DOI:
10.1039/D3RA07654G
(Paper)
RSC Adv., 2024,
14, 2422-2428
Self-assembled nanovesicles based on chiral bis-H8-BINOL for Fe3+ recognition and secondary recognition of L-cysteine by 1 + 1 complex†
Received
9th November 2023
, Accepted 8th January 2024
First published on 12th January 2024
Abstract
A novel fluorescent “off” sensor, R-β-D-1, was obtained in high yield (91.2%) by using octahydronaphthol as a backbone, introducing an alkyne group at the 2-position, and linking azido-glucose via a click reaction. The sensor was analyzed by scanning electron microscopy and transmission electron microscopy and was found to be a self-assembled vesicle. AFM results showed that the fluorescence burst was extinguished by the addition of Fe3+, and the fluorescence was restored by the addition of cysteine. This is due to charge transfer within the molecular structure, resulting in the ICT effect and phototransfer of electrons (PET), as well as redshifting (from 331 nm to 351 nm) and quenching of the fluorescence. The self-assembled vesicles of the fluorescent sensor R-β-D-1 encapsulated Fe3+, but upon addition of cysteine, the vesicles of R-β-D-1–Fe3+ were also complexed with it, forming the R-β-D-1–Fe3+–L-Cys complex, at which point fluorescence gradually returned. Therefore, the fluorescence test of this probe showed that the lowest detection limit of iron ions was 1.67 × 10−7 mol L−1, and its complexation mode was in the form of 1 + 1. The novel probe formed by R-β-D-1–Fe3+ can be used for the fluorescence detection of cysteine.
Introduction
Vesicles are self-assembled nanoparticles that, due to their unique hollow structure, can be used as drug carriers in medical therapies, transporting drug molecules to reach targeted cells and protecting surrounding healthy tissues.1,2 In this respect, vesicles are similar in structure to cell membranes and can be used to mimic biological membranes, which has attracted the interest of many researchers.3 The vesicle membrane can be used for molecular recognition through metal ion coordination, hydrogen bonding and other non-covalent bonding effects. In addition, metal coordination-induced vesicle aggregation can help to better understand the role of metal ions in cell membrane movement, as phospholipids in cell membranes are able to form complexes with some specific metal ions, such as Ca2+.4,5 Selective metal ions can trigger vesicle aggregation through rational building block design. Thus, vesicle aggregation systems may be a good platform for metal ion sensors, which are less often mentioned.
Due to the importance of transition metal ions in biology and the environment, the design and synthesis of fluorescent sensors that can effectively recognize transition metal ions has attracted great interest.6–10 The pollution by metal ions is increasing today with the rapid development of modern industry, especially industrial effluents discharged into rivers and lakes, etc. The pollution caused by transition metal ions pollution is the most serious, of which Fe3+ pollution is the most common. If the Fe3+ content in fresh water (especially drinking water) is insufficient or excessive, it will cause a series of problems. For example, too much Fe3+ in human body will cause iron poisoning and increase the chance of intestinal cancer; too little lead to cause anemia, low immunity and other adverse reactions.11–15 Excess Fe3+ in rivers may lead to the death or overpopulation of aquatic organisms, so rapid detection of the presence of Fe3+ is essential.16 Amino acids have important roles in living organisms, for example, methionine can participate in detoxification reactions in the liver, which can promote the discharge of toxic substances, with certain antioxidant effects; arginine can promote the synthesis of urea in the human body, thus reducing the concentration of blood ammonia, so it is very important to detect arginine, and it is very convenient and fast to detect it by the method of fluorescence sensor17–19 and cysteine also plays a very important role in the human body such as skin damage, hair discoloration, lethargy and other issues. There are many ways for organisms to ingest cysteine; animals mainly ingest cysteine through feed, while humans mainly ingest cysteine through milk and food.20–26 Therefore, the detection of cysteine is very important, and the fluorescent probe assay has a great advantage with the advantages of high sensitivity and simplicity.27,28 According to research reports, triazole can be used as a corrosion inhibitor for organic copper29,30 and is a very important metal complex.31,32 1,2,3-Triazole has better affinity and easier coordination with metal ions.33,34
In this paper, a 2-substituted 1,2,3-triazole-glucose derivative based on octahydronaphthol was synthesized by introducing glucose molecules via click reaction. It can be observed by scanning electron microscopy (SEM) that the new fluorescent probe self-assembles in ethanol solution into a vesicular structure suitable for the recognition of Fe3+, which has not been reported in the literature. The fluorescence properties of the derivative were investigated and it was found to selectively recognize Fe3+ and to provide secondary recognition of cysteine. The secondary recognition of the sensor R-β-D-1 with Fe3+ and cysteine was probed using SEM and TEM.
Experimental
Reagents and instruments
To remove impurities, all solvents were redistilled before use. The analytically pure chemicals used were supplied by pharmaceutical companies or synthesized by routes known in our laboratory. The corresponding metal nitrates or chlorides were used to configure 0.1 M metal ions in methanol solutions. FeCl3 was used as the source of Fe3+ unless otherwise noted. TMS was used as the internal standard and chloroform-D or dimethylsulfoxide-D6 was used as the solvent. 13C NMR and 1H NMR were measured by a Bruker AM-400WB spectrometer. Fluorescence emission spectra were measured by Hitachi F-7100 fluorescence spectrometer unless otherwise stated. Electrospray mass spectra were detected by a Bruker Amazon SL ion trap MS.
R-2,2′-Bis(prop-2-yn-1-yloxy)-5,5′,6,6′,7,7′,8,8′-octahydro-1,1′-binaphthalene (0.20 g, 0.54 mmol) and 1-azido-2,3,4-triacetoxy-5-acetoxymethyl-D-glucose (0.44 g, 1.19 mmol). Under argon protection, 5 mL of tetrahydrofuran was added to the system. The ice bath was stirred for a few minutes to dissolve it completely, next, sodium ascorbate (0.24 g, 1.18 mmol) and anhydrous copper sulfate (0.13 g, 0.54 mmol) were accurately weighed and dissolved in 4 mL of deionized water, shaken well, and when the mixed solution turned to an earthy yellow color, it was added to the reaction system. The system was reacted for 10 h at room temperature and TLC was performed (PE
:
EA = 4
:
1). The reaction was quenched by adding 10 mL of ice water to the reaction system and the aqueous phase was extracted three times with 30 mL of dichloromethane. The organic phases were then combined, washed once with saturated NaCl solution and dried with anhydrous magnesium sulfate for 30 min. After filtration and rotary evaporation of the solvent, it was separated by column chromatography (PE
:
EA = 1
:
1). About 0.55 g of white solid was obtained in 91.2% yield. 1H NMR (400 MHz, DMSO-d6) δ 8.03 (s, 1H), 6.95 (s, 2H), 6.31 (d, J = 8.6 Hz, 1H), 5.59–5.46 (m, 2H), 5.15 (t, J = 9.5 Hz, 1H), 5.05–4.92 (m, 2H), 4.19–3.97 (m, 3H), 2.04–1.96 (m, 8H).·13C NMR (101 MHz, DMSO-d6) δ 170.06, 169.43, 168.42, 152.95, 144.25, 136.23, 129.61, 128.45, 126.11, 123.10, 111.04, 83.90, 73.49, 72.30, 70.34, 67.73, 61.92, 61.57, 40.36, 39.10, 28.88, 26.79, 22.73, 20.55, 20.35, 19.85.
Results and discussion
According to Scheme 1, the relatively easily synthesized R-1 was synthesized from R-H8-BINOL and 3-bromo-1-propyne with moderate yields, respectively. Then the click reactions of R-1 and 1-azido-2,3,4-triacetoxy-5-acetoxymethyl-D-glucose were carried out under the catalytic effect of Cu+ (formed by the reduction of copper sulfate with sodium ascorbate) to obtain the desired target sensors, respectively. The yields were 89%, respectively. The resulting compounds were structurally characterized by 1H NMR, 13C NMR and ESI-MS.
 |
| Scheme 1 Synthesis of chiral fluorescence sensor R-β-D-1. | |
It was found by DLS that it could self-assemble into vesicles with a tendency to polymerize, and its particle size was about 1–2 μm (Fig. 1). The ability to self-assemble into vesicle structure may be due to the hydrophilicity of the glucose moiety and the hydrophobicity of the benzene ring and triazole moiety.
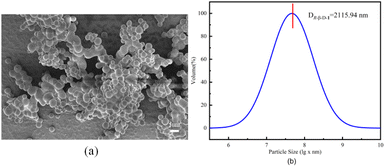 |
| Fig. 1 (a) SEM image of probe R-β-D-1, (b) DLS assay of probe R-β-D-1 (5 mg mL−1 in ethanol). | |
Fluorescence study
The fluorescence spectral characteristics of the R-β-D-1 fluorescent chemosensor were investigated using a Hitachi F-7100 fluorescence spectrometer. As indicated in Fig. S8,† R-β-D-1 emitted mid-intensity fluorescence at 331 nm (λex = 278 nm, EX slit = 2.5 nm, EM slit = 2.5 nm). The effect of R-β-D-1 on different cations (Ba2+, Mn2+, Cu+, Ca2+, K+, Co3+, Cr3+, Zn2+, Al3+, Mg2+, Pb2+, Cu2+, Cd2+, Gd3+, Li+, Na+, NH4+, Ni2+, and Fe3+) in methanol solution was investigated by fluorescence spectrophotometry. The fluorescence of the sensor R-β-D-1 showed significant fluorescence quenching in response to Fe3+, whereas it did not show significant fluorescence quenching for other ions under the same conditions. Fig. 2 shows the fluorescence of R-β-D-1 and R-β-D-1 with the addition of 5 eq. Fe3+, fluorescence quenching rate of 65%. This indicates that R-β-D-1 is capable of specific fluorescence recognition of Fe3+.
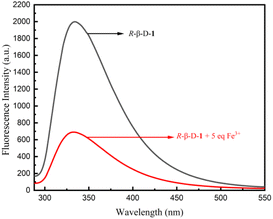 |
| Fig. 2 Fluorescence recognition plot of R-β-D-1 (20 μM, MeOH solution λex = 278 nm, EX slit = 2.5 nm, EM slit = 2.5 nm) and Fe3+, the remaining ions are recognized in the ESI.† | |
Cations competition studies. In order to further investigate the selectivity and specificity of the fluorescence recognition of Fe3+ by R-β-D-1, we carried out ionic immunity experiments using fluorescence spectrometry on R-β-D-1, as shown in Fig. S7.† Other 5.0 equivalents of metal ions and the same equivalents of Fe3+ were mixed in the assay solution, and the fluorescence response was performed at λex = 278 nm and the fluorescence emission intensity was measured. The results of the study showed that the other metal ions had little effect on R-β-D-1–Fe3+. This suggests that R-β-D-1 can specifically selectively fluoresce in response to Fe3+ and can be used as a specific fluorescent probe for detecting background competing ions.The concentration-dependent fluorescence experimental detection of Fe3+ by the sensor R-β-D-1 is shown in Fig. 3. According to the results of the titration experiment, as shown in Fig. 3a, the fluorescence intensity of R-β-D-1 at 331 nm gradually decreased and red-shifted to 351 nm when Fe3+ increased from 0 to 17 eq. The maximum fluorescence intensity showed a good linear relationship with Fe3+ concentration (Fig. 3b).
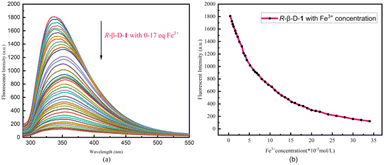 |
| Fig. 3 (a) Fluorescence titration of sensor R-β-D-1 (20 μM in CH3OH, λex = 278 nm, EX slit = 2.5 nm, EM slit = 2.5 nm) with a gradual increase in Fe3+ (0.01 M) equivalents (0–17 eq.). (b) Plot of the change in fluorescence intensity of R-β-D-1 at 331 nm with increasing Fe3+ concentration. | |
To determine the complexation of R-β-D-1 with Fe3+, we investigated the bonding affinity of R-β-D-1 with Fe3+ and then plotted a Job's plot using the reported method. The total concentration of the mixed solution of R-β-D-1 and Fe3+ was kept at 2.0 × 10−5 M (R = 0.991) throughout the experiment. Based on the concentration-dependent fluorescence titration experiment (Fig. 4a), the minimum detection limit (LOD) of the novel sensor R-β-D-1 for Fe3+ was calculated from “LOD = 3σ/S” as 2.84 × 10−7 M. Based on the plots of I0/(I0 − I) and 1/[Fe3+], the complexation constant (Ka) of R-β-D-1 with Fe3+ was calculated to be 0.33 × 104 using the Benesi–Hildebrand equation as shown in Fig. 4b. As shown in the results of Fig. 4c, the molar fraction of [Fe3+] reached a maximum when [Fe3+]/([R-β-D-1] + [Fe3+]) was about 0.5, indicating that the sensor R-β-D-1 formed a 1 + 1 complex with Fe3+. And based on the fluorescence test data of R-β-D-1–Fe3+ and L-Cys, straight lines of I/(I0 − I) and 1/[L-Cys] were also fitted for R-β-D-1–Fe3+ and L-Cys. The binding constants of R-β-D-1–Fe3+ and L-Cys, Ka = 4.18 × 103 M−1, and the binding affinity, Kd = 2.39 × 10−4 M, were calculated as shown in Fig. S10.†
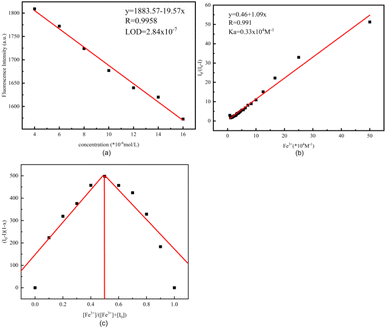 |
| Fig. 4 (a) Linear relationship between fluorescence intensity and Fe3+ concentration for R-β-D-1, calculated for LOD = 2.8 × 10−7 M. (b) 1 : 1 combination based on stoichiometric I0/(I0 − I) and 1/[Fe3+] Benesi–Hildebrand equation plots. (c) Working curves of 1 : 1 complexes of R-β-D-1 with Fe3+, x is the molar fraction of Fe3+. | |
To further demonstrate that the complex R-β-D-1–Fe3+ of the sensor R-β-D-1 with Fe3+ was formed in a 1
:
1 stoichiometric ratio, the sensor R-β-D-1 and the complex R-β-D-1–Fe3+ were probed with ESI-MS, and a significant free at m/z = 1139.4050 was obtained, supplied by the [R-β-D-1 + Na+] R-β-D-1 molecular ion peak (Fig. 5a). Meanwhile, as can be seen from Fig. 6b, the complex R-β-D-1–Fe3+ was trapping an Cl− and a new peak was found at m/z = 1207.3193 (Fig. 5b). This finding supports our hypothesis that the R-β-D-1–Fe3+ complex has a strong binding affinity for Fe3+.
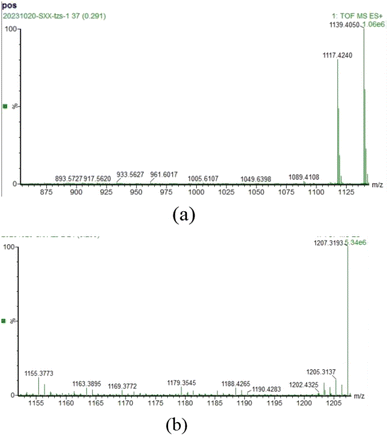 |
| Fig. 5 (a) ESI-MS variation of R-β-D-1; (b) ESI-MS variation of R-β-D-1–Fe3+. | |
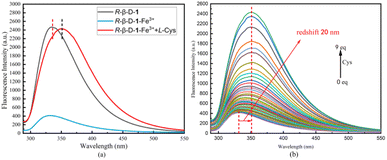 |
| Fig. 6 (a) Is a fluorescence control plot of R-β-D-1, R-β-D-1 + 10 eq. Fe3+, R-β-D-1 + 10 eq. Fe3+ + 10 eq. L-Cys, with the remaining amino acids identified in the ESI.† (b) Fluorescence titration plot of R-β-D-1–Fe3+ (20 μM in MeOH, 10 eq. Fe3+, λex = 278 nm) with gradual increase in Cys (0.01 M) equivalents (0–9 eq.). | |
Fluorescence and absorption titration of amino acids against R-β-D-1–Fe3+. Fluorescence spectroscopy of the sensor R-β-D-1 was performed using a fluorescence spectrometer to analyze the R-β-D-1–Fe3+ complex as a novel fluorescent sensor for 17 natural amino acids. The fluorescence recognition plots of only R-β-D-1–Fe3+ versus L-Cys are presented in Fig. 6a, with the rest of the amino acids recognized in the ESI Fig. S9.† The fluorescence selectivity identification experiments were performed by adding 10.0 eq. of different natural amino acids (Phe, His, Lys, Ala, Arg, Ser, Met, Leu, Gly, Val, Asn, Gln, Thr, Glu, Pro, Asp, and Cys) to R-β-D-1–Fe3+ in MeOH solvent. As shown in Fig. 6a, there was some degree of fluorescence enhancement of R-β-D-1–Fe3+ at 331 nm in the presence of Cys, while there were no other significant changes in the fluorescence emission intensity of the other amino acids on the probe. This demonstrated that R-β-D-1–Fe3+ was able to specifically and selectively identify Cys. Meanwhile, fluorescence titration experiments were carried out with Cys against R-β-D-1–Fe3+ as shown in Fig. 6b. During the fluorescence titration of R-β-D-1–Fe3+, a gradual enhancement of the fluorescence intensity of R-β-D-1–Fe3+ was observed at 331 nm, and the fluorescence intensity reached a maximum with the addition of 9 eq. Cys, with a simultaneous redshift of 20 nm. The titration phenomenon of R-β-D-1 with Cys showed that the fluorescence gradually recovered with the titration of L-Cys, but instead of recovering the original fluorescence of R-β-D-1, it was red-shifted by 20 nm, which indicated that the L-Cys did not only complex with Fe3+, but rather the three substances, namely, R-β-D-1, Fe3+ as well as L-Cys, complexed, which caused the fluorescence of the sensor to be changed.
Electron microscopy studies. AFM also explored the process of recognition of Fe3+ by the sensor R-β-D-1 and secondary recognition of Cys. R-β-D-1 was seen and fluoresced under a microscope when dissolved in ethanol. While fluorescence disappeared from the field of view after the addition of Fe3+, it was restored by the addition of Cys, which was under the same phenomenon as in the fluorescence test (Fig. 7).
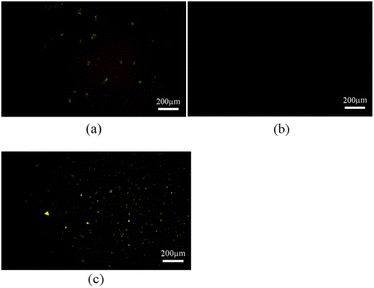 |
| Fig. 7 (a) AFM picture of R-β-D-1 (5 mg mL−1 in ethanol), (b) is the AFM picture after addition of Fe3+ to R-β-D-1, (c) is the AFM picture after addition of L-Cys to R-β-D-1–Fe3+. | |
R-β-D-1 was dissolved in ethanol, configured with Fe3+ as well as amino acid test solutions, and used for SEM and TEM to obtain Fig. 8a–f. Fig. 8a showed that R-β-D-1 was dispersed vesicles with a particle size of approximately 2 μm. Furthermore, the color of the interior of the vesicles in contrast to the surrounding color suggested that the interior of the vesicles was a hollow structure similar to that of the vesicles formed by phospholipids. After the addition of Fe3+, R-β-D-1–Fe3+ vesicles were obtained, and due to the dynamic properties of the vesicle membrane, the added Fe3+ bound to the vesicles, which could be presumed to be due to the vesicles encapsulating the added Fe3+ inside the vesicle cavity according to the TEM (Fig. 8e). And after the addition of cysteine, like Fe3+, L-Cys also bound to the sensor R-β-D-1–Fe3+, forming three compounds of R-β-D-1–Fe3+–L-Cys complexed together (Fig. 8f). This indicates that the vesicle membrane is soft and flexible. This is the recognition of Fe3+ by R-β-D-1 and the secondary recognition of Cys.
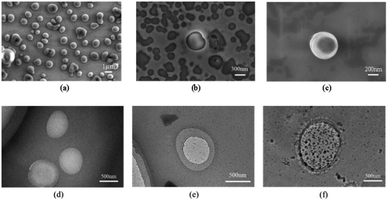 |
| Fig. 8 (a) Is the SEM image of sensor R-β-D-1 (5 mg mL−1 in ethanol), (b) is the SEM image of R-β-D-1 with Fe3+, (c) is the SEM image of L-Cys added to R-β-D-1–Fe3+, (d) is the TEM image of sensor R-β-D-1, (e) is the TEM image of Fe3+ added to sensor R-β-D-1; (f) is the TEM plot of the addition of L-Cys in R-β-D-1–Fe3+. | |
Plausible mechanism for sensing of R-β-D-1. The plausible sensing mechanism of R-β-D-1. The article published by our group in 2022 was a monosubstituted xylose structure introduced by click reaction in BINOL,35 and the fluorescence redshift of its sensor was not obvious after the addition of Fe3+ and blueshifted to the initial fluorescence of the sensor after the addition of L-Cys, whereas in this paper, we introduced a double-substituted glucose on H8-BINOL, and the introduced glycan structures with different spatial resistances were not the same, and a significant redshift occurred after the addition of Fe3+ and no blueshift to the initial fluorescence of the sensor after the addition of L-Cys in the case of R-β-D-1, a significant redshift occurred after the addition of Fe3+, and there was no blue shift to the initial fluorescence after the addition of L-Cys, so the fluorescence phenomenon and mechanism of the two are not the same.According to the structural analysis of the sensor R-β-D-1, the rational mechanism for the recognition of Fe3+ and the secondary recognition of L-Cys is the intramolecular charge transfer effect (ICT) and phototropic electron transfer (PET),36,37 this is due to the fact that the N-3 of 1,4-disubstituted triazole can easily act as an electron acceptor,38–40 and the addition of Fe3+ acts as an electron donor, and the coordination between the two is easy to occur, which leads to the red-shift of fluorescence, and the electron jump from the ground state to the excited state of R-β-D-1 induced by light, and the electron in the excited state can not go back to the ground state after the addition of Fe3+, which leads to the quenching of fluorescence. And after the addition of L-Cys, the HS-group in cysteine is easy to coordinate with metal ions,41 and then combined with fluorescence titration Fig. 6b, fluorescence did not return to the starting fluorescence but red-shifted from 331 nm to 351 nm, in addition to then according to the TEM of Fig. 8f also proved the possibility of the three compounds complexed together. In order to further understand the recognition mechanism of R-β-D-1 with Fe3+, we performed DFT calculations. Density functional calculations of B3LYP/6-31G were carried out using the Gaussian 16W program, and the structure of R-β-D-1 before and after coordination with Fe3+ was calculated by taking into account the dispersion correction of GD3BJ (Fig. 9). From the figure, it can be found that Fe3+ complexes with the 3-N of 1,2,3-triazole, forming Fe3+–N bonds with bond lengths of 2.13 Å and 2.11 Å, which verifies the conjecture of our mechanism.
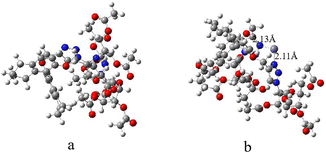 |
| Fig. 9 (a) Shows the structure of the sensor R-β-D-1 and (b) shows the structure of the R-β-D-1–Fe3+ coordination. | |
Conclusions
H8-BINOL–glucose derivatives were synthesized and characterized from azido glucose and R-H8-BINOL. The fluorescence properties of metals were investigated by spectroscopic techniques. The high selectivity and sensitivity of the sensor R-β-D-1 in Fe3+ sensing was confirmed by fluorescence response experiments, competition experiments and fluorescence titration experiments on 19 different metal ions. The stoichiometry and coordination of the complexes were determined by ESI-MS and fluorescence emission spectroscopy, confirming the existence of 1 + 1 mode binding between the sensor R-β-D-1 and Fe3+. The fluorescence response of the R-β-D-1–Fe3+ complex to amino acids was further explored using the R-β-D-1–Fe3+ complex as a novel fluorescent probe, and it was found that R-β-D-1–Fe3+ could perform secondary recognition of Cys. R-β-D-1–Fe3+ produced selective fluorescence enhancement of Cys in 17 natural amino acids through the interactions of the –SH side chain, which enabled R-β-D-1 and R-β-D-1–Fe3+ complex systems more attractive. In addition, SEM and TEM were also used to study the process of R-β-D-1 and Fe3+ and secondary recognition of L-Cys. It was found that after the sensor R-β-D-1 complexed with Fe3+, L-Cys was added to make the sensor vesicle wrap it together. These three compounds, R-β-D-1–Fe3+–L-Cys, were complexed together. The results suggest that R-β-D-1 can be used as a specific fluorescent probe for the detection of Fe3+ and can help to recognize Cys.
Conflicts of interest
There are no conflicts to declare.
Acknowledgements
The authors are grateful for the financial support of the National Natural Science Foundation of China (No. 21462018 and 21861025), the Science Fund of the Technology Office of Jiangxi, China (20192BAB203003) and University-Industry Collaborative Education Program (202102249008).
Notes and references
- L. Wang, G. Wang, W. Mao, Y. Chen, M. M. Rahman, C. Zhu, P. M. Prisinzano, B. Kong, J. Wang, L. P. Lee and Y. Wan, Bioinspired engineering of fusogen and targeting moiety equipped nanovesicles, Nat. Commun., 2023, 14, 3366 CrossRef CAS PubMed.
- J. M. Carnino, Z. Hao Kwok and Y. Jin, Extracellular Vesicles: A Novel Opportunity for Precision Medicine in Respiratory Diseases, Front. Med., 2021, 8, 661679 CrossRef.
- J. R. Sanborn, X. Chen, Y. Yao, J. A. Hammons, R. H. Tunuguntla, Y. Zhang, C. C. Newcomb, J. A. Soltis, J. J. De Yoreo, A. Van Buuren, A. N. Parikh and A. Noy, Carbon Nanotube Porins in Amphiphilic Block Copolymers as Fully Synthetic Mimics of Biological Membranes, Adv. Mater., 2018, 30, 1803355 CrossRef.
- S. K. De, N. Kanwa and A. Chakraborty, Influence of Trivalent Metal Ions on Lipid Vesicles: Gelation and Fusion Phenomena, Langmuir, 2019, 35, 6429–6440 CrossRef CAS PubMed.
- P. Xing, Y. Wang, M. Yang, Y. Zhang, B. Wang and A. Hao, ACS Appl. Mater. Interfaces, 2016, 8, 17676–17684 CrossRef CAS PubMed.
- A. Nayal, P. K. Muwal and P. S. Pandey, Bile acid based receptors for multiple metal ion recognition, Tetrahedron, 2019, 75, 1968–1974 CrossRef CAS.
- M. Shi, C. Fu, J. Yu, Y. Yang and P. Shi, A novel 2D metal–organic framework probe: a highly sensitive and visual fluorescent sensor for Al3+, Cr3+ and Fe3+ ions, New J. Chem., 2022, 46, 18911–18916 RSC.
- D. Wu, L. Chen, W. Lee, G. Ko, J. Yin and J. Yoon, Recent progress in the development of organic dye based near-infrared fluorescence probes for metal ions, Coord. Chem. Rev., 2018, 354, 74–97 CrossRef CAS.
- C. Fu, X. Sun, G. Zhang, P. Shi and P. Cui, Porphyrin-Based Metal–Organic Framework Probe: Highly Selective and Sensitive Fluorescent Turn-On Sensor for M3+ (Al3+, Cr3+, and Fe3+) Ions, Inorg. Chem., 2021, 60, 1116–1123 CrossRef CAS PubMed.
- J.-X. Hou, J.-P. Gao, J. Liu, X. Jing, L.-J. Li and J.-L. Du, Highly selective and sensitive detection of Pb2+ and UO22+ ions based on a carboxyl-functionalized Zn(II)-MOF platform, Dyes Pigm., 2019, 160, 159–164 CrossRef CAS.
- X. Liang, X. Li, Y. Tang, L. Hong, W. Wei and X. Liu, Hyperbranched poly(ester ether)s as an amplified fluorescence sensor for selective and sensitive Fe3+ detection and bioimaging, J. Appl. Polym. Sci., 2022, 139, 51865 CrossRef CAS.
- N. Roy, A. Dutta, P. Mondal, P. C. Paul and T. S. Singh, A new coumarin based dual functional chemosensor for colorimetric detection of Fe3+ and fluorescence turn-on response of Zn2+, Sens. Actuators, B, 2016, 236, 719–731 CrossRef CAS.
- Y. Ma and X. Cheng, Readily soluble cellulose-based fluorescent probes for the detection and removal of Fe3+ ion, Int. J. Biol. Macromol., 2023, 253, 127393 CrossRef CAS PubMed.
- X. Bao, X. Cao, X. Nie, Y. Xu, W. Guo, B. Zhou, L. Zhang, H. Liao and T. Pang, A new selective fluorescent chemical sensor for Fe3+ based on rhodamine B and a 1,4,7,10-tetraoxa-13-azacyclopentadecane conjugate and its imaging in living cells, Sens. Actuators, B, 2015, 208, 54–66 CrossRef CAS.
- Y. Yang, C.-Y. Gao, N. Zhang and D. Dong, Tetraphenylethene functionalized rhodamine chemosensor for Fe3+ and Cu2+ ions in aqueous media, Sens. Actuators, B, 2016, 222, 741–746 CrossRef CAS.
- S. Munusamy and S. Kulathu Iyer, A chiral (S)-BINOL based fluorescent
sensor for the recognition of Fe(III) and cascade discrimination of α-amino acids, Tetrahedron: Asymmetry, 2016, 27, 492–497 CrossRef CAS.
- X. Yu, B. Zhang, C. Fan, Q. Yan, S. Wang, H. Hu, Q. Dong, G. Du, Y. Gao and C. Zeng, Rapid, enantioselective and colorimetric detection of D-arginine, iScience, 2022, 25, 104964 CrossRef CAS PubMed.
- X. Yu, B. Zhang, P. Liao, J. Huang, C. Fan, H. Hu, Q. Dong, G. Du, Y. Gao and C. Zeng, A chemoselective fluorescent probe for arginine in aqueous phase, Dyes Pigm., 2022, 203, 110339 CrossRef CAS.
- P. Liao, X. Yu, C. Fan, B. Zhang, J. Huang, Y. Wu, G. Du, Q. Dong and C. Zeng, Acrylate-guided chemoselective fluorescent detection of arginine and lysine in aqueous media, Dyes Pigm., 2023, 215, 111288 CrossRef CAS.
- C. A. Huerta-Aguilar, B. Ramírez-Guzmán, P. Thangarasu, J. Narayanan and N. Singh, Simultaneous recognition of cysteine and cytosine using thiophene-based organic nanoparticles decorated with Au NPs and bio-imaging of cells, Photochem. Photobiol. Sci., 2019, 18, 1761–1772 CrossRef CAS PubMed.
- C. A. Huerta-Aguilar, P. Thangarasu and J. G. Mora, Tetraphenylethene functionalized rhodamine chemosensor for Fe3+ and Cu2+ ions in aqueous media, J. Mol. Struct., 2018, 1157, 660–671 CrossRef CAS.
- X. Li, K. Fan, W. Kang, R. Yang, B. Qu and L. Lu, γ-Aminobutyric acid-modified graphene oxide as a highly selective and low-toxic fluorescent nanoprobe for relay recognition of copper(II) and cysteine, Microchim. Acta, 2019, 186, 461 CrossRef PubMed.
- A. K. Shoveller, J. G. Pezzali, J. D. House, R. F. Bertolo, P. B. Pencharz and R. O. Ball, Methionine and cysteine oxidation are regulated in a dose dependent manner by dietary Cys intake in neonatal piglets receiving enteral nutrition, PLoS One, 2022, 17, e0275760 CrossRef CAS PubMed.
- X. Han, Z. Zhai, X. Yang, D. Zhang, J. Tang, J. Zhu, X. Zhu and Y. Ye, A FRET-based ratiometric fluorescent probe to detect cysteine metabolism in mitochondria, Org. Biomol. Chem., 2020, 18, 1487–1492 RSC.
- T. Song, W. Qin, Z. Lai, H. Li, D. Li, B. Wang, W. Deng, T. Wang, L. Wang and R. Huang, Dietary cysteine drives body fat loss via FMRFamide signaling in Drosophila and mouse, Cell Res., 2023, 33, 434–447 CrossRef CAS PubMed.
- A. T. S. Wyse, L. D. Bobermin, T. M. Dos Santos and A. Quincozes-Santos, Homocysteine and Gliotoxicity, Neurotoxic. Res., 2021, 39, 966–974 CrossRef CAS PubMed.
- E. Mirsadoughi, F. Nemati, F. Oroojalian and M. Hosseini, Turn –on FRET-based cysteine sensor by sulfur-doped carbon dots and Au nanoparticles decorated WS2 nanosheet, Spectrochim. Acta, Part A, 2022, 272, 120903 CrossRef CAS PubMed.
- R. Jia, K. Jin, J. Zhang, X. Zheng, S. Wang and J. Zhang, Colorimetric and fluorescent detection of glutathione over cysteine and homocysteine with red-emitting N-doped carbon dots, Sens. Actuators, B, 2020, 321, 128506 CrossRef CAS.
- V. S. Bieber, E. Ozcelik, H. J. Cox, C. J. Ottley, J. K. Ratan, M. Karaman, M. Tabakci, S. K. Beaumont and J. P. S. Badyal, Capture and Release Recyclable Dimethylaminomethyl-Calixarene Functional Cloths for Point-of-Use Removal of Highly Toxic Chromium Water Pollutants, ACS Appl. Mater. Interfaces, 2020, 12, 52136–52145 CrossRef CAS PubMed.
- J.-L. Chen, X.-H. Zeng, P. Ganesan, L.-H. He, J.-S. Liao, S.-J. Liu, H.-R. Wen, F. Zhao and Y. Chi, Heterobimetallic Copper(i) Complexes Bearing Both 1,1′-Bis(Diphenylphosphino)Ferrocene and Functionalized 3-(2′-Pyridyl)-1,2,4-Triazole, New J. Chem., 2019, 43, 4261–4271 RSC.
- M. Simmons, K. Cummings Premack, E. D. Guerra, M. J. Bohle, K. A. Rosadiuk and D. S. Bohle, 2,3,5-Metallotriazoles: Amphoteric Mesoionic Chelates from Nitrosoguanidines, Inorg. Chem., 2021, 60, 9621–9630 CrossRef CAS PubMed.
- G. Singh, Diksha, A. Singh, P. Satija, Pawan, Mohit, D. González-Silvera, C. Espinosa-Ruíz and M. A. Esteban, Organosilanes and Their Magnetic Nanoparticles as Naked Eye Red Emissive Sensors for Ag+ Ions and Potent Anti-Oxidants, New J. Chem., 2021, 45, 5517–5525 RSC.
- S. U. Ofoegbu, T. L. P. Galvão, J. R. B. Gomes, J. Tedim, H. I. S. Nogueira, M. G. S. Ferreira and M. L. Zheludkevich, Corrosion inhibition of copper in aqueous chloride solution by 1H-1,2,3-triazole and 1,2,4-triazole and their combinations: electrochemical, Raman and theoretical studies, Phys. Chem. Chem. Phys., 2017, 19, 6113–6129 RSC.
- R. Balasubramanian, M. I. Fathima Rigana, P. Thirukumaran, R. Atchudan, M. Sarojadevi and J. Lee, Synthesis and properties of polytriazoleimide containing anthracene, pyridine and 1,2,3-triazole groups and their nanocomposites with titanium dioxide, Polym. Eng. Sci., 2019, 59, 129–138 CrossRef CAS.
- H. Wang, Y. Liu, Y. Zhang and X. Sun, Synthesis of BINOL–Xylose-Conjugates as “Turn-off” Fluorescent Receptors for Fe3+ and Secondary Recognition of Cysteine by Their Complexes, RSC Adv., 2022, 12, 10379–10385 RSC.
- K. Xiong, F. Huo, J. Chao, Y. Zhang and C. Yin, Colorimetric and NIR Fluorescence Probe with Multiple Binding Sites for Distinguishing Detection of Cys/Hcy and GSH In Vivo, Anal. Chem., 2019, 91, 1472–1478 CrossRef CAS PubMed.
- B. Karasulu and W. Thiel, Photoinduced Intramolecular Charge Transfer in an Electronically Modified Flavin Derivative: Roseoflavin, J. Phys. Chem. B, 2015, 119, 928–943 CrossRef CAS PubMed.
- V. K. Tiwari, B. B. Mishra, K. B. Mishra, N. Mishra, A. S. Singh and X. Chen, Cu-Catalyzed Click Reaction in Carbohydrate Chemistry, Chem. Rev., 2016, 116, 3086–3240 CrossRef CAS PubMed.
- D. S. Pedersen and A. Abell, 1,2,3-Triazoles in Peptidomimetic Chemistry, Eur. J. Org Chem., 2011, 2399–2411 CrossRef CAS.
- A. K. Agrahari, P. Bose, M. K. Jaiswal, S. Rajkhowa, A. S. Singh, S. Hotha, N. Mishra and V. K. Tiwari, Cu(I)-Catalyzed Click Chemistry in Glycoscience and Their Diverse Applications, Chem. Rev., 2021, 121, 7638–7956 CrossRef CAS PubMed.
- M. Lu, Y. Duan, Y. Song, J. Tan and L. Zhou, Green preparation of versatile nitrogen-doped carbon quantum dots from watermelon juice for cell imaging, detection of Fe3+ ions and cysteine, and optical thermometry, J. Mol. Liq., 2018, 269, 766–774 CrossRef CAS.
|
This journal is © The Royal Society of Chemistry 2024 |
Click here to see how this site uses Cookies. View our privacy policy here.