DOI:
10.1039/D3QM01115A
(Research Article)
Mater. Chem. Front., 2024,
8, 287-296
Activating the electrode–electrolyte interface via a ZnO@black phosphorus modulation layer for dendrite-free Li metal anodes†
Received
13th October 2023
, Accepted 26th October 2023
First published on 31st October 2023
Abstract
Lithium metal is the most promising anode for high energy density batteries. However, lithium dendrite growth resulting from poor interface activity seriously hampers its practical application. An effective method to suppress the Li dendrite growth is to increase the interface activity by enhancing the interface kinetics and reducing lateral Li atom diffusion energy barriers. Herein, ZnO-encapsulated black phosphorus (ZnO@BP) nanosheets are synthesized and utilized as a modulation layer to inhibit the growth of lithium dendrites. The ZnO@BP modulation layer incorporates the P species with moderate electronegativity, which enhances the interface kinetics. This facilitates rapid Li+ desolvation and migration ensuring a uniform Li+ flux. The lithiophilic ZnO contributes to the homogeneous nucleation of lithium and increases the lateral Li atom diffusion rate along the electrode. Consequently, the ZnO@BP coated Li metal anodes exhibit enhanced interface kinetics and outstanding cycle stability in symmetric cells. Furthermore, full cells coupled with LiFePO4 exhibit enhanced rate capacities and cycling stabilities. These results demonstrate the excellent potential of the ZnO@BP modulation layer in activating the electrode–electrolyte interface for stable Li metal anodes, providing opportunities for the application of lithium metal in high energy density devices.
1. Introduction
The lithium metal anode has recently regained the attention of researchers due to its lowest electrochemical potential (−3.045 V vs. the standard hydrogen electrode) and high theoretical capacity (3860 mA h g−1), making it highly desirable for high energy density devices.1–6 Despite its numerous advantages, the commercialization of the lithium metal anode is still hampered by severe lithium dendrite growth.7–10 The lithium dendrites usually cause a series of critical issues in the anode, such as volume expansion and dead lithium, ultimately leading to battery failure.11,12 Furthermore, the aggressive lithium dendrite growth can even penetrate the separator, causing serious safety concerns.13
A previous study reveals that the lithium dendrite growth is primarily attributed to low electrode–electrolyte interface activity.8,14 This low interface activity is mainly manifested in two aspects: (1) the sluggish interface kinetics of Li+ desolvation and diffusion15–18 and (2) high surface diffusion barriers for Li adatoms.7,19,20 Regarding the first issue, the sluggish interface kinetics of Li+ desolvation and diffusion exacerbates the severe fluctuation of Li+ flux. The fluctuation of Li+ flux initially arises from the native solid electrolyte interface (SEI).21,22 However, the sluggish interface kinetics results in an insufficient amount of naked lithium ions at the interface for Li deposition, leading to Li+ aggregation in areas with high local current density.23 This further exacerbates the severe fluctuation of Li+ flux and uneven Li deposition. As for the second issue, the growth of Li dendrites is an inherent property of Li metal.24 High surface diffusion barriers for Li adatoms cause radial Li growth along the preferential crystallographic orientation, forming 1D dendrites.25 Subsequently, lithium ions aggregate at these preferentially formed 1D dendrites due to the comparatively shorter diffusion pathway and stronger electric field. This further aggravates the heterogeneity of Li+ flux and dendrite growth.
To solve these problems, creating a uniform modulation layer with (1) enhanced interface kinetics and (2) high surface Li-atom affinity to activate the anode–electrolyte interface is probably a feasible way. The uniform modulation layer with enhanced interface kinetics promotes the Li+ desolvation and diffusion. This alleviates the concentration polarization of interface Li+ due to the rapid Li+ dispersion and migration. Meanwhile, the high interface affinity for lithium atoms facilitates the uniform Li nucleation and accelerates the lateral diffusion rate of lithium adatoms, which inhibits the formation of 1D dendrites. The synergistic effect between enhanced interface kinetics and high interface affinity for lithium atoms ensures the rapid and uniform distribution of the Li+ flux to the nucleation sites and promotes the lateral lithium growth, thus achieving uniform Li deposition.26,27
Herein, we design ZnO-encapsulated black phosphorus (ZnO@BP) nanosheets as a modulation layer on the Li anode surface with enhanced interface kinetics and high surface Li-atom affinity. In the ZnO@BP modulation layer, P species with moderate electronegativity promote Li+ desolvation and migration, which enhances the interface kinetics along with a steady Li+ flux.22,28 Meanwhile, the lithiophilic ZnO facilitates the uniform Li nucleation and increases the lateral Li atom diffusion rate.29–31 Due to the synergistic effect between BP and ZnO, uniform Li deposition is achieved. Consequently, Li metal anodes with the ZnO@BP nanosheet coating (ZnO@BP/Li) exhibit enhanced interface kinetics and excellent cycling performances in symmetric cells. Moreover, full cells coupled with LiFePO4 (LFP) cathodes exhibit enhanced rate capacities and cycling stabilities, demonstrating the potential application of ZnO@BP/Li anodes in high-energy density batteries.
2. Results and discussion
2.1 Characterization of ZnO@BP nanosheets and ZnO@BP/Li
Hybrid ZnO@BP nanosheets, composed of amorphous ZnO on 2D BP, were prepared using a simple one-step solvothermal method. In this method, the metal salts of Zn(Ac)2·2H2O and few-layer BP nanosheets were mixed in diethylene glycol and then heated to 150 °C for 30 minutes. The excellent affinity of BP for metal ions facilitated the uniform formation of ZnO on the surface of BP.32 This affinity arises from the Lewis acid base interactions, where the lone pair electrons on the black phosphorus surface act as Lewis acids, attracting Lewis base metal ions.33 Fig. S1a (ESI†) displays a typical transmission electron microscopy (TEM) image of few-layer BP nanosheets. The scanning electron microscopy (SEM) and TEM images (Fig. 1a and b) reveal that the 2D structure is preserved after solvent heating. The diffused spots of the selected area electron diffraction (SAED) pattern only match with BP (Fig. 1c). In the high resolution transmission electron microscope (HRTEM) image (Fig. 1d), lattice fringes are observed only at the edges of the nanosheets corresponding to the (111) facet of BP. Energy dispersive X-ray (EDX) mapping (Fig. 1e) demonstrates the uniform distribution of Zn, O and P over the nanosheets. Moreover, the X-ray diffraction (XRD) pattern only gives the diffraction peaks of BP (JCPDS no. 73-1358). These results indicate that Zn species are amorphous. However, after annealing at 800
°C in an Ar atmosphere, diffraction peaks of ZnO and Zn2P2O7 emerge (Fig. S2, ESI†), indicating that zinc primarily exists in the form of ZnO. These results suggest that the BP nanosheets are encapsulated by amorphous ZnO. Atomic force microscopy (AFM) was used to measure the thickness of nanosheets. The AFM image (Fig. S1b, ESI†) reveals that the thickness of BP is ∼4 nm, while after solvent heating, the thickness of the nanosheets increases to ∼8 nm (Fig. 1f), indicating that the thickness of ZnO is ∼2 nm. The ZnO@BP nanosheets exhibit a Brunauer–Emmett–Teller (BET) specific surface of 40.38 m2 g−1 (Fig. S3, ESI†), which is higher than that of pure BP (21.99 m2 g−1). The increased specific surface can be attributed to the ZnO layer preventing the aggregation of nanosheets. Raman spectroscopy (Fig. S4, ESI†) of ZnO@BP shows three peaks at 361, 438, and 466 cm−1, which can be assigned to the A1g, B2g and A2g vibration modes of BP, respectively.34 When compared with pure BP, the interface interaction between BP and ZnO results in a slight blue shift of the three peaks, indicating a tight bonding interaction between BP and ZnO.35 X-Ray photoelectron spectroscopy (XPS) was performed to analyze the surface valence states of ZnO@BP. In the high-resolution spectrum of Zn 2p (Fig. 1h), the peaks located at around 1045.5 and 1022.4 eV can be ascribed to Zn 2p3/2 and Zn 2p1/2 of Zn2+.36 The O 1s spectrum (Fig. S5, ESI†) can be fitted with two peaks: the peak located at 530.1 eV is attributed to O2− in ZnO, while the peak at 531.6 eV arises from the P–O bond due to the oxidation of BP.36,37 The P 2p spectrum can be fitted with three peaks at 130.1, 130.9 and 134.5 eV, which can be assigned to P 2p3/2 and P 2p1/2 of BP, and P–O bonds, respectively.34 All these results confirm the successful synthesis of ZnO@BP nanosheets.
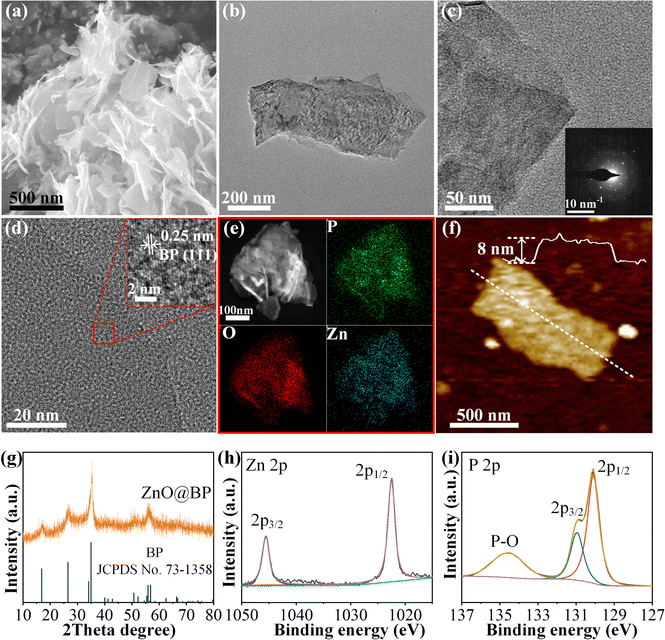 |
| Fig. 1 Morphological characterization of ZnO@BP: (a) SEM and (b) and (c) TEM images, the inset of (c) is the SAED pattern image, (d) HRTEM image, (e) HAADF mapping, (f) AFM image, (g) XRD patterns, (h) and (i) high-resolution XPS spectra of Zn 2p and P 2p. | |
To construct a stable modulation layer, ZnO@BP nanosheets were dispersed in dimethyl carbonate and subsequently dropped onto the electrode surface. The SEM image and EDS mapping of ZnO@BP nanosheets (Fig. S6a, ESI†) coated on the lithium metal anode (ZnO@BP/Li) reveal that the surface of the lithium metal is densely covered by ZnO@BP nanosheets. In the further magnified SEM images (Fig. S6b and c, ESI†), the nanosheets are observed to be tightly and flatly laid on the electrode surface. The cross-section SEM image shows that the ZnO@BP modulation layer tightly adheres to the lithium metal surface with a thickness of ∼1.2 μm (Fig. S6d, ESI†). Fig. S7 (ESI†) demonstrates that bare Li exhibits a contact angle of 48° between the Li metal surface and electrolyte. In contrast, with the modification of the ZnO@BP modulation layer, the electrolyte spreads directly on the surface of the electrode. This super wettability of ZnO@BP/Li prevents the polarization of Li+ on the electrode surface, mitigating dendrite formation.15,22,38 Furthermore, XPS analysis was conducted on Li metal anodes with and without the protection of the ZnO@BP modulation layer to determine the composition of the electrode surface (Fig. S8, ESI†). In the case of bare Li, the Li 1s XPS spectrum peak located at 55.6 eV corresponds to Li–O bonds, indicating that lithium oxide is the main component of the electrode surface.39 Lithium oxide typically delivers low ionic conductivity, which hinders the Li+ transport, leading to the accumulation of Li ions in the areas of higher current density, resulting in uncontrollable dendrite formation.23 For ZnO@BP/Li, in the Zn 2p XPS spectrum, the two peaks attributed to Zn 2p are shifted to 1020.5 and 1043.4 eV, respectively, corresponding to the LixZn alloy.40 In the P 2p XPS spectrum, the 2p1/2 and 2p3/2 peaks of P exhibit a blue shift of 1.5 eV compared with the initial ZnO@BP nanosheets. Moreover, in the Li 1s XPS spectrum, a peak ascribed to Li–P bonds emerges, and lithium phosphide becomes the dominant component for fast Li+ distribution.41 These results demonstrate the robust interaction between ZnO@BP and the lithium metal anode. By employing the ZnO@BP modulation layer coating, an LiyP/LixZn protective layer can form on the Li metal anode surface, regulating the deposition behavior of lithium.
2.2 Electrochemical kinetics
ZnO@BP nanosheets are intentionally prepared because they can enhance interface kinetics and induce uniform lithium deposition. In a liquid electrolyte, the Li+ migration is typically accompanied by two to six solvent molecules.1 Desolvation of the solvated lithium ion is generally considered as the rate control step that requires conquering a high energy barrier before deposition onto the electrode surface.15 The moderate electronegativity of P species accelerates the Li+ desolvation and migration, resulting in enhanced interface kinetics along with a steady Li+ flux.28 Meanwhile, high surface diffusion barriers for Li adatoms cause Li to deposit along the preferential crystallographic orientation to form 1D dendrites. Subsequently, the lithium ions aggregate at preferentially formed 1D dendrites due to the comparatively short diffusion pathway and strong electric field. The lithiophilic ZnO facilitates uniform Li nucleation and increases the Li-atom lateral diffusion rate, inhibiting the formation of initial 1D dendrites. Therefore, the ZnO@BP modulation layer ensures the rapid and uniform distribution of the Li+ flux at the nucleation sites and promotes the lateral lithium growth. To verify the assumptions, XPS was first performed on ZnO@BP after immersion in an ethanol solution of lithium bromide (LiBr) for 2 h to investigate the interaction between lithium ions and ZnO@BP nanosheets. In P 2p high resolution spectra (Fig. 2a), a red shift of 0.2 eV is observed for the 2p1/2 and 2p3/2 peaks of BP after Li-salt treatment, indicating the electron donation behavior of P species due to the strong interaction between BP and Li+.22 Furthermore, the Li 1s spectrum (Fig. 2b) can be well fitted as two peaks. The peak at 56.9 eV corresponds to Li–Br bonds, originating from residual LiBr compounds, while the peak located at 56.2 eV is attributed to Li–P bonds.15,41 Furthermore, in the Raman spectra, when ZnO@BP is immersed in the electrolyte, the peak arising from the A2g vibration of BP shows a blue shift, which can be attributed to the interaction between Li+ and P species. To further verify the role of the ZnO@BP nanosheets in promoting the solvent removal process, cyclic voltammetry (CV) was performed at varying scan rates on Li|Cu cells (Fig. S9, ESI†). The relationship between the peak current (i) and scan rate (v) can be articulated as: i = avb, where the value of b offers insight into the charge storage mechanism. With the coating of the ZnO@BP modulation layer, the value of b escalates from 0.318 to 0.608 (Fig. 2d). This suggests that the pristine diffusion-limited process might be partially transformed into a surface-controlled process with the assistance of the ZnO@BP modulation layer, indicating an adequate naked-Li+ source in close proximity to the lithium surface during Li plating.42 The nucleation overpotentials were measured to demonstrate the positive role of ZnO. As shown in Fig. 2e, the half cell with ZnO@BP/Cu exhibits a small overpotential of 9 mV, whereas the cells with Cu foil and BP/Cu show higher overpotentials of 53 and 32 mV, respectively. Fig. 2f presents the Tafel plots and the corresponding exchange current densities (j0). The cell with ZnO@BP/Li demonstrates an exchange current density of 3.52 mA cm−2, which is higher than those of the cells with bare Li (1.98 mA cm−2) and BP/Li (0.88 mA cm−2). The activation energy of Li deposition is also studied using electrochemical impedance spectroscopy (EIS) at different temperatures (Fig. S10, ESI† and Fig. 2g). The calculated activation energies for bare Li, BP/Li and ZnO@BP/Li are calculated as 7.88, 6.75 and 6.21 kJ mol−1, respectively, confirming the active role of the ZnO@BP modulation layer in promoting uniform lithium deposition.43,44
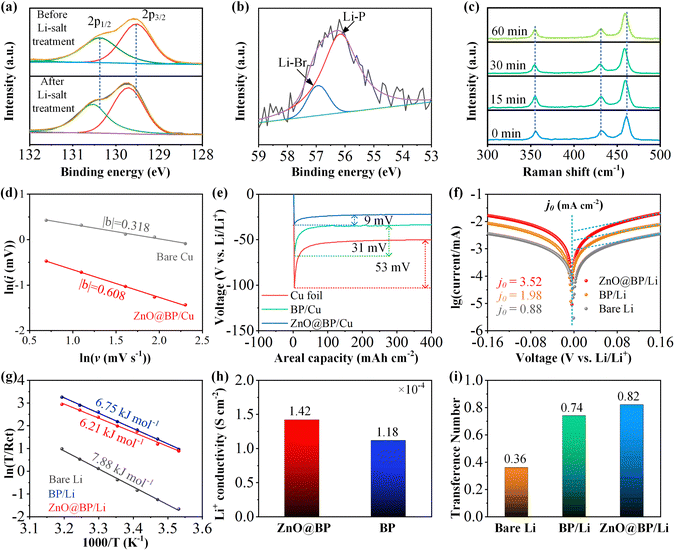 |
| Fig. 2 (a) P 2p and (b) Li 1s XPS spectra of ZnO@BP after Li-salt solution treatment, (c) Raman spectra of ZnO@BP before and after being immersed in electrolyte, (d) linear fitting of lni to lnv from the CVs at different scan rates of Li|Cu cells with or without the ZnO@BP modulation layer, (e) nucleation overpotentials and (f) Tafel plots for different electrodes, (g) activation energy of Li+ deposition, (h) Li+ conductivities of BP and ZnO@BP, and (i) transference numbers on different electrodes. | |
The Li+ conductivity of the ZnO@BP layer was evaluated to further study the role of the ZnO@BP nanosheets in Li+ migration by the EIS measurement (Fig. S11, ESI† and Fig. 2h). The ZnO@BP layer exhibits a higher Li+ conductivity of 1.42 × 10−4 S cm−1 compared to BP (1.18 × 10−4 S cm−1), due to the prevention of nanosheet aggregation by ZnO.45 The Li+ transference numbers, an important parameter to evaluate the Li+ migration ability, were further determined using the Bruce–Vincent–Evans equation (Fig. S12, ESI† and Fig. 2i). In the Li symmetric cell, the migration of lithium ions in solvents accompanied by two to six solvent molecules results in a slower migration speed than that of anions, leading to a low Li+ transference number of 0.36. However, with BP coating, the Li+ transference number rapidly increases to 0.74 due to the moderate electronegativity of P species. Furthermore, benefiting from the good dispersibility of the ZnO@BP nanosheets, the cell with ZnO@BP/Li achieves the highest Li+ transference number of 0.82. These results demonstrate the excellent Li+ migration behavior of the ZnO@BP modulation layer.
2.3 Li deposition behavior
To evaluate the effectiveness of the ZnO@BP modulation layer for uniform Li deposition, Li|Cu half cells were assembled with Cu foil, BP coated Cu foil (BP/Cu) and ZnO@BP coated Cu foil (ZnO@BP/Cu) electrodes. The stability of the cells was evaluated by measuring the coulombic efficiency (CE), which represents the ratio of the amount of Li plating to the amount of Li stripping. In the case of the Cu foil, uneven Li deposition leads to severe side reactions, resulting in irreversible capacity loss (Fig. S13, ESI†). After only 60 cycles, a rapid decay of CE happens on Cu foil at a current density of 1 mA cm−2 for 1 mAh cm-2. In contrast, the cell with ZnO@BP/Cu achieves the highest coulombic efficiency (CE) of 98.5% after 150 cycles with stable voltage hysteresis. This performance is superior to that of the cell with BP/Cu, indicating the superiority of the ZnO@BP modulation layer in promoting uniform lithium deposition and suppressing lithium dendrite growth.
The long-term cycling stability and voltage polarization during Li plating/stripping were evaluated for symmetric cells based on bare Li, BP/Li and ZnO@BP/Li. As shown in Fig. 3a, the symmetric cell with bare Li exhibits an overpotential of 35 mV which starts to increase after 220 h at a current density of 1 mA cm−2 with a fixed deposition capacity of 1 mA h cm−2. The instability is attributed to the lithium dendrite growth blocking Li+ migration and exhausting the electrolyte. After BP coating, the lifespan of the symmetric cell extends to 450 h with a smaller voltage hysteresis of 20 mV. The improvement is attributed to the ability of P species to accelerate Li+ solvation and migration along with uniform lithium ion distribution, thereby partially inhibiting the growth of dendrites. Furthermore, with the assistance of ZnO, which promotes uniform lithium nucleation and lateral Li atom diffusion rate, the symmetric cell with ZnO@BP/Li successfully achieves an ultralong cycling stability for 1200 h with a low polarization potential of 15 mV. Due to the assistance of the ZnO@BP modulation layer, when the current density is further increased to 3 mA cm−2 at an areal capacity of 3 mA h cm−2 (Fig. 3b), the symmetric cell with ZnO@BP/Li still maintains a long term stability of 500 h with a low voltage hysteresis of 26 mV. In contrast, the cells with bare Li and BP/Li exhibit erratic voltage behavior after only 20 and 100 h of cycling, due to the uncontrollable dendrite growth. The rate performance of the symmetric cells is further evaluated at different current densities and at an areal capacity of 1 mA h cm−2 (Fig. 3c). As expected, the ZnO@BP/Li based symmetric cell delivers the lowest overpotentials at successively increased current densities from 0.2 to 5 mA cm−2, indicating the steady Li plating/stripping processes. Moreover, when current densities return to 0.5 and 3 mA cm−2, the voltage hysteresis of the ZnO@BP/Li based symmetric cell returns to the previous level, indicating the high reversibility of lithium platting/stripping. In contrast, the cells with bare Li and BP/Li exhibit high overpotentials and erratic voltage behaviors especially at high current densities. These results indicate the energetic roles of ZnO@BP in steady Li plating/stripping. To meet the requirements for energy density, practical cells may be fabricated using high-voltage cathodes and carbonate electrolytes. The long-term cycling stability of the symmetric cell was also evaluated in the EC/DEC electrolyte (Fig. S14, ESI†). Despite the degraded cycling stability due to the poor reduction stability of EC/DEC, the symmetric cell based on ZnO@BP/Li still runs stably for over 550 h with a small overpotential.46 In contrast, the symmetric cells with bare Li and BP/Li failed within 100 and 400 h, respectively. This result indicates that the ZnO@BP/Li modulation layer can promote uniform Li deposition even under harsh conditions.
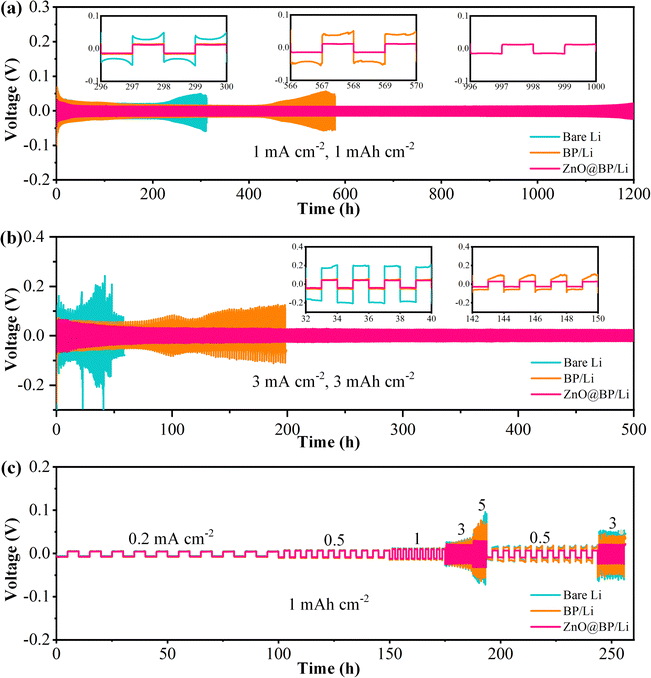 |
| Fig. 3 Cycling performance of symmetric cells with bare Li, BP/Li and ZnO@BP/Li in DOL/DME electrolyte. Voltage profiles at (a) 1 mA cm−2 for 1 mA h cm−2 and (b) 3 mA cm−2 for 3 mA h cm−2. (c) Rate performance with a fixed capacity of 1 mA h cm−2. | |
The morphology evolution of various Li metal anodes was observed by SEM. In the case of bare Li (Fig. 4a), a rugged surface with plenty of fractal Li was observed after 20 cycles at a current density of 1 mA cm−2 with a fixed deposition capacity of 1 mA h cm−2. After 100 cycles, large portions of dendrites were significantly formed, leading to rapid consumption of the electrolyte and pulverization of the electrode surface. The cross-sectional view shows a thickness of 86 μm for Li deposition, which is due to the severe volume expansion caused by dendrite growth. With the BP coating (Fig. S15, ESI†), only a few dendrites were observed on the electrode surface after 20 cycles. As the cycle progressed, chunky Li, proven to be beneficial for improving battery life, was observed with a thickness of 26 μm after 100 cycles.47,48 In contrast, with the protection of ZnO@BP, the electrode exhibited uniform and flat Li deposition after 20 cycles. Even after 100 cycles, a smooth surface and dendrite-free morphology were maintained with a deposition layer of 6 μm. Additionally, EDS mapping of cross section after 100 cycles shows that P and Zn species predominantly reside on the surface of the electrode (Fig. S16, ESI†), indicating that Li prefers to deposit beneath the modulation layer. Consequently, it is inferred that the lithiated modulation layer remains intact after long cycles, thereby facilitating stable Li plating/stripping. The structural evolution upon deposition and the corresponding mechanisms of bare Li and ZnO@BP/Li are schematically illustrated in Fig. 4g and h. Typically, the lithium metal surface spontaneously forms a native SEI layer due to the high chemical reactivity of lithium. However, the native SEI is characterized by poor Li+ conduction, chemical heterogeneity, and fragility, inevitably leading to an uneven Li+ flux and dendrite growth. Furthermore, severe volume fluctuation caused by lithium dendrite growth leads to a serious and continuous crack of native SEI films, causing continuous decomposition of the electrolyte and low Li+ transference numbers, eventually leading to battery failure. In contrast, the ZnO@BP modulation layer enhances the interface kinetics and increases surface affinity for Li atoms. This promotes the flat and compact deposition of lithium, improving the stability of the Li metal anode.
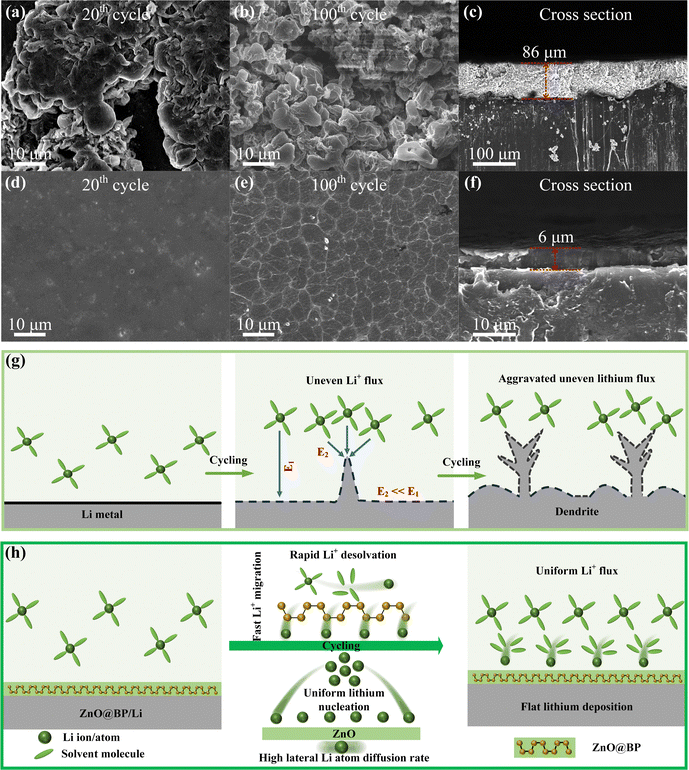 |
| Fig. 4
Ex situ SEM images of bare Li and ZnO@BP/Li disassembled from symmetric cells after cycling. (a) and (b) Top views of the bare Li electrode after (a) 20 cycles and (b) 100 cycles. (d) and (e) Top views of the ZnO@BP/Li electrode after (d) 20 cycles and (e) 100 cycles. Cross-sections of (c) bare Li and (f) ZnO@BP/Li electrodes after 100 cycles. Schematic illustration of structural evolution upon deposition and the corresponding mechanisms over (g) bare Li and (h) ZnO@BP/Li electrodes. | |
The Li–electrolyte interface was further investigated through EIS measurements on symmetric cells based on different electrodes at various stages. For the bare Li symmetric cell, a decreased interface resistance (including RSEI and Rct) was observed in the first 50 cycles, followed by a rapid increase after 100 cycles (Fig. S17a, ESI†). Initially, the formation of a fractal lithium morphology on the electrode surface resulted in a significant increase in the contact surface with the electrolyte. However, as the cycle went on, the gradually thickened passivation layer and lithium dendrites hindered the lithium ion transfer. Thus, the interface resistance firstly decreased and then rapidly increased.23,49,50 On the other hand, with the BP and ZnO@BP coating, the interface resistance decreased in the first 50 cycles and remained stable in the subsequent cycles (Fig. S17b and c, ESI†). This indicated the excellent stability of interfaces during Li plating/stripping.48,51 Moreover, the cell-based on ZnO@BP/Li exhibited the lowest interface resistance at all stages, suggesting the enhanced interface kinetics.
2.4 Full cell performances
To assess the practical application of ZnO@BP/Li anodes, full cells paired with commercialized LFP cathodes were assembled. In Fig. 5a and Fig. S18 (ESI†), it can be observed that the full cells with bare Li, BP/Li and ZnO@BP/Li initially exhibit similar specific capacities of about 150 mA h g−1 at 0.5C. However, as the charge and discharge process continues, the specific capacities of the bare Li|LFP and BP/Li|LFP full cells start to decay within 200 cycles with low coulombic efficiencies. In contrast, the ZnO@BP/Li|LFP cell demonstrates high specific capacity retention even after 350 cycles. Furthermore, the ZnO@BP/Li|LFP cell exhibits excellent rate capability performance (Fig. 5b and Fig. S19, ESI†). Even at 10C, the cell still maintains a high reversible specific capacity of 95 mA h g−1, surpassing those of Li|LFP and BP/Li|LFP. Additionally, the ZnO@BP/Li|LFP full cell exhibits an outstanding long-term stability of 1600 cycles at 2C with stable charge–discharge profiles (Fig. 5c and Fig. S20, ESI†). The full cell performances of ZnO@BP/Li anodes were further investigated at a high LFP mass loading of 10.8 mg cm−2. The ZnO@BP/Li|LFP full cell maintains a high reversible specific capacity of 155 mA h g−1 (Fig. 5d and Fig. S21, ESI†) due to the enhanced interface kinetics and homogeneous lithium deposition, while a cliff drop happens on the bare Li |LFP full cell within 40 cycles at 0.2C. Notably, long cycle stability is achieved at 1C. The ZnO@BP/Li|LFP full cell stably runs for over 400 cycles with stable charge–discharge profiles (Fig. 5e and Fig. S22, ESI†). The surface morphologies of bare Li and ZnO@BP/Li anodes after cycling in the Li|LFP full cells were observed by SEM (Fig. S23, ESI†). The bare Li anode exhibits a rough surface with aggressive lithium dendrites, while ZnO@BP/Li exhibits flat and smooth morphologies, confirming the excellent electrochemical superiority of the ZnO@BP/Li anode due to the well-suppressed Li dendrites.
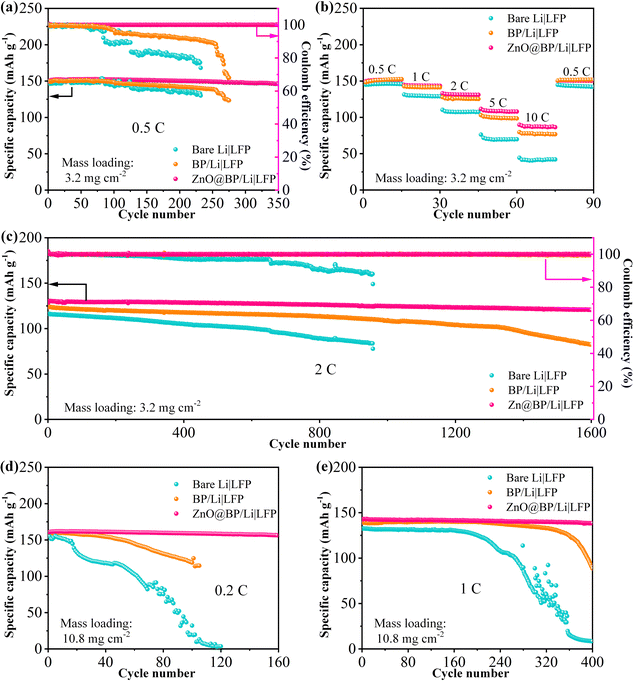 |
| Fig. 5 (a)–(c) Cycling performance of full cells with an LPF mass loading of 3.2 mg cm−2: (a) 0.5C, (b) rate performance, and (c) 2C. (d) and (e) Cyclic performance of full cells with an LPF mass loading of 10.8 mg cm−2: (d) 0.2C and (e) 1C. | |
3. Conclusion
In conclusion, our study presents a promising strategy for enhancing the performance of metal lithium anodes by utilizing a uniform ZnO@BP modulation layer directly coated on the Li anode surface. The incorporation of electronegative P species within the BP component improves the interface kinetics of Li+ desolvation and migration, ensuring a uniform flow of Li+ into the bulk Li metal. Additionally, the lithiophilic ZnO enhances the surface Li-atom affinity and facilitates the lateral diffusion of lithium atoms, effectively preventing the formation of initial lithium tips and inhibiting dendrite formation. As a result, the ZnO@BP coated Li metal anodes (ZnO@BP/Li) exhibit exceptional stability with minimal voltage hysteresis. Furthermore, the outstanding electrochemical performances of the full cells, even at high LFP mass loadings, highlight the tremendous potential of this approach in the development of high energy density devices. These groundbreaking findings provide valuable insights for developing the next-generation high energy density energy storage devices.
Conflicts of interest
There are no conflicts to declare.
Acknowledgements
This study is financially supported by the National Nature Science Foundation of China (2272142), the Fundamental Research Funds for the Central Universities (20720220031), the 111 Project (B16029), the Open Innovation Fund for undergraduate students of Xiamen University (KFJJ-202223), and the Shccig-Qinling Program (SMYJY20230234).
References
- X. B. Cheng, R. Zhang, C. Z. Zhao and Q. Zhang, Toward Safe Lithium Metal Anode in Rechargeable Batteries: A Review, Chem. Rev., 2017, 117, 10403–10473 CrossRef CAS PubMed.
-
S. Li, J. Yang and Y. Lu, Lithium Metal Anode, Encyclopedia of Inorganic and Bioinorganic Chemistry, Wiley online library, 2019, pp. 1–21 DOI:10.1002/9781119951438.eibc2677.
- J. Wang, B. Ge, H. Li, M. Yang, J. Wang, D. Liu, C. Fernandez, X. Chen and Q. Peng, Challenges and progresses of lithium-metal batteries, Chem. Eng. J., 2021, 420, 129739 CrossRef CAS.
- Y. Hyeon, J. Lee, H. Qutaish, S. A. Han, S. H. Choi, S. W. Moon, M.-S. Park, D. Whang and J. H. Kim, Lithium metal storage in zeolitic imidazolate framework derived nanoarchitectures, Energy Storage Mater., 2020, 33, 95–107 CrossRef.
- Y. Liu, Z. Song, Z. Wang, J. Xing, W. Zou and J. Li, Regulating Li nucleation/deposition by bamboo-shoot like lithiophilic particles anchored on carbon cloth for a dendrite-free lithium metal anode, Mater. Chem. Front., 2023, 7, 117–127 RSC.
- Y. Qi, X. Zeng, L. Xiao, X. Li, H. Liao, Q. Xu and J. Xu, An invisible hand: Hydrogen bonding guided synthesis of ultrathin two-dimensional amorphous TiO2 nanosheets, Sci. China Mater., 2022, 65, 3017–3024 CrossRef CAS.
- H. Liu, X.-B. Cheng, Z. Jin, R. Zhang, G. Wang, L.-Q. Chen, Q.-B. Liu, J.-Q. Huang and Q. Zhang, Recent advances in understanding dendrite growth on alkali metal anodes, EnergyChem, 2019, 1, 100003 CrossRef.
- J. Wang, L. Li, H. Hu, H. Hu, Q. Guan, M. Huang, L. Jia, H. Adenusi, K. V. Tian, J. Zhang, S. Passerini and H. Lin, Toward Dendrite-Free Metallic Lithium Anodes: From Structural Design to Optimal Electrochemical Diffusion Kinetics, ACS Nano, 2022, 16, 17729–17760 CrossRef CAS PubMed.
- P. Zhai, L. Liu, X. Gu, T. Wang and Y. Gong, Interface Engineering for Lithium Metal Anodes in Liquid Electrolyte, Adv. Energy Mater., 2020, 10, 2001257 CrossRef CAS.
- X. B. Cheng, R. Zhang, C. Z. Zhao, F. Wei, J. G. Zhang and Q. Zhang, A Review of Solid Electrolyte Interphases on Lithium Metal Anode, Adv. Sci., 2016, 3, 1500213 CrossRef PubMed.
- K. Zhang, G.-H. Lee, M. Park, W. Li and Y.-M. Kang, Recent Developments of the Lithium Metal Anode for Rechargeable Non-Aqueous Batteries, Adv. Energy Mater., 2016, 6, 1600811 CrossRef.
- S. Li, M. Jiang, Y. Xie, H. Xu, J. Jia and J. Li, Developing High-Performance Lithium Metal Anode in Liquid Electrolytes: Challenges and Progress, Adv. Mater., 2018, 30, 1706375 CrossRef PubMed.
- Y. Chen, Y. Luo, H. Zhang, C. Qu, H. Zhang and X. Li, The Challenge of Lithium Metal Anodes for Practical Applications, Small Methods, 2019, 3, 1800551 CrossRef.
- K. Dong, Y. Xu, J. Tan, M. Osenberg, F. Sun, Z. Kochovski, D. T. Pham, S. Mei, A. Hilger, E. Ryan, Y. Lu, J. Banhart and I. Manke, Unravelling the Mechanism of Lithium Nucleation and Growth and the Interaction with the Solid Electrolyte Interface, ACS Energy Lett., 2021, 6, 1719–1728 CrossRef CAS.
- Y. Guo, P. Niu, Y. Liu, Y. Ouyang, D. Li, T. Zhai, H. Li and Y. Cui, An Autotransferable g-C3N4 Li+-Modulating Layer toward Stable Lithium Anodes, Adv. Mater., 2019, 31, 1900342 CrossRef.
- P. Zhai, T. Wang, H. Jiang, J. Wan, Y. Wei, L. Wang, W. Liu, Q. Chen, W. Yang, Y. Cui and Y. Gong, 3D Artificial Solid-Electrolyte Interphase for Lithium Metal Anodes Enabled by Insulator-Metal-Insulator Layered Heterostructures, Adv. Mater., 2021, 33, 2006247 CrossRef CAS.
- F. Hao, A. Verma and P. P. Mukherjee, Mechanistic insight into dendrite-SEI interactions for lithium metal electrodes, J. Mater. Chem. A, 2018, 6, 19664–19671 RSC.
- Z. Wang, Z. Sun, J. Li, Y. Shi, C. Sun, B. An, H. M. Cheng and F. Li, Insights into the deposition chemistry of Li ions in nonaqueous electrolyte for stable Li anodes, Chem. Soc. Rev., 2021, 50, 3178–3210 RSC.
- A. Hagopian, M.-L. Doublet and J.-S. Filhol, Thermodynamic origin of dendrite growth in metal anode batteries, Energy Environ. Sci., 2020, 13, 5186–5197 RSC.
- J. Tan, W. Yao, M. Ye and J. Shen, Atomistic insights into the morphology of deposited Li, J. Mater. Chem. A, 2022, 10, 18577–18591 RSC.
- H. Wu, H. Jia, C. Wang, J. G. Zhang and W. Xu, Recent Progress in Understanding Solid Electrolyte Interphase on Lithium Metal Anodes, Adv. Energy Mater., 2020, 11, 2003092 CrossRef.
- G. Zhang, P. Li, K. Chen, H. Zheng, W. He, L. Xiao, X. Li, Q. Xu, J. Weng and J. Xu, A robust Janus bilayer with tailored ionic conductivity and interface stability for stable Li metal anodes, J. Energy Chem., 2022, 74, 368–375 CrossRef CAS.
- Z. Luo, S. Li, L. Yang, Y. Tian, L. Xu, G. Zou, H. Hou, W. Wei, L. Chen and X. Ji, Interfacially Redistributed charge for robust lithium metal anode, Nano Energy, 2021, 87, 106212 CrossRef CAS.
- Y. Lu, Z. Tu and L. A. Archer, Stable lithium electrodeposition in liquid and nanoporous solid electrolytes, Nat. Mater., 2014, 13, 961–969 CrossRef CAS.
- M. Jackle and A. Gross, Microscopic properties of lithium, sodium, and magnesium battery anode materials related to possible dendrite growth, J. Chem. Phys., 2014, 141, 174710 CrossRef PubMed.
- Z. Chang, Y. Qiao, H. Deng, H. Yang, P. He and H. Zhou, A Liquid Electrolyte with De-Solvated Lithium Ions for Lithium-Metal Battery, Joule, 2020, 4, 1776–1789 CrossRef CAS.
- Z. Tu, S. Choudhury, M. J. Zachman, S. Wei, K. Zhang, L. F. Kourkoutis and L. A. Archer, Designing Artificial Solid-Electrolyte Interphases for Single-Ion and High-Efficiency Transport in Batteries, Joule, 2017, 1, 394–406 CrossRef CAS.
- Y. Kim, D. Koo, S. Ha, S. C. Jung, T. Yim, H. Kim, S. K. Oh, D. M. Kim, A. Choi, Y. Kang, K. H. Ryu, M. Jang, Y. K. Han, S. M. Oh and K. T. Lee, Two-Dimensional Phosphorene-Derived Protective Layers on a Lithium Metal Anode for Lithium-Oxygen Batteries, ACS Nano, 2018, 12, 4419–4430 CrossRef CAS PubMed.
- P. Ma, Z. Zhuang, J. Cao, B. Ju and X. Xi, ZnO-CoO Composite Nanosphere Array-Modified Carbon Cloth for Low-Voltage Hysteresis Li Metal Anodes, ACS Appl. Energy Mater., 2022, 5, 6417–6422 CrossRef CAS.
- D. Y. Jeong, W. J. Chang, S. Jang, J. H. Shin and W. I. Park, Janus Membranes with Graphene Meshes and ZnO Rods for Controlling Dendritic Growth in High-Performance Li Metal Anodes, ACS Appl. Energy Mater., 2022, 5, 4413–4420 CrossRef CAS.
- A. Mohammadi, A. Hagopian, S. Sayegh, M. Bechelany, J.-S. Filhol, R. Younesi, L. Stievano and L. Monconduit, Towards understanding the nucleation and growth mechanism of Li dendrites on zinc oxide-coated nickel electrodes, J. Mater. Chem. A, 2022, 10, 17593–17602 RSC.
- Z. Sofer, D. Sedmidubsky, S. Huber, J. Luxa, D. Bousa, C. Boothroyd and M. Pumera, Layered Black Phosphorus: Strongly Anisotropic Magnetic, Electronic, and Electron-Transfer Properties, Angew. Chem., Int. Ed., 2016, 55, 3382–3386 CrossRef CAS PubMed.
- S. Lei, X. Wang, B. Li, J. Kang, Y. He, A. George, L. Ge, Y. Gong, P. Dong, Z. Jin, G. Brunetto, W. Chen, Z. T. Lin, R. Baines, D. S. Galvao, J. Lou, E. Barrera, K. Banerjee, R. Vajtai and P. Ajayan, Surface functionalization of two-dimensional metal chalcogenides by Lewis acid-base chemistry, Nat. Nanotechnol., 2016, 11, 465–471 CrossRef CAS PubMed.
- J. Plutnar, J. Šturala, V. Mazánek, Z. Sofer and M. Pumera, Fluorination of Black Phosphorus-Will Black Phosphorus Burn Down in the Elemental Fluorine ?, Adv. Funct. Mater., 2018, 28, 1801438 CrossRef.
- X. Li, L. Xiao, L. Zhou, Q. Xu, J. Weng, J. Xu and B. Liu, Adaptive Bifunctional Electrocatalyst of Amorphous CoFe Oxide@2D Black Phosphorus for Overall Water Splitting, Angew. Chem., Int. Ed., 2020, 59, 21106–21113 CrossRef CAS PubMed.
- Y. Qiao, Q. Li, X. B. Cheng, F. Liu, Y. Yang, Z. Lu, J. Zhao, J. Wu, H. Liu, S. Yang and Y. Liu, Three-Dimensional Superlithiophilic Interphase for Dendrite-Free Lithium Metal Anodes, ACS Appl. Mater. Interfaces, 2020, 12, 5767–5774 CrossRef CAS PubMed.
- F. Zhou, L. Ouyang, J. Liu, X.-S. Yang and M. Zhu, Chemical bonding black phosphorus with TiO2 and carbon toward high-performance lithium storage, J. Power Sources, 2020, 449, 227549 CrossRef CAS.
- Y. Zhou, J. Zhang, K. Zhao, Y. Ma, H. Zhang, D. Song, X. Shi, L. Zhang and Y. Ding, A novel dual-protection interface based on gallium-lithium alloy enables dendrite-free lithium metal anodes, Energy Storage Mater., 2021, 39, 403–411 CrossRef.
-
J. R. C. King. and J. Chastain, Handbook of X-ray Photoelectron Spectroscopy, PerkinElmer Corporation, 1992, vol. 40, p. 221 Search PubMed.
- L. Jiang, S. Tan, J. Yang, Z. Xu, S. Zhang, T. Feng, H. Zhou and M. Wu, Zinc atoms introduction alloying to the artificial interface protection layer for ultra-stable LiB alloy anodes, J. Power Sources, 2023, 556, 232373 CrossRef CAS.
- H. Shen, Y. Huang, R. Hao, Y. Chang, Z. Ma, B. Guo, P. Wang, H. Yang, J. Cheng, Q. Li, H. Wang, Z. Liu and A. Nie, Mechanical Robustness Two-Dimensional Silicon Phosphide Flake Anodes for Lithium Ion Batteries, ACS Sustainable Chem. Eng., 2020, 8, 17597–17605 CrossRef CAS.
- V. Augustyn, J. Come, M. A. Lowe, J. W. Kim, P.-L. Taberna, S. H. Tolbert, H. D. Abruña, P. Simon and B. Dunn, High-rate electrochemical energy storage through Li+ intercalation pseudocapacitance, Nat. Mater., 2013, 12, 518–522 CrossRef CAS PubMed.
- Y. Wang, F. Liu, G. Fan, X. Qiu, J. Liu, Z. Yan, K. Zhang, F. Cheng and J. Chen, Electroless Formation of a Fluorinated Li/Na Hybrid Interphase for Robust Lithium Anodes, J. Am. Chem. Soc., 2021, 143, 2829–2837 CrossRef CAS PubMed.
- R. Du, Y. Jie, Y. Chen, F. Huang, W. Cai, Y. Liu, X. Li, S. Wang, Z. Lei, R. Cao, G. Zhang and S. Jiao, Modulating Lithium Nucleation Behavior through Ultrathin Interfacial Layer for Superior Lithium Metal Batteries, ACS Appl. Energy Mater., 2020, 3, 6692–6699 CrossRef CAS.
- X. Zhang, Y. Zhu, A. M. Bruck, L. M. Housel, L. Wang, C. D. Quilty, K. J. Takeuchi, E. S. Takeuchi, A. C. Marschilok and G. Yu, Understanding aggregation hindered Li-ion transport in transition metal oxide at mesoscale, Energy Storage Mater., 2019, 19, 439–445 CrossRef CAS.
- J. Liu, S. Ihuaenyi, R. Kuphal, J. Salinas, L. Xie, L. Yang, U. Janakiraman, M. E. Fortier and C. Fang, A Comparison of Carbonate-Based and Ether-Based Electrolyte Systems for Lithium Metal Batteries, J. Electrochem. Soc., 2023, 170, 010535 CrossRef CAS.
- H. Qiu, T. Tang, M. Asif, W. Li, T. Zhang and Y. Hou, Stable lithium metal anode enabled by lithium metal partial alloying, Nano Energy, 2019, 65, 103989 CrossRef CAS.
- Y. Ma, L. Wei, Y. Gu, L. Zhao, Y. Jing, Q. Mu, Y. Su, X. Yuan, Y. Peng and Z. Deng, Insulative Ion-Conducting Lithium Selenide as the Artificial Solid-Electrolyte Interface Enabling Heavy-Duty Lithium Metal Operations, Nano Lett., 2021, 21, 7354–7362 CrossRef CAS PubMed.
- K. N. Wood, E. Kazyak, A. F. Chadwick, K. H. Chen, J. G. Zhang, K. Thornton and N. P. Dasgupta, Dendrites and Pits: Untangling the Complex Behavior of Lithium Metal Anodes through Operando Video Microscopy, ACS Cent. Sci., 2016, 2, 790–801 CrossRef CAS PubMed.
- K.-H. Chen, K. N. Wood, E. Kazyak, W. S. LePage, A. L. Davis, A. J. Sanchez and N. P. Dasgupta, Dead lithium: mass transport effects on voltage, capacity, and failure of lithium metal anodes, J. Mater. Chem. A, 2017, 5, 11671–11681 RSC.
- T. Chen, W. Kong, P. Zhao, H. Lin, Y. Hu, R. Chen, W. Yan and Z. Jin, Dendrite-Free and Stable Lithium Metal Anodes Enabled by an Antimony-Based Lithiophilic Interphase, Chem. Mater., 2019, 31, 7565–7573 CrossRef CAS.
|
This journal is © the Partner Organisations 2024 |
Click here to see how this site uses Cookies. View our privacy policy here.