydrogen Energy, 2020, 45, 1541–1558 CrossRef CAS.
- R. D. Milton and S. D. Minteer, Nitrogenase Bioelectrochemistry for Synthesis Applications, Acc. Chem. Res., 2019, 52, 3351–3360 CrossRef CAS PubMed.
- Y. Chen, Q. Sun, X. Tu, L. Chen, W. Han, L. Zhang, X. Duan, M. Liu and H. Zheng, Bio-inspired citric acid-based bimetallic photocatalyst for nitrogen fixation from air under ambient conditions, J. Cleaner Prod., 2023, 392, 136314 CrossRef CAS.
- V. Smil, Detonator of the population explosion, Nature, 1999, 400, 415–415 CrossRef CAS.
- G. P. Connor and P. L. Holland, Coordination chemistry insights into the role of alkali metal promoters in dinitrogen reduction, Catal. Today, 2017, 286, 21–40 CrossRef CAS PubMed.
- S. Li, Y. Wu, Q. Liu, B. Li, T. Li, H. Zhao, A. A. Alshehri, K. A. Alzahrani, Y. Luo, L. Li and X. Sun, CuS concave polyhedral superstructures enabled efficient N2 electroreduction to NH3 at ambient conditions, Inorg. Chem. Front., 2021, 8, 3105–3110 RSC.
- T. Li, S. Han, C. Cheng, Y. Wang, X. Du, Y. Yu and B. Zhang, Sulfate–Enabled Nitrate Synthesis from Nitrogen Electrooxidation on a Rhodium Electrocatalyst, Angew. Chem., Int. Ed., 2022, 61, e202204541 CrossRef CAS PubMed.
- J. G. Chen, R. M. Crooks, L. C. Seefeldt, K. L. Bren, R. M. Bullock, M. Y. Darensbourg, P. L. Holland, B. Hoffman, M. J. Janik, A. K. Jones, M. G. Kanatzidis, P. King, K. M. Lancaster, S. V. Lymar, P. Pfromm, W. F. Schneider and R. R. Schrock, Beyond fossil fuel–driven nitrogen transformations, Science, 2018, 360, 873 CAS.
- X.-B. Li, Z.-K. Xin, S.-G. Xia, X.-Y. Gao, C.-H. Tung and L.-Z. Wu, Semiconductor nanocrystals for small molecule activationviaartificial photosynthesis, Chem. Soc. Rev., 2020, 49, 9028–9056 RSC.
- L. P. Camargo, P. R. C. da Silva, A. Batagin-Neto, V. Klobukoski, M. Vidotti and L. H. Dall'Antonia, Over 21.0% faradaic efficiency of ambient ammonia production: Photoelectrocatalytic activity of MOF-235, Appl. Mater. Today, 2022, 28, 101540 CrossRef.
- H. Miyama, N. Fujii and Y. Nagae, Heterogeneous photocatalytic synthesis of ammonia from water and nitrogen, Chem. Phys. Lett., 1980, 74, 523–524 CrossRef CAS.
- Y. Shi, Z. Zhao, D. Yang, J. Tan, X. Xin, Y. Liu and Z. Jiang, Engineering photocatalytic ammonia synthesis, Chem. Soc. Rev., 2023, 52, 6938–6956 RSC.
- Y. Zheng, S. Zhang, J. Guo, R. Shi, J. Yu, K. Li, N. Li, Z. Zhang and Y. Chen, Green and Scalable Fabrication of High–Performance Biocatalysts Using Covalent Organic Frameworks as Enzyme Carriers, Angew. Chem., Int. Ed., 2022, 61, e202208744 CrossRef CAS PubMed.
- X.-Q. Zhan, F.-C. Tsai, L. Xie, K.-D. Zhang, H.-L. Liu, N. Ma, D. Shi and T. Jiang, Ligands-Coordinated Zr-Based MOF for Wastewater Treatment, Nanomaterials, 2018, 8, 655 CrossRef PubMed.
- S. Zhang, R. Wu, C. Mu, Y. Wang, L. Han, Y. Wu and W.-H. Zhu, Conjugated Self-Assembled Monolayer as Stable Hole-Selective Contact for Inverted Perovskite Solar Cells, ACS Mater. Lett., 2022, 4, 1976–1983 CrossRef CAS.
- B. L. Bonnett, E. D. Smith, M. De La Garza, M. Cai, J. V. Haag, J. M. Serrano, H. D. Cornell, B. Gibbons, S. M. Martin and A. J. Morris, PCN-222 Metal–Organic Framework Nanoparticles with Tunable Pore Size for Nanocomposite Reverse Osmosis Membranes, ACS Appl. Mater. Interfaces, 2020, 12, 15765–15773 CrossRef CAS PubMed.
- S. Liu, T. Wang, L. Zhang, C. Wang and H. Pang, Cerium-based metal-organic framework-modified natural mineral vermiculite for photocatalytic nitrogen fixation under visible-light irradiation, Chin. Chem. Lett., 2024, 110058, DOI:10.1016/j.cclet.2024.110058.
- H. Huang, X.-S. Wang, D. Philo, F. Ichihara, H. Song, Y. Li, D. Li, T. Qiu, S. Wang and J. Ye, Toward visible-light-assisted photocatalytic nitrogen fixation: A titanium metal organic framework with functionalized ligands, Appl. Catal., B, 2020, 267, 118686 CrossRef CAS.
- K. An, H. J. Ren, D. Yang, Z. F. Zhao, Y. C. Gao, Y. Chen, J. D. Tan, W. J. Wang and Z. Y. Jiang, Nitrogenase-inspired bimetallic metal organic frameworks for visible-light-driven nitrogen fixation, Appl. Catal., B, 2021, 292, 120167 CrossRef CAS.
- W. Gao, X. Li, X. Zhang, S. Su, S. Luo, R. Huang, Y. Jing and M. Luo, Photocatalytic nitrogen fixation of metal–organic frameworks (MOFs) excited by ultraviolet light: insights into the nitrogen fixation mechanism of missing metal cluster or linker defects, Nanoscale, 2021, 13, 7801–7809 RSC.
- G. Ren, J. Zhao, Z. Zhao, Z. Li, L. Wang, Z. Zhang, C. Li and X. Meng, Defects-Induced Single-Atom Anchoring on Metal-Organic Frameworks for High-Efficiency Photocatalytic Nitrogen Reduction, Angew. Chem., Int. Ed., 2023, 63, e202314408 CrossRef PubMed.
- Z. Zhao, D. Yang, H. Ren, K. An, Y. Chen, Z. Zhou, W. Wang and Z. Jiang, Nitrogenase-inspired mixed-valence MIL-53(FeII/FeIII) for photocatalytic nitrogen fixation, Chem. Eng. J., 2020, 400, 125929 CrossRef CAS.
- L. Chen, Y. Chen, X. Tu, S. Zhu, C. Sun, L. Zhang, W. Han, X. Duan, Q. Sun and H. Zheng, Fe/Zr-MOFs constructed by a sunlight-responsive ligand for efficient photocatalytic nitrogen fixation under ambient condition, J. Colloid Interface Sci., 2023, 633, 703–711 CrossRef CAS PubMed.
- Y. Sun, H. Ji, Y. Sun, G. Zhang, H. Zhou, S. Cao, S. Liu, L. Zhang, W. Li, X. Zhu and H. Pang, Synergistic Effect of Oxygen Vacancy and High Porosity of Nano MIL–125(Ti) for Enhanced Photocatalytic Nitrogen Fixation, Angew. Chem., Int. Ed., 2023, 63, e202316973 CrossRef PubMed.
- S. Yan, X. Zhang, D. Wu, Y. Yu and Z. Ding, A system investigation on Ru-MOF-74 with efficient photocatalytic nitrogen fixation performance, Surf. Interfaces, 2022, 33, 102225 CrossRef CAS.
- L. Meng, X. Li, W. Ding, M. Tan, S. Su, Y. Cao, Z. Yu, S. Yuan, Y. Hai, Z. Liu and M. Luo, Construction of ZnCdS@CAU-17 heterostructures containing intermediate mediator Bi2S3 as a highly efficient photocatalyst for nitrogen reduction reaction, J. Alloys Compd., 2023, 963, 171253 CrossRef CAS.
- B. Guo, X. Cheng, Y. Tang, W. Guo, S. Deng, L. Wu and X. Fu, Dehydrated UiO-66(SH)2: The Zr-O Cluster and Its Photocatalytic Role Mimicking the Biological Nitrogen Fixation, Angew. Chem., Int. Ed., 2022, 61, e202117244 CrossRef CAS PubMed.
- X. Zhang, X. Li, W. Gao, S. Luo, S. Su, R. Huang and M. Luo, Bimetallic CeZr5-UiO-66 as a highly efficient photocatalyst for the nitrogen reduction reaction, Sustainable Energy Fuels, 2021, 5, 4053–4059 RSC.
- X. H. Li, P. He, T. Wang, X. W. Zhang, W. L. Chen and Y. G. Li, Keggin–Type Polyoxometalate–Based ZIF–67 for Enhanced Photocatalytic Nitrogen Fixation, ChemSusChem, 2020, 13, 2769–2778 CrossRef CAS PubMed.
- Z. Ding, S. Wang, X. Chang, D.-H. Wang and T. Zhang, Nano-MOF@defected film C3N4 Z-scheme composite
for visible-light photocatalytic nitrogen fixation, RSC Adv., 2020, 10, 26246–26255 RSC.
- P. Fageria, K. Y. Sudharshan, R. Nazir, M. Basu and S. Pande, Decoration of MoS2 on g-C3N4 surface for efficient hydrogen evolution reaction, Electrochim. Acta, 2017, 258, 1273–1283 CrossRef CAS.
- G. Jiang, X. Liu, H. Jian, P. Lu, J. Bai, G. Zhang, W. Yun, S. Li and Y. He, Cu-clusters nodes of 2D metal-organic frameworks as a cost-effective noble-metal-free cocatalyst with high atom-utilization efficiency for efficient photocatalytic hydrogen evolution, Chin. Chem. Lett., 2022, 33, 3049–3052 CrossRef CAS.
- X. Liu, C. Jia, G. Jiang, C. Zhang, M. Chen, X. Zhao, X. Zhang, M. Fu, S. Li, J. Wu, Y. Jia and Y. He, Single-atom Pd anchored in the porphyrin-center of ultrathin 2D-MOFs as the active center to enhance photocatalytic hydrogen-evolution and NO-removal, Chin. Chem. Lett., 2023, 109455, DOI:10.1016/j.cclet.2023.109455.
- Q. Li, C. Zhao, S. Jia, Q. Chen, X. Li, M. She, H. Liu, P. Liu, Y. Wang and J. Li, Design and fabrication of CuI/CuII-MOF-incorporated hydrogel photocatalysts for synergy removal of Cr(VI) and congo red, Chin. Chem. Lett., 2024, 109936, DOI:10.1016/j.cclet.2024.109936.
- Y. Li, Y. Liu, Z. Wang, P. Wang, Z. Zheng, H. Cheng, Y. Dai and B. Huang,
In situ growth of Ti3C2@MIL-NH2 composite for highly enhanced photocatalytic H2 evolution, Chem. Eng. J., 2021, 411, 128446 CrossRef CAS.
- Y. Zhang, D. Ma, J. Li, C. Zhi, Y. Zhang, L. Liang, S. Mao and J.-W. Shi, Recent research advances of metal organic frameworks (MOFs) based composites for photocatalytic H2 evolution, Coord. Chem. Rev., 2024, 517, 215995 CrossRef CAS.
- Z. Liang, H. Guo, H. Lei and R. Cao, Co porphyrin-based metal-organic framework for hydrogen evolution reaction and oxygen reduction reaction, Chin. Chem. Lett., 2022, 33, 3999–4002 CrossRef CAS.
- J. Xue, Z. Chen, K. Dang, L. Wu, H. Ji, C. Chen, Y. Zhang and J. Zhao, The plasmonic effect of Cu on tuning CO2 reduction activity and selectivity, Phys. Chem. Chem. Phys., 2024, 26, 2915–2925 RSC.
- L. Lu, B. Wu, W. Shi and P. Cheng, Metal–organic framework-derived heterojunctions as nanocatalysts for photocatalytic hydrogen production, Inorg. Chem. Front., 2019, 6, 3456–3467 RSC.
- Y. Kataoka, K. Sato, Y. Miyazaki, K. Masuda, H. Tanaka, S. Naito and W. Mori, Photocatalytic hydrogen production from water using porous material [Ru2(p-BDC)2]n, Energy Environ. Sci., 2009, 2, 397–400 RSC.
- M. Sun, Q. Q. Wang, C. Qin, C. Y. Sun, X. L. Wang and Z. M. Su, An Amine-Functionalized Zirconium Metal-Organic Polyhedron Photocatalyst with High Visible–Light Activity for Hydrogen Production, Chem. – Eur. J., 2019, 25, 2824–2830 CrossRef CAS PubMed.
- H. Zhang, Q. Li, B. Weng, L. Xiao, Z. Tian, J. Yang, T. Liu and F. Lai, Edge engineering of platinum nanoparticles via porphyrin-based ultrathin 2D metal–organic frameworks for enhanced photocatalytic hydrogen generation, Chem. Eng. J., 2022, 442, 136144 CrossRef CAS.
- R. Djellabi, L. Noureen, V.-D. Dao, D. Meroni, E. Falletta, D. D. Dionysiou and C. L. Bianchi, Recent advances and challenges of emerging solar-driven steam and the contribution of photocatalytic
effect, Chem. Eng. J., 2022, 431, 134024 CrossRef CAS.
- H. Deng, J. Wang, Y. Ouyang, D. Li, Y. Xiao, Q. Zhang and S. Huang, Multiple roles of 2D conductive metal-organic framework enable noble metal-free photocatalytic hydrogen evolution, Appl. Surf. Sci., 2023, 622, 156853 CrossRef CAS.
- S. Zhang, L. Lu, J. Jiang, N. Liu, B. Zhao, M. Xu, P. Cheng and W. Shi, Organizing Photosensitive and Photothermal Single–Sites Uniformly in a Trimetallic Metal–Organic Framework for Efficient Photocatalytic Hydrogen Evolution, Adv. Mater., 2024, 36, 2403464 CrossRef CAS PubMed.
- W. Xu, J. Wang, H. Yu, P. Liu, G.-R. Zhang, H. Huang and D. Mei, Size-dependent electron injection over sensitized semiconductor heterojunctions for enhanced photocatalytic hydrogen production, Appl. Catal., B, 2022, 308, 121218 CrossRef CAS.
- A. García-Baldoví, R. Del Angel, G. Mouchaham, S. Liu, D. Fan, G. Maurin, S. Navalón, C. Serre and H. Garcia, Active site imprinting on Ti oxocluster metal–organic frameworks for photocatalytic hydrogen release from formic acid, Energy Environ. Sci., 2023, 16, 167–177 RSC.
- H. Liu, C. Xu, D. Li and H. L. Jiang, Photocatalytic Hydrogen Production Coupled with Selective Benzylamine Oxidation over MOF Composites, Angew. Chem., Int. Ed., 2018, 57, 5379–5383 CrossRef CAS PubMed.
- X. Wang, L. Zhu, Z. Lv, Z. Qi, Y. Xu, T. Miao, X. Fu and L. Li, Coupled visible-light driven photocatalytic reactions over porphyrin-based MOF materials, Chem. Eng. J., 2022, 442, 136186 CrossRef CAS.
- Y. Zhou, W. Hu, S. Yang, Y. Zhang, J. Nyakuchena, K. Duisenova, S. Lee, D. Fan and J. Huang, Site-Selective Probes of Mixed-Node Metal Organic Frameworks for Photocatalytic Hydrogen Generation, J. Phys. Chem. C, 2019, 124, 1405–1412 CrossRef.
- X. Wang, X. Zhang, W. Zhou, L. Liu, J. Ye and D. Wang, An ultrathin porphyrin-based metal-organic framework for efficient photocatalytic hydrogen evolution under visible light, Nano Energy, 2019, 62, 250–258 CrossRef CAS.
- C. Zhang, C. Xie, Y. Gao, X. Tao, C. Ding, F. Fan and H. L. Jiang, Charge Separation by Creating Band Bending in Metal–Organic Frameworks for Improved Photocatalytic Hydrogen Evolution, Angew. Chem., 2022, 134, e202204108 CrossRef.
- X. Yang, Y. Guo, Y. Lou and J. Chen, Crown-like Zn0.5Cd0.5S/M-TiO2 composites derived from metal-organic frameworks for photocatalytic hydrogen production, Int. J. Hydrogen Energy, 2021, 46, 2156–2165 CrossRef CAS.
- X. Kong, Q. Pan, S. Song, Z. He, T. Zeng and Y. Yu, Dual Metal UiO-Type Metal–Organic Frameworks for Solar-Driven Photocatalytic Hydrogen Evolution, J. Phys. Chem. C, 2021, 125, 20320–20330 CrossRef CAS.
- N. Hu, Y. Cai, L. Li, X. Wang and J. Gao, Amino-Functionalized Titanium Based Metal-Organic Framework for Photocatalytic Hydrogen Production, Molecules, 2022, 27, 4241 CrossRef CAS PubMed.
- Z. Li, J. Zi, X. Luan, Y. Zhong, M. Qu, Y. Wang and Z. Lian, Localized Surface Plasmon Resonance Promotes Metal–Organic Framework–Based Photocatalytic Hydrogen Evolution, Adv. Funct. Mater., 2023, 33, 2303069 CrossRef CAS.
- C. Lu, D. Xiong, C. Chen, J. Wang, Y. Kong, T. Liu, S. Ying and F.-Y. Yi, Indium-Based Metal–Organic Framework for Efficient Photocatalytic Hydrogen Evolution, Inorg. Chem., 2022, 61, 2587–2594 CrossRef CAS PubMed.
- H. Ma, Y. Liu, R. Xiong and J. Wei, Hetero-structured ZnIn2S4-NiO@MOF photo-catalysts for efficient hydrogen evolution, Chin. Chem. Lett., 2022, 33, 1042–1046 CrossRef CAS.
- X. Feng, Y. Pi, Y. Song, C. Brzezinski, Z. Xu, Z. Li and W. Lin, Metal–Organic Frameworks Significantly Enhance Photocatalytic Hydrogen Evolution and CO2 Reduction with Earth-Abundant Copper Photosensitizers, J. Am. Chem. Soc., 2020, 142, 690–695 CrossRef CAS PubMed.
- F. Mu, B. Dai, W. Zhao, L. Zhang, J. Xu and X. Guo, A review on metal-organic frameworks for photoelectrocatalytic applications, Chin. Chem. Lett., 2020, 31, 1773–1781 CrossRef CAS.
- Z. Wang, J. Li, J. Chen, Z. Cao, H. Li, Y. Cao, Q. Li, M. She, P. Liu, S. Zhang and J. Li, A NIR fluorescent probe for imaging thiophenol in the living system and revealing thiophenol-induced oxidative stress, Chin. Chem. Lett., 2023, 34, 108507 CrossRef CAS.
- W. Han, L.-H. Shao, X.-J. Sun, Y.-H. Liu, F.-M. Zhang, Y. Wang, P.-Y. Dong and G.-L. Zhang, Constructing Cu ion sites in MOF/COF heterostructure for noble-metal-free photoredox catalysis, Appl. Catal., B, 2022, 317, 121710 CrossRef CAS.
- T. Wang, X. Tao, Y. Xiao, G. Qiu, Y. Yang and B. Li, Charge separation and molecule activation promoted by Pd/MIL-125-NH2 hybrid structures for selective oxidation reactions, Catal. Sci. Technol., 2020, 10, 138–146 RSC.
- Q.-Q. Li, P.-H. Pan, H. Liu, L. Zhou, S.-Y. Zhao, B. Deng, Y.-J. He, J.-X. Song, P. Liu, Y.-Y. Wang and J.-L. Li, Incorporating a D–A–D-Type Benzothiadiazole Photosensitizer into MOFs for Photocatalytic Oxidation of Phenylsulfides and Benzylamines, Inorg. Chem., 2023, 62, 17182–17190 CrossRef CAS PubMed.
- C. Xu, H. Liu, D. Li, J.-H. Su and H.-L. Jiang, Direct evidence of charge separation in a metal–organic framework: efficient and selective photocatalytic oxidative coupling of amines via charge and energy transfer, Chem. Sci., 2018, 9, 3152–3158 RSC.
- N. S. Bobbitt, M. L. Mendonca, A. J. Howarth, T. Islamoglu, J. T. Hupp, O. K. Farha and R. Q. Snurr, Metal–organic frameworks for the removal of toxic industrial chemicals and chemical warfare agents, Chem. Soc. Rev., 2017, 46, 3357–3385 RSC.
- W.-Q. Zhang, K. Cheng, H. Zhang, Q.-Y. Li, Z. Ma, Z. Wang, J. Sheng, Y. Li, X. Zhao and X.-J. Wang, Highly Efficient and Selective Photooxidation of Sulfur Mustard Simulant by a Triazolobenzothiadiazole-Moiety-Functionalized Metal–Organic Framework in Air, Inorg. Chem., 2018, 57, 4230–4233 CrossRef CAS PubMed.
- Y. Zhu, X. Qiu, S. Zhao, J. Guo, X. Zhang, W. Zhao, Y. Shi and Z. Tang, Structure regulated catalytic performance of gold nanocluster-MOF nanocomposites, Nano Res., 2020, 13, 1928–1932 CrossRef CAS.
- G. Ayoub, M. Arhangelskis, X. Zhang, F. Son, T. Islamoglu, T. Friščić and O. K. Farha, Air oxidation of sulfur mustard gas simulants using a pyrene-based metal–organic framework photocatalyst, Beilstein J. Nanotechnol., 2019, 10, 2422–2427 CrossRef CAS PubMed.
- D. A. Giannakoudakis and T. J. Bandosz, Defectous UiO-66 MOF Nanocomposites as Reactive Media of Superior Protection against Toxic Vapors, ACS Appl. Mater. Interfaces, 2019, 12, 14678–14689 CrossRef PubMed.
- M. Wei, W.-M. Chai, R. Wang, Q. Yang, Z. Deng and Y. Peng, Quinazolinone derivatives: Synthesis and comparison of inhibitory mechanisms on α-glucosidase, Bioorg. Med. Chem., 2017, 25, 1303–1308 CrossRef CAS PubMed.
- S. M. A. H. Siddiki, K. Kon, A. S. Touchy and K.-i. Shimizu, Direct synthesis of quinazolinones by acceptorless dehydrogenative coupling of o-aminobenzamide and alcohols by heterogeneous Pt catalysts, Catal. Sci. Technol., 2014, 4, 1716–1719 RSC.
- S. Parua, S. Das, R. Sikari, S. Sinha and N. D. Paul, One-Pot Cascade Synthesis of Quinazolin-4(3H)-ones via Nickel-Catalyzed Dehydrogenative Coupling of o-Aminobenzamides with Alcohols, J. Org. Chem., 2017, 82, 7165–7175 CrossRef CAS PubMed.
- M. R. Ghaleno, M. Ghaffari-Moghaddam, M. Khajeh, A. R. Oveisi and M. Bohlooli, Iron species supported on a mesoporous zirconium metal-organic framework for visible light driven synthesis of quinazolin-4(3H)-ones through one-pot three-step tandem reaction, J. Colloid Interface Sci., 2019, 535, 214–226 CrossRef CAS PubMed.
- Y. Quan, W. Shi, Y. Song, X. Jiang, C. Wang and W. Lin, Bifunctional Metal–Organic Layer with Organic Dyes and Iron Centers for Synergistic Photoredox Catalysis, J. Am. Chem. Soc., 2021, 143, 3075–3080 CrossRef CAS PubMed.
- I. H. Choi, S. Yoon, S. Huh, S. J. Kim and Y. Kim, Photophysical Properties and Heterogeneous Photoredox Catalytic Activities of Ru(bpy)3@InBTB Metal–Organic Framework (MOF), Chem. – Eur. J., 2020, 26, 14580–14584 CrossRef CAS PubMed.
- H. Han, X. Zheng, C. Qiao, Z. Xia, Q. Yang, L. Di, Y. Xing, G. Xie, C. Zhou, W. Wang and S. Chen, A Stable Zn-MOF for Photocatalytic Csp3−H Oxidation: Vinyl Double Bonds Boosting Electron Transfer and Enhanced Oxygen Activation, ACS Catal., 2022, 12, 10668–10679 CrossRef CAS.
- S. Saini, D. Chakraborty, E. S. Erakulan, R. Thapa, R. Bal, A. Bhaumik and S. L. Jain, Visible Light-Driven Metal–Organic Framework-Mediated Activation and Utilization of CO2 for the Thiocarboxylation of Olefins, ACS Appl. Mater. Interfaces, 2022, 14, 50913–50922 CrossRef CAS PubMed.
- L. Liao, D. Ditz, F. Zeng, M. A. Favaro, A. Iemhoff, K. Gupta, H. Hartmann, C. Szczuka, P. Jakes, P. J. C. Hausoul, J. Artz and R. Palkovits, Efficient Photocatalytic Oxidation of Aromatic Alcohols over Thiophene–based Covalent Triazine Frameworks with A Narrow Band Gap, ChemistrySelect, 2020, 5, 14438–14446 CrossRef CAS.
- H. Li, H. Liu, C. Li, J. Liu, J. Liu and Q. Yang, Micro-scale spatial location engineering of COF–TiO2 heterojunctions for visible light driven photocatalytic alcohol oxidation, J. Mater. Chem. A, 2020, 8, 18745–18754 RSC.
- Q. Zhang, J.-B. Liu, L. Chen, C.-X. Xiao, P. Chen, S. Shen, J.-K. Guo, C.-T. Au and S.-F. Yin, An etching and re-growth method for the synthesis of bismuth ferrite/MIL-53(Fe) nanocomposite as efficient photocatalyst for selective oxidation of aromatic alcohols, Appl. Catal., B, 2020, 264, 118529 CrossRef CAS.
- S. Bhat, M. Aasif, M. Ahmad, F. Khurshaid, G. N. Yatoo, Z.-U. K. Rather, G. A. Bhat, J. Banday and M. Wahid, Ni2P Anchored on Barbituric Acid-Modified Graphitic Carbon Nitride as a Versatile Photocatalyst for Hydroxylation of Aryl Boronic Acids and Oxidation of Benzyl Alcohols, ACS Sustainable Chem. Eng., 2023, 12, 141–153 CrossRef.
- S. Ye, N. Hosono and T. Uemura, Polymer-Grafting from MOF Nanosheets for the Fabrication of Versatile 2D Materials, Adv. Funct. Mater., 2023, 34, 2312265 CrossRef.
- J.-K. Jin, K. Wu, X.-Y. Liu, G.-Q. Huang, Y.-L. Huang, D. Luo, M. Xie, Y. Zhao, W. Lu, X.-P. Zhou, J. He and D. Li, Building a Pyrazole–Benzothiadiazole–Pyrazole Photosensitizer into Metal–Organic Frameworks for Photocatalytic Aerobic Oxidation, J. Am. Chem. Soc., 2021, 143, 21340–21349 CrossRef CAS PubMed.
- M. Hou, L. Lin, X. Chai, X. Zhao, B. Qiao and Z. Jiang, Enantioselective photoredox dehalogenative protonation, Chem. Sci., 2019, 10, 6629–6634 RSC.
- H. Zheng, Y. Hou, S. Li, J. Ma, J. Nan and T. Li, Recent advances in the application of metal organic frameworks using in advanced oxidation progresses for pollutants degradation, Chin. Chem. Lett., 2022, 33, 5013–5022 CrossRef CAS.
- J. He, J. Li, Q. Han, C. Si, G. Niu, M. Li, J. Wang and J. Niu, Photoactive Metal–Organic Framework for the Reduction of Aryl Halides by the Synergistic Effect of Consecutive Photoinduced Electron-Transfer and Hydrogen-Atom-Transfer Processes, ACS Appl. Mater. Interfaces, 2019, 12, 2199–2206 CrossRef PubMed.
- C. Chen, Y. Miao, K. De Winter, H.-J. Wang, P. Demeyere, Y. Yuan and F. Verpoort, Ruthenium-Based Catalytic Systems Incorporating a Labile Cyclooctadiene Ligand with N-Heterocyclic Carbene Precursors for the Atom-Economic Alcohol Amidation Using Amines, Molecules, 2018, 23, 2413 CrossRef PubMed.
- H. Jiang, H. Cheng, C. Zang, J. Tan, B. Sun and F. Bian, Photocatalytic aldehydes/alcohols/toluenes oxidative amidation over bifunctional Pd/MOFs: Effect of Fe-O clusters and Lewis acid sites, J. Catal., 2021, 401, 279–287 CrossRef CAS.
- B. Ma, Q. Xia, D. Wang, J. K. Jin, Z. Li, Q. J. Liang, M. Y. Sun, D. Liu, L. J. Liu, H. X. Shu, J. Yang, D. Li and J. He, Metal–Organic Framework Supported Copper Photoredox Catalysts for Iminyl Radical–Mediated Reactions, Angew. Chem., Int. Ed., 2023, 62, e202300233 CrossRef CAS PubMed.
- H. Liu, Q.-Q. Li, L. Zhou, B. Deng, P.-H. Pan, S.-Y. Zhao, P. Liu, Y.-Y. Wang and J.-L. Li, Confinement of Organic Dyes in UiO-66-Type Metal–Organic Frameworks for the Enhanced Synthesis of [1,2,5]Thiadiazole[3,4 g]benzoimidazoles, J. Am. Chem. Soc., 2023, 145, 17588–17596 CrossRef CAS PubMed.
- H. Liu, S.-Y. Zhao, Q.-Q. Li, X.-S. Li, Y.-J. He, P. Liu, Y.-Y. Wang and J.-L. Li, Linker engineering in UiO-68-type metal–organic frameworks for the photocatalytic thioamide cyclization, J. Mater. Chem. A, 2024, 12, 9527–9531 RSC.
- H. Liu, Q. Li, P. Pan, L. Zhou, B. Deng, S. Zhao, P. Liu, Y. Wang and J. Li, Robust chromophore-integrated MOFs as highly visible-white-light active and tunable size-selective photocatalysts towards benzothiazoles, Chin. Chem. Lett., 2023, 34, 108562 CrossRef CAS.
- M. Ding, X. Cai and H.-L. Jiang, Improving MOF stability: approaches and applications, Chem. Sci., 2019, 10, 10209–10230 RSC.
- G. Cai, P. Yan, L. Zhang, H.-C. Zhou and H.-L. Jiang, Metal–Organic Framework-Based Hierarchically Porous Materials: Synthesis and Applications, Chem. Rev., 2021, 121, 12278–12326 CrossRef CAS PubMed.
- C. Zhang, D. Lei, C. Xie, X. Hang, C. He and H. L. Jiang, Piezo-Photocatalysis over Metal-Organic Frameworks: Promoting Photocatalytic Activity by Piezoelectric Effect, Adv. Mater., 2021, 33, 2106308 CrossRef CAS PubMed.
Footnote |
† These authors contributed equally to this work. |
|
This journal is © the Partner Organisations 2024 |
Click here to see how this site uses Cookies. View our privacy policy here.