DOI:
10.1039/D3QM01077E
(Research Article)
Mater. Chem. Front., 2024,
8, 2173-2180
Simultaneously excited downshifting/upconversion luminescence from lanthanide-doped core–shell lead-free perovskite nanocrystals for encryption and data storage†
Received
5th October 2023
, Accepted 2nd February 2024
First published on 2nd February 2024
Abstract
Metal ion doping is the most viable method to modulate the optical properties of lead-free Cs2AgInCl6 double perovskite (DP) nanocrystals (NCs). However, despite the fact that doping multiple cations can allow for emission color tuning, their random distribution into the perovskite matrix poses severe limitations caused by uncontrollable and adverse energy interactions between the dopants, resulting in poor emission color purity and photoluminescence (PL) quenching. To address this issue, we designed Cs2AgInCl6-based core–shell NCs doped with different metal ions in the two layers via an epitaxial growth strategy, with the aim to spatially separate the diverse optically active centers. We selected Cs2AgInCl6:Bi as the core and Cs2AgInCl6:Yb, Er as the shell and successfully constructed Cs2AgInCl6:Bi@Cs2AgInCl6:Yb, Er core–shell NCs. The so-designed core–shell NCs allow for non-interacting dual-modal luminescence (upconversion/downshifting) yielding not only broadband self-trapping exciton orange emission under ultraviolet (370 nm) excitation but also upconversion green light emission under near-infrared (980 nm) excitation, so far unprecedented in DP NCs. Taking advantage of the high photostability and stability against moisture bestowed by the shell coating, we demonstrate that the resulting core–shell DP NCs can be successfully employed as luminescent ink for dual-mode anti-counterfeiting labels. These results not only provide a novel design strategy for multi-emissive lead-free NCs, but can also represent a milestone to realize controllable luminescent materials for anti-counterfeiting and information storage.
Introduction
Double perovskite (DP) Cs2AgInCl6 nanocrystals (NCs) are attracting much interest as a replacement for the classic and toxic lead halide CsPbX3 perovskites for optical and optoelectronic applications, because of their superior environmental stability, widely tunable direct bandgap and achievable broad-band self-trapping exciton (STE) emission. However, their low photoluminescence (PL) intensity, single emission band and lack of near-infrared (NIR) photoexcitation limit their further development.1–6 It has been shown that the incorporation of metal ions, including d- and p-metal and 4f-lanthanide ions (Ln3+), is an effective strategy to expand the luminescent properties of DPs in the visible and near-infrared (NIR) spectral regions.7–12 However, current research mainly focuses on the traditional single fluorescence mode, only featuring downshifted (UV-Vis to Vis/NIR) monotonous emission with non-tunable optical characteristics, whereas the realization of upconversion (NIR to Vis) emission still remains a challenge.13 These inherent limitations greatly hinder the applications of DP materials in information security. Therefore, it is highly desirable to endow Cs2AgInCl6 NCs with various and controllable optical modes by bringing together both downshifting and upconversion luminescence mechanisms simultaneously,14–16 to realize photonic devices for data encryption and storage.
Among the metal ion dopants yielding downshifted broadband luminescence, Bi3+ is particularly popular because of its excellent stability and environmental friendliness.17–19 The incorporation of Bi3+ with its outermost ns2 electrons into Cs2AgInCl6 DP allows for tailoring the projected boundary state, leading to significant changes in the optical absorption and emission properties and realizing STE downshifted visible luminescence under UV excitation.20,21 On the other hand, 4f-metal ions, whose emission, originating from intra-shell f–f transitions, is characterized by high color purity and long-lifetimes, can in principle allow for narrow-band upconversion luminescence in the visible upon NIR excitation.22 Among the Ln3+ ions, Yb3+, which can be photoexcited at 980 nm, is often coupled as a sensitizer with the Er3+ activator to yield high color purity green upconversion emission.23–25 Chen's group incorporated Yb3+ and Er3+ into Cs2AgBiCl6 DP attempting to achieve upconversion and downshifting dual-mode PL; however, the coupling of the energy levels of Bi3+ and Er3+ led to a yellow-green light emission with poor color purity.26,27 Therefore, although Ln3+ and transition metal ion doping can endow Cs2AgInCl6 NCs with excellent optical performance, the limited color purity caused by the energy level coupling and uncontrollable energy transfer pathways between the dopants still remains to be solved, but this aspect is still lacking researchers’ attention.
To overcome the issue mentioned above, it is necessary to spatially separate the downshifting and upconversion emission centers. Efforts from this field mainly focused on the mechanical mixing of upconversion fluoride nanoparticles and perovskite NCs. Zhang's group designed a nanocomposite composed of CsPbX3 perovskite NCs decorated with upconversion Yb3+/Er3+ doped NaYF4 fluoride nanoparticles to achieve downshifting/upconversion dual-mode emission, but the nanocomposite needs to be mixed with prepolystyrene to improve its stability to light and water, due to the absence of protection derived from the heterogeneous structure.28 The most striking limitation of this approach comes from the fact that the establishment of heterogeneous core–shell structures to assemble fluoride and perovskite hosts is nearly inaccessible due to the lattice mismatch of the components.29–31
In this work, we successfully designed DP only core–shell nanostructures based on Cs2AgInCl6, with the aim to confine the upconversion and downshifting emissive centers achieving excellent multimodal optical properties and good environmental tolerance while avoiding the structural instability induced by lattice mismatch. We selected Cs2AgInCl6
:
2%Bi NCs as core, and Cs2AgInCl6
:
20%Yb, 7%Er NCs as shell to construct Cs2AgInCl6
:
2%Bi@Cs2AgInCl6
:
20%Yb, 7%Er core–shell DP NCs by an epitaxial growth method. Under 370 nm excitation, the core–shell NCs display bright orange light stemming from the STEs emission of the Bi3+ doped core, while 980 nm photoexcitation triggers green light upconverted emission with high color purity. The successful establishment of core–shell structured DP NCs not only realizes multi-mode PL in the visible region with high color purity under UV/NIR excitation, but also further improves the stability of the DP NCs. Thanks to the combination of these properties, the designed core–shell DP NCs are suitable for the practical application in the field of dual-modal luminescence anti-counterfeiting, which we demonstrate through the successful realization of a prototype information storage and encryption system working under UV and NIR light illumination.
Experimental
Materials and chemicals
Silver acetate (Ag(ac), 99.95%), indium(III) acetate (In(ac)3, 99.99%), bismuth(III) acetate (Bi(ac)3, 99.99%), ytterbium(III) acetate (Yb(ac)3, 99.9%), erbium(III) acetate (Er(ac)3, 99.99%), cesium carbonate (Cs2CO3, 99.99%), oleic acid (OA, 90%), oleylamine (Olam, 90%), cyclohexane (99%), benzoyl chloride (Bz-Cl, 99%), and diphenyl ether (DPE, 99.9%) were all purchased from Aladdin. All chemicals were directly used without any further purification.
Synthesis of Cs-oleate
Cs2CO3 (1630 mg) and OA (20 mL) were loaded into a 50 mL three-necked flask, then heated to 100 °C at a rate of 10 °C min−1 and degassed under vacuum for 40 min. The mixture was then heated to 150 °C in an N2 atmosphere and reacted for 3 h until the solution became clear. Finally, the obtained solution was cooled to room temperature and stored in a light-protected glass vial.
Synthesis of undoped and Bi3+ doped Cs2AgInCl6 NCs (denoted as x%Bi)
In a typical synthesis, In(ac)3 (0.25 mmol), Ag(ac) (0.2 mmol), Olam (0.5 mL), Cs-oleate solution (1 mL), and DPE (4 mL) were added into a 50 ml three-necked flask and degassed under vacuum for 30 min. The mixed solution was heated up to 120 °C at a ramping rate of 10 °C min−1 under N2 atmosphere, then 0.5 mL of degassed DPE thoroughly mixed with 0.25 mL of Bz-Cl was swiftly injected into the three-necked flask. The flask was immersed in an ice-water bath for 15 s to quench the reaction. The obtained crude solution was centrifuged at 10
000 rpm for 5 min. The supernatant was discarded, and the residues were redispersed in 3 mL of cyclohexane and washed with ethyl acetate. The washing step was repeated at least twice. x%Bi NCs (the molar concentration of the prepared samples is 0.02 mol L−1) were synthesized through a similar procedure as mentioned above, except adding the appropriate quantities of Bi(ac)3 to the initial reaction mixture (x = Bi/In precursors percentage of 0.5, 1, 2, 5, and 10).
Synthesis of Yb3+, Er3+ co-doped Cs2AgInCl6 NCs (denoted as 20%Yb, x%Er)
20%Yb, x%Er NCs (the molar concentration of the prepared samples is 0.02 mol L−1) were synthesized following a similar protocol, except employing 0.05 mmol of Yb(ac)3 and adding the appropriate amounts of Er(ac)3 to the initial reaction mixture (x = Er/In precursors percentage of 1, 3, 5, 7, and 10).
Synthesis of Bi3+, Yb3+, Er3+ co-doped Cs2AgInCl6 NCs (denoted as 2%Bi, 20%Yb, 7%Er)
2%Bi, 20%Yb, 7%Er NCs (the molar concentration of the prepared samples is 0.02 mol L−1) were synthesized following a similar protocol, except employing 0.1775 mmol of In(ac)3 in the initial reaction mixture.
Synthesis of Cs2AgInCl6
:
2%Bi core NCs (denoted as 2%Bi)
2%Bi NCs were synthesized by adopting the same protocol as mentioned in the synthesis of Bi3+ doped Cs2AgInCl6 NCs, and the as-prepared NCs were dispersed in 1 mL of cyclohexane for storage.
Synthesis of Cs2AgInCl6
:
2%Bi@Cs2AgInCl6
:
20%Yb, 7%Er core–shell NCs (denoted as 2%Bi@20%Yb, 7%Er)
Typically, In(ac)3 (0.1825 mmol), Ag(ac) (0.2 mmol), Yb(ac)3 (0.05 mmol), Er(ac)3 (0.0175 mmol), Olam (0.5 mL), Cs-oleate solution (1 mL), and DPE (4 mL) were loaded into a 50 mL three-necked flask, and degassed under vacuum for 30 min. Then, the temperature was increased to 60 °C at a heating rate of 5 °C min−1 under a N2 atmosphere. Meanwhile, 2%Bi NCs (1 mL) were rapidly injected into the flask and degassed under vacuum for 30 min to remove the cyclohexane. The temperature was then increased to 120 °C at a heating rate of 10 °C min−1 under a N2 atmosphere, following which 0.5 mL of degassed DPE thoroughly mixed with 0.25 mL of Bz-Cl was swiftly injected into the three-necked flask. The flask was immersed in an ice-water bath for 15 s to quench the reaction. The resulting crude solution was centrifuged at 10
000 rpm for 5 min, and the supernatant was discarded. The residues were redispersed in 3 mL of cyclohexane and washed with ethyl acetate. The washing step was repeated at least twice (the molar concentration of the prepared samples is 0.02 mol L−1).
Characterization
The X-ray diffraction (XRD, XRD-7000) patterns were collected using a Shimadzu X-ray diffractometer with Cu Kα radiation (λ = 1.54 Å). Transmission electron microscopy (TEM) images, high-resolution TEM (HRTEM) images, energy-dispersive X-ray spectroscopy (EDS) spectra and selected area electron diffraction patterns (SAED) of the samples were obtained using a JEM-F200 electron microscope. The UV-Vis absorption spectra were measured using a Hitachi UH4150 in the range of 200–800 nm. The PL spectra and decay curves of samples were collected using an Edinburgh fluorescence spectrometer FLS1000. Inductively coupled plasma-optical emission spectrometry (ICP-OES) data were recorded using an Agilent 5110 optical emission spectrometer. The X-ray photoelectron spectroscopy (XPS) data were taken by a Thermo Scientific K-Alpha using a monochromatic Al Kα source. The thermogravimetric (TG) measurements were performed using a PerkinElmer STA-8000 in the temperature range from 30 °C to 800 °C, at a ramping rate of 10 °C min−1 under Ar flow (20 mL min−1).
Results and discussion
The pristine Cs2AgInCl6 and its doped counterparts (2%Bi, 20%Yb, 7%Er), as well as the core–shell structured 2%Bi@20%Yb, 7%Er, have been successfully synthesized via a modified thermal injection method.19 Their crystal structures have been characterized by XRD. As seen in Fig. 1a, all the samples exhibit high crystallinity, and the XRD diffraction peaks can be classified as a cubic DP (ICSD No. 257115). The consilience of XRD patterns indicates that there is no detectable impurity introduced into the DP with the employed Ln3+ doping method. The diffraction peak of the (220) plane of Ln3+-doped samples around 2θ = 24° is slightly shifted towards the smaller-angle region compared to that of the pristine Cs2AgInCl6 (Fig. 1a), which may be attributed to the replacement of In3+ with Bi3+, Yb3+ and Er3+.10,32 The lattice parameters and unit cell volume of the DP NCs, summarized in Table S1 (ESI†), gradually increase as the dopants are introduced. Fig. 1b represents a schematic crystal structure of Bi3+-doped and Yb3+, Er3+ co-doped Cs2AgInCl6 unit cells, which are constituted by Cs+ ions, [AgCl6]5−, [InCl6]3−, [BiCl6]3−, [YbCl6]3− and [ErCl6]3− octahedra, depending on the crystal composition. As illustrated, some of the In3+ sites are occupied by the dopants.18,25,26,33
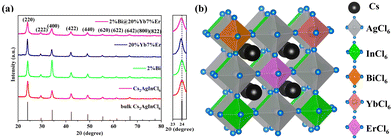 |
| Fig. 1 (a) XRD patterns of Cs2AgInCl6, Cs2AgInCl6:2%Bi (denoted as 2%Bi), Cs2AgInCl6:20%Yb, 7%Er (denoted as 20%Yb, 7%Er) and Cs2AgInCl6:2%Bi@Cs2AgInCl6:20%Yb, 7%Er (denoted as 2%Bi@20%Yb, 7%Er) NCs (left) and the zoomed-in XRD patterns in the range of 23–24.5° (right); (b) schematic structure illustrating the unit cells of Bi3+-doped Cs2AgInCl6 and Yb3+, Er3+ co-doped Cs2AgInCl6 for brevity. | |
XPS data in Fig. S1a–c (ESI†) show that all the samples contain Cl, Cs, Ag, and In elements, whereas high-resolution spectra prove the presence of Bi, Yb, Er in the core–shell NCs, as seen in Fig. S1d–f (ESI†). In addition, Fig. S1b and c (ESI†) indicate that the characteristic peaks of In 3d3/2 and In 3d5/2 in Ln3+-doped NCs are slightly shifted towards the higher binding energy region compared to the pristine sample, while there is no obvious change in the Ag 3d peak. This may be ascribed to the lattice expansion resulting from the successful incorporation of Ln3+, as Yb3+ and Er3+ occupy the In3+ sites rather than the Ag+ sites.10 The presence of Bi, Yb, and Er elements in the as-prepared NCs is also confirmed by ICP-OES measurements, and the atomic ratios of Cs/In, Bi/In, Yb/In, and Er/In in different samples are shown in Table S2 (ESI†).
The detailed morphologies of the as-prepared NCs have been investigated by TEM. The synthesized NCs with different doping contents all exhibit similarly uniform cubic morphologies and high crystallinity (Fig. 2). The average sizes of the NCs are altered on variations of the composition but maintain a homogeneous distribution (Fig. S2, ESI†). In addition, HRTEM images in the insets of Fig. 2(a, c, e and g) show that the lattice spacing of the (220) crystal plane is increased from 0.37 nm to 0.38 nm, 0.40 nm and 0.39 nm depending on the doped cations, which is consistent with the lattice expansion validated by the XRD analysis. The SAED patterns, shown in Fig. 2(b, d, f and h), illustrate the presence of (220) and (400) planes of the cubic structure, revealing the consistent DP structure of as-prepared nanocrystals. The TEM-EDS results in Fig. S3 (ESI†) indicate that the nanocubes are well-constructed with evenly distributed elements, whose content is consistent with the ICP data. The particle size of the core-only 2%Bi NCs is 11.9 nm, while the size of 2%Bi@20%Yb, 7%Er NCs is 15.2 nm. The EDS mapping of 2%Bi@20%Yb, 7%Er NCs is shown in Fig. S3 (ESI†). We investigated the XRD pattern and TEM images of a physical mixture of 2%Bi and 20%Yb, 7%Er NCs (Fig. S4, ESI†) to reveal the microstructural difference in contrast. As seen in the XRD pattern, the physical mixture exhibits the cubic DP structure (ICSD No. 257115) without detectable impurities. Compared to the well-defined core–shell structured DP NCs (Fig. 2g), the NCs with different dopants are randomly distributed in the physical mixture, leading to strong irregularity in morphologies and sizes (Fig. S4b, ESI†). Thus, based on the combination of the TEM, XRD and XPS investigation, it is possible to conclude that the seeded growth method adopted in this work was successful for the synthesis of the designed core–shell DP NCs.
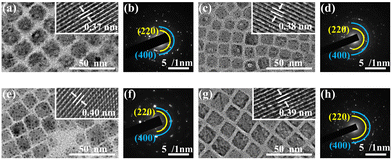 |
| Fig. 2 TEM and HRTEM images (insets) of (a) Cs2AgInCl6, (c) 2%Bi, (e) 20%Yb, 7%Er and (g) 2%Bi@20%Yb, 7%Er NCs, and their corresponding SAED patterns (b), (d), (f) and (h). Some ‘dark dots’ appearing in the TEM images over the surface of the NCs are attributable to the reduction of Ag+ to Ag0 by Olam.21,34 | |
As shown in Fig. 3, the optical features of both the Bi3+-doped and the Yb3+, Er3+ co-doped DP NCs are fully preserved in the constructed core–shell NCs (Fig. S7 and S8, ESI†). The core–shell DP NCs present excitation-dependent multicolor emission properties, yielding a broadband STE orange emission peaked at around 690 nm under 370 nm excitation (Fig. 3a) and narrow emission peaks at 524 nm, 550 nm and 659 nm, corresponding to green light (Fig. 3b) when illuminated with 980 nm light. The possible PL mechanisms are shown in Fig. 3c and d (detailed in the ESI†). The CIE coordinates of the core–shell Cs2AgInCl6 NCs at different excitation wavelengths (Fig. S10a, ESI†) show that the core–shell NCs display strikingly different orange and green colors when excited with UV and NIR light, respectively.
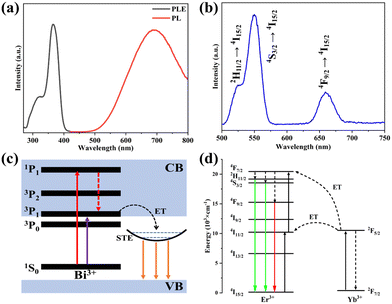 |
| Fig. 3 PL properties of 2%Bi@20%Yb, 7%Er. (a) PL (λex = 370 nm) and PLE (λem = 690 nm) spectra; (b) PL (λex = 980 nm) spectrum; (c) schematic diagram of the energy-transfer mechanism of Bi3+ in core–shell NCs; (d) schematic diagram of the energy-transfer mechanism of Yb3+–Er3+ in core–shell NCs. | |
In fact, the spatial separation and confinement of the downshifting and upconversion emitting centers in such layered nanostructures allows for successfully ruling out adverse energy interactions and uncontrollable energy transfer pathways, especially between Bi3+ and Er3+, leading to poor color purity PL and PL quenching. To confirm this conclusion, we compared the PL properties of 2%Bi@20%Yb, 7%Er, 2%Bi and 2%Bi, 20%Yb, 7%Er NCs. As expected, the emission intensity upon 370 nm excitation of core–shell NCs is significantly stronger than that of 2%Bi and 2%Bi, 20%Yb, 7%Er NCs (Fig. 4a and b), whereas the PL quantum yield (PLQY) of core–shell NCs increases to 7.4% compared to the 2.2% value retrieved for 2%Bi, 20%Yb, 7%Er NCs. This indicates that the spatial separation of the diverse optically active centers in the constructed core–shell structure ultimately improves the PL performance of DP NCs. To further prove this conclusion, we performed time-resolved PL measurements of 2%Bi@20%Yb, 7%Er NCs and 2%Bi, 20%Yb, 7%Er NCs at 690 nm (Fig. 4c and d). It is observed that the retrieved lifetime (τavg) of 2%Bi, 2%Yb, 7%Er NCs is reduced to 163.21 ns compared with 2%Bi NCs (τavg = 195.64 ns) likely due to a competitive and uncontrolled energy transfer between Bi3+ and Er3+ (Fig. S7d, ESI†), which quenches the emission. On the other hand, the average lifetime (τavg) of core–shell NCs remarkably increases up to 298.31 ns, leading to the observed increased PLQY and emission intensity.
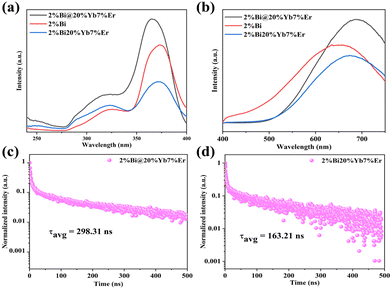 |
| Fig. 4 (a) and (b) The PLE and PL spectra of 2%Bi@20%Yb, 7%Er, 2%Bi and 2%Bi, 20%Yb, 7%Er NCs; (c) and (d) PL decay curves of 2%Bi@20%Yb, 7%Er and 2%Bi, 20%Yb, 7%Er NCs emission at around 690 nm under 375 nm excitation. | |
Additionally, the core–shell structured NCs are beneficial in broadening the absorption and excitation ranges (Fig. 4a and Fig. S5b, ESI†). Meanwhile, we can observe from the PLE spectra (Fig. 4a) the two broad excitation peaks of the three samples are centered at approximately 320 nm and 370 nm. The former was attributed to the sub-band gap shallow trap state, while the latter was attributed to the spin-allowed 1S0 → 3P1 transition of Bi3+.20 Interestingly, the PLE spectrum of 2%Bi@20%Yb, 7%Er NCs showed a blue shift compared to the others’, which could be attributed to the reduced surface defects by the construction of the core–shell structure.28,31,35,36
In addition, the construction of core–shell NCs is an effective strategy to protect the active core (2%Bi), improving the structural and optical stability of DP NCs. Compared with the physical mixture of bare 2%Bi and 20%Yb, 7%Er NCs as well as the naked 2%Bi NCs, the constructed core–shell NCs exhibit an overall improvement in PL stability under various conditions, e.g. moisture, continuous UV light irradiation and high temperatures (Fig. 5). The molar concentration of the prepared samples is all 0.02 mol L−1. The luminescence intensity was recorded as a function of time in a moisture environment (0.1 mL of DI water was added to 0.9 mL of 2%Bi@20%Yb, 7%Er core–shell NCs, the mixture of 2%Bi NCs and 20%Yb, 7%Er NCs and 2%Bi NCs cyclohexane solutions, respectively) or under UV light irradiation. The luminescence intensity was measured at different temperatures from 20 to 80 °C. As the boiling point of cyclohexane is around 80.7 °C, we set the maximum tested temperature to 80 °C. First, The core–shell structured NCs show superior optical stability in moisture, as their normalized PL intensity remains above 0.2 for 90 minutes, while the naked particles are nearly quenched (Fig. 5a). Similarly, under long-time UV irradiation (Fig. 5b), the core–shell NCs present much slower decay than the other NCs in the entire period of investigation (300 minutes). Finally, upon temperature increase (20–80 °C), the PL intensity of bare 2%Bi NCs rapidly declines below 0.3 at 50 °C, and further decreases to below 0.1 above this point, while the PL intensity of core–shell NCs remains above that of the nanocrystal mixture in the range from 30 °C to 80 °C, with a much slower decrease trend (Fig. 5c). These results indicate that effective protection of Bi3+-doped Cs2AgInCl6 DP NCs from external perturbing agents is realized by the designed core–shell structure.
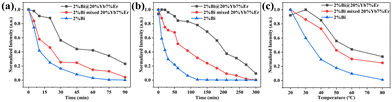 |
| Fig. 5 Comparison of the PL intensity of 2%Bi@20%Yb, 7%Er core–shell NCs, the mixture of 2%Bi NCs and 20%Yb, 7%Er NCs and 2%Bi NCs under 370 nm excitation: (a) in cyclohexane and DI water (v/v = 1 : 9) mixed solvents under 370 nm excitation; (b) under continuous UV light irradiation; (c) at different temperatures. | |
To demonstrate the feasibility and applicability of core–shell DP NCs in information storage and anti-counterfeiting, we designed a series of patterns taking advantage of the dual-mode PL (upconversion and downshifting) properties, as shown in Fig. 6. 2%Bi(I), 20%Yb, 7%Er(II) and 2%Bi@20%Yb, 7%Er(III) NCs were dispersed in cyclohexane to obtain a fluorescent ink, then deposited on 5 × 5 mm silicon wafers to display the elementary information (Fig. 6a). As shown, there is no color change observed on all three types of materials under daylight irradiation. Under 365 nm excitation, material I and material III emitted orange light, and material II emitted whitish light stemming from the Cs2AgInCl6 matrix (Fig. S9, ESI†). Under 980 nm excitation, only materials II and III emitted green light. Each silicon wafer coated with PL material acts as a basic module displaying optical signals. The designed pattern is constituted by these pixel-like modules that exhibit preloaded information under a specific excitation mode. As shown in Fig. 6b, the optical signal modules of materials I and III were arranged into a 5 × 11 array in a specific order. When illuminated at 365 nm excitation wavelength, all the modules displayed an orange color. Under 980 nm light irradiation, the modules coated with material I showed no response, while the other modules emitted green light owing to the presence of core–shell DP (material III). As a consequence, the whole array exhibits the preloaded information (“S”, “W”, “U”) in contrast, which proves the feasibility of the as-prepared materials for information storage.
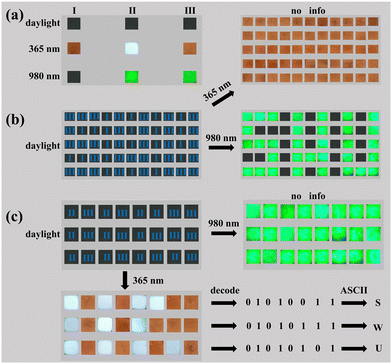 |
| Fig. 6 Storage and decoding of optical information. (a) PL photographs of different NCs deposited on silicon wafers (5 × 5 mm) under the illumination of daylight, 365 nm and 980 nm light, respectively (I: 2%Bi NCs, II: 20%Yb, 7% Er NCs, III: 2%Bi@20%Yb, 7%Er NCs); (b) under the 365 nm and 980 nm excitation, the PL signals of materials I and III display the preloaded information “S”, “W”, “U”; (c) under 365 nm and 980 nm excitation, the PL signals of materials II and III were converted into ASCII codes and read the preload information “S”, “W”, “U”. | |
Besides the storage/display of directly readable characters, such luminescent materials can also be utilized to store the information encoded with 8-bit ASCII code for future integration with electronic systems, as shown in Fig. 6c. When they were exposed to 365 nm light, the modules containing material II and III emitted white and orange light, respectively. Thus, according to the sequence of emission colors, the information embedded in the three module arrays can be decoded as “S”, “W”, “U”. When excited at 980 nm, all modules only emitted visible green light, protecting the stored information. The above results further prove that the investigated core–shell NCs possess a great application prospect in the fields of anti-counterfeiting and information storage, and the dual-mode emission characteristics of preloaded information can also play a vital role in improving the level of information security.
Conclusions
In summary, novel luminescent lead-free Cs2AgInCl6-based core–shell NCs have been resoundingly synthesized via an epitaxial growth method, where a Cs2AgInCl6:Bi core is surrounded by a Cs2AgInCl6:Er, Yb shell. The resulting core–shell perovskite NCs exhibit a broadband downshifted STE orange emission under UV excitation related to the incorporation of Bi3+ centers; as well as the first time reported green upconversion emission under 980 nm excitation from the Yb3+, Er3+ combination in the shell. Thanks to the spatial separation and confinement of the luminescent downshifting and upconversion dopant centers, adverse intermetallic interactions and uncontrollable energy transfer pathways are ruled out, resulting in high emission color purity in the visible region under dual-mode excitation. The constructed Cs2AgInCl6:Bi@Cs2AgInCl6:Yb, Er core–shell NCs not only allow for controllable wavelength-responsive multi-color emission but also further improve the stability against moisture, light and high temperature exposure of Cs2AgInCl6 DP NCs. The satisfactory optical and structural features of the obtained luminophores enable promising applications in the fields of anti-counterfeiting and information storage.
Author contributions
Experiments and original draft – writing were completed by Endian Cui under the guidance of Jing Liu, Kai Liu and Flavia Artizzu. Gaoyuan Xing, Xiangyang Yuan, Yi Zhang, Xiaoling Liao, Jianfeng Tang, Yanan Zhao, and Pengzhou Zhao contributed to the data curation and experimental discussion. Funding acquisition was done by Jing Liu.
Conflicts of interest
There are no conflicts to declare.
Acknowledgements
This research was supported by the China Postdoctoral Science Foundation (Grant No. 2022M711514, No. 2022T150298) and the starting grant for talents of Chongqing Jiaotong University. We acknowledge the critical and quantity of testing work supported by the Shiyanjia Lab (https://www.shiyanjia.com). We thank the Analytical and Testing Center of Southwest University for their assistance with UV-Vis absorption spectra analysis.
Notes and references
- G. Volonakis, A. A. Haghighirad, R. L. Milot, W. H. Sio, M. R. Filip, B. Wenger, M. B. Johnston, L. M. Herz, H. J. Snaith and F. Giustino, Cs2AgInCl6: A new lead-free halide double perovskite with direct band gap, J. Phys. Chem. Lett., 2017, 8, 772–778 CrossRef CAS PubMed.
- J. Zhou, Z. Xia, M. S. Molokeev, X. Zhang, D. Peng and Q. Liu, Composition design, optical gap and stability investigations of lead-free halide double perovskite Cs2AgInCl6, J. Mater. Chem. A, 2017, 5, 15031–15037 RSC.
- J. Luo, S. Li, H. Wu, Y. Zhou, Y. Li, J. Liu, J. Li, K. Li, F. Yi, G. Niu and J. Tang, Cs2AgInCl6 double perovskite single crystals: parity forbidden transitions and their application for sensitive and fast UV photodetectors, ACS Photonics, 2018, 5, 398–405 CrossRef CAS.
- S. Li, J. Luo, J. Liu and J. Tang, Self-trapped excitons in all-inorganic halide perovskites: Fundamentals, status, and potential applications, J. Phys. Chem. Lett., 2019, 10, 1999–2007 CrossRef CAS PubMed.
- J. C. Dahl, W. T. Osowiecki, Y. Cai, J. K. Swabeck, Y. Bekenstein, M. Asta, E. M. Chan and A. P. Alivisatos, Probing the stability and band gaps of Cs2AgInCl6 and Cs2AgSbCl6 lead-free double perovskite nanocrystals, Chem. Mater., 2019, 31, 3134–3143 CrossRef CAS.
- J. Luo, M. Hu, G. Niu and J. Tang, Lead-free halide perovskites and perovskite variants as phosphors toward light-emitting applications, ACS Appl. Mater. Interfaces, 2019, 11, 31575–31584 CrossRef CAS PubMed.
- R. Song, S. Xu, Y. Li, Q. Zhang, Y. Gao, H. Yu, Y. Cao, X. Li, S. Zhang and B. Chen, Bi3+ and Tb3+ co-doped Cs2AgInCl6 lead-free double perovskite nanocrystals for detection of temperature and copper ions, Spectrochim. Acta, Part A, 2023, 288, 122181 CrossRef CAS PubMed.
- L. Chen, H. Jiang, Z. Luo, G. Liu, X. Wu, Y. Liu, P. Sun and J. Jiang, Enhancement of the efficiency and thermal stability of the double perovskite Cs2AgInCl6 single crystal by Sc substitution, Mater. Adv., 2022, 3, 4381–4386 RSC.
- S. Wang, R. Shi, B. Tang, Y. Xiong, A. Portniagin, X. Zhao, S. V. Kershaw, R. Long and A. L. Rogach, Co-doping of tellurium with bismuth enhances stability and photoluminescence quantum yield of Cs2AgInCl6 double perovskite nanocrystals, Nanoscale, 2022, 14, 15691–15700 RSC.
- Y. Liu, X. Rong, M. Li, M. S. Molokeev, J. Zhao and Z. Xia, Incorporating rare-earth terbium(III) Ions into Cs2AgInCl6:Bi nanocrystals toward tunable photoluminescence, Angew. Chem., Int. Ed., 2020, 59, 11634–11640 CrossRef CAS PubMed.
- Y. Mahor, W. J. Mir and A. Nag, Synthesis and near-infrared emission of Yb-Doped Cs2AgInCl6 double perovskite microcrystals and nanocrystals, J. Phys. Chem. C, 2019, 123, 15787–15793 CrossRef CAS.
- W. Lee, S. Hong and S. Kim, Colloidal synthesis of lead-Free silver–indium double-perovskite Cs2AgInCl6 nanocrystals and their doping with lanthanide Ions, J. Phys. Chem., 2019, 123, 2665–2672 CrossRef CAS.
- H. Arfin, J. Kaur, T. Sheikh, S. Chakraborty and A. Nag, Bi3+-Er3+ and Bi3+-Yb3+ co-doped Cs2AgInCl6 double perovskite near-infrared emitters, Angew. Chem., Int. Ed., 2020, 59, 11307–11311 CrossRef CAS PubMed.
- J. Liu, H. Rijckaert, M. Zeng, K. Haustraete, B. Laforce, L. Vincze, I. Van Driessche, A. M. Kaczmarek and R. Van Deun, Simultaneously excited downshifting/upconversion luminescence from lanthanide-doped core/shell fluoride nanoparticles for multimode anticounterfeiting, Adv. Funct. Mater., 2018, 28, 1707365 CrossRef.
- D. Gao, D. Zhao, Y. Pan, R. Chai, Q. Pang, X. Zhang and W. Chen, Extending the color response range of Yb3+ concentration-dependent multimodal luminescence in Yb/Er doped fluoride microrods by annealing treatment, Ceram. Int., 2021, 47, 32000–32007 CrossRef CAS.
- D. Gao, J. Gao, F. Gao, Q. Kuang, Y. Pan, Y. Chen and Z. Pan, Quintuple-mode dynamic anti-counterfeiting using multi-mode persistent phosphors, J. Mater. Chem. C, 2021, 9, 16634–16644 RSC.
- S. Wang, J. Qi, S. V. Kershaw and A. L. Rogach, Co-doping of cerium and bismuth into lead-free double perovskite Cs2AgInCl6 nanocrystals results in improved photoluminescence efficiency, ACS Nanosci. Au, 2022, 2, 93–101 CrossRef CAS PubMed.
- H. Arfin, A. S. Kshirsagar, J. Kaur, B. Mondal, Z. Xia, S. Chakraborty and A. Nag, ns2 electron (Bi3+ and Sb3+) doping in lead-free metal halide perovskite derivatives, Chem. Mater., 2020, 32, 10255–10267 CrossRef CAS.
- F. Locardi, M. Cirignano, D. Baranov, Z. Dang, M. Prato, F. Drago, M. Ferretti, V. Pinchetti, M. Fanciulli, S. Brovelli, L. De Trizio and L. Manna, Colloidal synthesis of double perovskite Cs2AgInCl6 and Mn-doped Cs2AgInCl6 nanocrystals, J. Am. Chem. Soc., 2018, 140, 12989–12995 CrossRef CAS PubMed.
- D. Manna, T. K. Das and A. Yella, Tunable and stable white light emission in Bi3+-alloyed Cs2AgInCl6 double perovskite nanocrystals, Chem. Mater., 2019, 31, 10063–10070 CrossRef CAS.
- Y. Liu, Y. Jing, J. Zhao, Q. Liu and Z. Xia, Design optimization of lead-free perovskite Cs2AgInCl6:Bi nanocrystals with 11.4% photoluminescence quantum yield, Chem. Mater., 2019, 31, 3333–3339 CrossRef CAS.
- Y. Liu, M. S. Molokeev and Z. Xia, Lattice doping of lanthanide ions in Cs2AgInCl6 nanocrystals enabling tunable photoluminescence, Energy Mater. Adv., 2021, 2585274 CAS.
- C. Homann, L. Krukewitt, F. Frenzel, B. Grauel, C. Würth, U. Resch-Genger and M. Haase, NaYF4:Yb, Er/NaYF4 core/shell nanocrystals with high upconversion luminescence quantum yield, Angew. Chem., Int. Ed., 2018, 57, 8765–8769 CrossRef CAS PubMed.
- H. X. Mai, Y. W. Zhang, L. D. Sun and C. H. Yan, Highly efficient multicolor up-conversion emissions and their mechanisms of monodisperse NaYF4:Yb, Er core and core/shell-structured nanocrystals, J. Phys. Chem. C, 2007, 111, 13721–13729 CrossRef CAS.
- Z. Rao, Q. Li, Z. Li, L. Zhou, X. Zhao and X. Gong, Ultra-high-sensitive temperature sensing based on Er3+ and Yb3+ co-doped lead-free double perovskite microcrystals, J. Phys. Chem. Lett., 2022, 13, 3623–3630 CrossRef CAS PubMed.
- Y. Pei, D. Tu, C. Li, S. Han, Z. Xie, F. Wen, L. Wang and X. Chen, Boosting near-infrared luminescence of lanthanide in Cs2AgBiCl6 double perovskites via breakdown of the local site symmetry, Angew. Chem., Int. Ed., 2022, 61, e202205276 CrossRef CAS PubMed.
- Z. Xu, L. Chen, L. Zhang, S. Jing, B. Zhuang, W. Xu and D. Chen, Yb/Er: Cs2Ag(In/Bi)Cl6 lead-free double perovskite for dual-modal optical temperature sensing, J. Lumin., 2022, 248, 118996 CrossRef CAS.
- K. Du, M. Zhang, Y. Li, H. Li, K. Liu, C. Li, J. Feng and H. Zhang, Embellishment of upconversion nanoparticles with ultrasmall perovskite quantum dots for full-color tunable, dual-modal luminescence anticounterfeiting, Adv. Opt. Mater., 2021, 9, 2100814 CrossRef CAS.
- P. J. Sugumaran, J. Zhang and Y. Zhang, Synthesis of stable core-shell perovskite based nano-heterostructures, J. Colloid Interface Sci., 2022, 628, 121–130 CrossRef CAS PubMed.
- H. Xiao, B. Liu, L. Qiu, G. Li, G. Zhang, D. Huang, Y. Zhao, C. Yang, F. Jiang, P. Dang, H. Lian, Z. Cheng and J. Lin, Core–shell structured upconversion/lead-free perovskite nanoparticles for anticounterfeiting applications, Angew. Chem., Int. Ed., 2022, 61, e202115136 CrossRef CAS PubMed.
- C. Zhang, J. Chen, L. Kong, L. Wang, S. Wang, W. Chen, R. Mao, L. Turyanska, G. Jia and X. Yang, Core/shell metal halide perovskite nanocrystals for optoelectronic applications, Adv. Funct. Mater., 2021, 31, 2100438 CrossRef CAS.
- N. Chen, T. Cai, W. Li, K. Hills-Kimball, H. Yang, M. Que, Y. Nagaoka, Z. Liu, D. Yang, A. Dong, C. Y. Xu, R. Zia and O. Chen, Yb- and Mn-doped lead-free double perovskite Cs2AgBiX6 (X = Cl−, Br−) nanocrystals, ACS Appl. Mater. Interfaces, 2019, 11, 16855–16863 CrossRef CAS PubMed.
- E. Cui, X. Yuan, L. Tang, L. Yang, X. Yang, X. Liao, J. Tang, Y. Zhao, W. Sun, K. Liu, Y. Liu and J. Liu, Eu3+, Tb3+ doping induced tunable luminescence of Cs2AgInCl6 double perovskite nanocrystals and its mechanism, Appl. Surf. Sci., 2023, 609, 155472 CrossRef CAS.
- P. Capdevielle, A. Lavigne, D. Sparfel, J. Baranne-Lafont, K. C. Nguyen and M. Maumy, Mechanism of primary aliphatic amines oxidation to nitriles by the cuprous chloride - dioxygen - pyridine system, Tetrahedron Lett., 1990, 31, 3305–3308 CrossRef CAS.
- Y. Xie, Y. Song, G. Sun, P. Hu, A. Bednarkiewicz and L. Sun, Lanthanide-doped heterostructured nanocomposites toward advanced optical anti-counterfeiting and information storage, Light: Sci. Appl., 2022, 11, 150 CrossRef CAS PubMed.
- J. Xu, W. Huang, P. Li, D. R. Onken, C. Dun, Y. Guo, K. B. Ucer, C. Lu, H. Wang, S. M. Geyer, R. T. Williams and D. L. Carroll, Imbedded nanocrystals of CsPbBr3 in Cs4PbBr6: Kinetics, enhanced oscillator strength, and application in light-emitting diodes, Adv. Mater., 2017, 29, 1703703 CrossRef PubMed.
|
This journal is © the Partner Organisations 2024 |
Click here to see how this site uses Cookies. View our privacy policy here.