DOI:
10.1039/D4RA00820K
(Paper)
RSC Adv., 2024,
14, 10199-10208
Nonlinear optical and spectroscopic properties, thermal analysis, and hemolytic capacity evaluation of quinoline-1,3-benzodioxole chalcone†
Received
1st February 2024
, Accepted 21st March 2024
First published on 27th March 2024
Abstract
This article describes the synthesis, characterization (1H NMR, 13C NMR, FT-IR, HRMS and XRD), UV-Vis absorption and fluorescence spectra, theoretical analysis, evaluation of nonlinear optical properties (NLO), thermal analysis and determination of the hemolytic capacity of the compound (E)-N-(4-(3-(benzo[d][1,3]dioxol-5-yl)acryloyl)phenyl)quinoline-3-carboxamide (5). Radiological findings showed that compound 5 crystallized in space group Pca21. Furthermore, theoretical DFT studies performed with the B3LYP and M062X functionals showed good agreement with the experimental results and provided valuable information on the molecular and electronic structure, reactivity, polarizability, and kinematic stability of the compound. Besides, compound 5 did not show any hemolytic effect on human erythrocytes and exhibited strong NLO properties. The TG and DTA thermograms of quinoline–chalcone (5) revealed a multi-step thermal decomposition process with a total mass loss of 83.2%, including water content loss. The DTA curves exhibited endothermic peaks corresponding to decomposition steps, melting point, and thermochemical transition. Additionally, exothermic peaks in the DTA thermograms align with significant mass loss, confirming the compound's melting point and water content, as validated by X-ray diffraction analysis. These results contribute to the advancement of research on compounds with NLO properties and offer a promising avenue for the development of substances potentially applicable to optical devices in the biomedical field.
1. Introduction
Nonlinear optics (NLO) is a field of study that explores the interaction between light and matter when the polarization response is nonlinear with respect to the electric field. The study of NLO characteristics, such as the nonlinear refractive index, nonlinear absorption coefficient, and NLO susceptibility, has become more significant in recent years owing to the development of photonic and optoelectronic devices. These materials are widely used in optical computing, optical switching, optical data storage, optical limiting, up conversion lasers, biomedicine, microscopy, among others.1,2
A NLO material experimentally exhibits a nonlinear optical phenomenon, which corresponds to the conversion of light wavelength, amplification of light and conversion of refractive index as a function of optical intensity. In the search for new materials, the study of first and second polarizability values allows researchers to predict and optimize materials for specific NLO applications. A non-zero first polarizability (α) indicates that the material can be polarized by an electric field, which is a prerequisite for NLO effects to occur; and a non-zero second polarizability (β) value determines the strength and type of NLO effect, reflecting the nonlinear response of the material to the applied electric field.3
An example of an NLO phenomenon is second harmonic generation (SHG), in which two photons of the same frequency are combined to generate a new photon at twice the original frequency. SHG has been widely studied for its unique optical properties, which supply valuable information about the underlying physics and the materials involved in this process. Over the years, researchers have made noteworthy progress in understanding and improving SHG, finding that organic molecules are a promising source of potent SHG materials because their structures can be tuned to achieve the desired NLO properties. In addition, chromophoric organic compounds can delocalize the electronic charge distribution, which leads to high mobility due to the overlap of π orbitals, giving them great NLO properties.1,4
Chalcones are a class of chromophoric organic compounds with several characteristics that make them suitable candidates for strong SHG generation.4–9 These features include extended π-conjugation, non-centrosymmetric structure, enhanced nonlinear optical response, structural flexibility, and nonlinear wave mixing capabilities (see Fig. 1). In general, it has been proved that chalcones are a class of organic compounds that usually exhibit satisfactory NLO properties, with enormous potential in the design of optical devices, comparable and even superior to urea.4,6 On the other hand, it has been understood that second-order molecular nonlinearity in chalcones can be enhanced by large delocalized π-electron systems with strong electron donor and acceptor groups.4,5
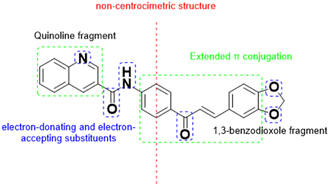 |
| Fig. 1 Typical characteristics that confer and improve NLO properties to chalcones. | |
The basic strategy of including electron donor and acceptor substituents to polarize the π-electron system of organic materials has been employed in the development of NLO chromophores with high first and second order hyperpolarizabilities, good thermal stability, improved solubility and processability.8,10
In addition to what was previously mentioned, chalcones with biologically relevant groups such as quinolines or 1,3-benzodioxol derivatives stand out for their wide variety of biological properties, such as antioxidants, antimicrobials, anticancer, anti-inflammatory, among others.11–17 These properties, together with their intrinsic NLO properties, make chalcones especially interesting for the design of optical materials with biomedical applications. However, it is essential to take into account that, due to the special care required by materials that come into contact with biological tissue, it is important that the candidate compounds do not present toxicological characteristics, including the induction of hemolysis, which is the rupture of the red blood cells in the blood, and can have harmful effects on the body.18
For this reason, in the present work the synthesis and characterization of the chalcone quinoline-1,3-benzodioxole (5) is presented by means of infrared (IR) spectroscopy, proton (1H NMR) and carbon (13C NMR) nuclear magnetic resonance, high resolution mass spectrometry (HRMS) and single crystal X-ray diffraction (XRD). Likewise, the absorption and emission behavior, thermal stability (TG/DTA) and the hemolytic effect on human erythrocytes are studied. Theoretical DFT calculations are used to gain more knowledge regarding the electronic and molecular structure, as well as the NLO properties, given the important potential of 5 in the development of optical devices applicable to the biomedical field. It is important to note that the results presented for compound 5 are derived from theoretical calculations and are compared with other compounds known to exhibit NLO properties, as direct experimental data are not available. This comparison is made to provide a preliminary assessment of its potential NLO behavior.
2. Experimental
2.1. Materials and methods
All chemical reagents and organic solvents were obtained from commercial suppliers and used without further purification. Thin-layer chromatography (TLC) was performed to check the purity of compounds on silica gel 60 HF254 plates (Merck, Germany). The melting point ranges (m.p.) were taken on an Electrothermal IA9100 apparatus (Stone, UK) using the one-end open capillary method and were uncorrected. IR spectra (KBr discs, 500–4000 cm−1) were recorded on a NEXUS 670 FT-IR spectrophotometer (Thermo Nicolet, USA). 1H and 13C NMR spectra were recorded using DMSO-d6 and CDCl3 as solvents and tetramethylsilane (TMS) as an internal reference on an AM-400 spectrometer (Bruker, Germany) at working frequencies 400 and 100 MHz, respectively. High resolution mass spectrometry (HRMS) analyses were conducted using a Bruker “Compact” quadrupole time-of-flight mass spectrometry (qTOF-MS, Germany) coupled with an Apollo II ion funnel electrospray ionization (ESI) source. UV-Vis absorption and fluorescence spectra were recorded in methanol at 10 μM using UV/Visible Metertech SP-8001 and Hitachi F-2710 spectrophotometers, respectively. In both cases, cells with an optical path of 10 mm were used and the fluorescence was acquired with a slit width of 2.5 nm.
2.2. Synthesis N-(4-acetylphenyl)quinoline-3-carboxamide (3)
Quinoline derivative (1) was prepared according to our previously reported experimental procedure (Scheme 1).19 A mixture of commercial quinoline-3-carboxylic acid and freshly distilled thionyl chloride was warmed into reflux for 3 h, then cooled to room temperature and evaporated under vacuum to dryness to quantitatively obtain the corresponding chloride acids (1). This crude material was used without further purification. A mixture of this acyl chloride (1, 1.0 equiv.) and 4-aminoacetophenone (2, 1.0 equiv.) in toluene (10 mL) was stirred at room temperature for 2 h and then treated with a saturated NaHCO3 solution. The biphasic solution was vigorously stirred for 30 min, then decanted, and finally separated. The collected aqueous phase was extracted with EtOAc (2 × 10 mL). The combined organic layer was dried over Na2SO4 and evaporated. The solid was washed with icy water and crude material was crystallized from ethanol. Yield 90%, white solid; m.p. = 255–257 °C; IR (KBr, cm−1): 3327, 3068, 3001, 1985, 1918, 1844, 1676, 1650, 1597, 1268, 1109, 799; 1H NMR (400 MHz, DMSO-d6) δH (ppm): 2.55 (s, 3H), 7.71 (t, J = 8.0 Hz, 1H), 7.89 (t, J = 8.0 Hz, 1H), 7.99 (s, 4H), 8.12 (dd, J = 8.0, 12.3 Hz, 2H), 8.99 (s, 1H), 9.37 (s, 1H), 10.90 (bs, 1H); 13C NMR (100 MHz, DMSO-d6) δC (ppm): 26.4 (CH3), 119.5 (2 × CH), 126.3 (C), 127.3 (C), 127.5 (CH), 128.8 (CH), 129.2 (CH), 129.3 (2 × CH), 131.5 (CH), 132.2 (C), 136.2 (CH), 143.4 (C), 148.5 (C), 149.0 (CH), 164.5 (C), 196.5 (C).
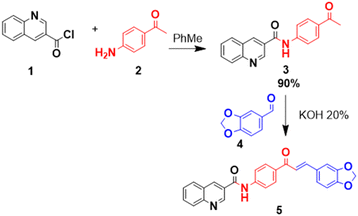 |
| Scheme 1 Synthesis of quinoline-1,3-benzodioxole chalcone 5. | |
2.3. Synthesis and crystallization of (E)-N-(4-(3-(benzo[d][1,3]dioxol-5-yl)acryloyl)phenyl)quinoline-3-carboxamide (5)
The quinolone-1,3-benzodioxole chalcone derivative 5 was prepared according to our previously reported experimental procedure (Scheme 1).20 A solution of equimolar amounts of piperonal (4) (1 mmol) and ketone (3) (1 mmol) was placed in an Erlenmeyer flask together with 20% potassium hydroxide (0.5 mL) and absolute ethanol (10 mL). This mixture was sonicated at room temperature for an appropriate time (10–20 min) until the reaction was completed (the reaction was monitored by TLC). The reaction mixture was then treated with a cold bath and filtered to leave a solid product, which was washed with a hexane, followed by recrystallization from ethanol to obtain the desired compound. Yield 92% (388.6 mg); yellow solid; m.p. = 237–239 °C; IR (KBr, cm−1): 3334, 2917, 1670, 1595, 1528, 1499, 1534, 1245, 1025, 920, 819, 755; 1H NMR (400 MHz, DMSO-d6) δH (ppm): 6.10 (s, 2H), 6.99 (d, J = 7.8 Hz, 1H), 7.33 (d, J = 8.1 Hz, 1H), 7.66 (s, 1H), 7.69–7.72 (m, 2H), 7.83 (s, 1H), 7.88–7.90 (m, 1H), 8.01 (d, J = 8.6 Hz, 2H), 8.13 (dd, J = 8.3, 16.1 Hz, 2H), 8.21 (d, J = 8.6 Hz, 2H), 9.00 (s, 1H), 9.39 (s, 1H), 10.93 (bs, 1H); 13C NMR (100 MHz, DMSO-d6) δC (ppm): 102.0 (CH2), 107.3 (CH), 108.9 (CH), 120.7 (CH), 121.7 (CH), 125.9 (CH), 127.3 (C), 127.4 (CH), 129.1 (2 × CH), 129.5 (2 × CH), 130.0 (2 × CH), 130.9 (CH), 135.9 (CH), 143.0 (CH), 148.5 (C), 148.6 (C), 149.7 (C), 150.8 (C), 165.9 (C), 187.4 (C); HRMS (ESI, m/z): calculated for C26H19N2O4 [M + H]+ 423.1345; found 423.1342.
2.4. X-ray crystal structure determination
Suitable crystals of the chalcone derivative 5 were chosen for the X-ray crystallographic analysis. X-ray intensity data were collected at 296 K in a Bruker D8 Venture diffractometer equipped with a bidimensional CMOS Photon 100 detector, using Mo-Kα radiation of wavelength 0.71073 Å. The diffraction frames were integrated using the APEX3 package and were corrected for absorption effects using the multi-scan method (SADABS). The crystal structure was solved by intrinsic phasing using the OLEX2 program.21 Refinement was conducted by full-matrix least-squares methods based on F2 (SHELXL-2014).22 Figures of the X-ray structure of the title compound were generated with the help of OLEX2 and ORTEP program packages. Crystallographic information files were deposited in the Cambridge Crystallographic Data Centre (CCDC) with the deposition number 2299694.†
2.5. Hemolytic assay
To rapidly assess the cellular toxicity of compound 5, we used a percent hemolysis assay.23 Briefly, non-heparinized blood was collected from a healthy volunteer type O Rh (+). Red blood cells were washed 3 times in PBS (10 mM sodium phosphate buffered saline, 150 mM sodium chloride pH 7.8 ± 0.2), and then diluted with PBS to obtain a 10% suspension. Compound 5 was dissolved in 400 μM DMSO and then PBS was added to prepare 10 serial dilutions starting from 200 μM to 0.39 μM using a microplate, each dilution was mixed 1
:
1 with the red blood cell solution for one volume final 200 μL per well, subsequently mixed gently and incubated at 37 °C with periodic pressure for 60 min of incubation. The solution was then centrifuged at 3000 rpm for 5 min. The absorbance of the supernatant was measured at 540 nm using an Epoch™ microplate photometer (BioTek® Instruments, Inc. Winooski, Vermont 05404-0998 USA). Finally, the hemolytic % was calculated by comparison with 100% of the hemolytic activity caused by Tween 20 as maximum hemolytic control. The hemolytic percentage developed by the PBS control and (1%, w/v) DMSO was used as negative control and blank for the readings in all groups. Each experiment was performed in triplicate at all concentrations used.
The hemolytic % was calculated with the formula:
where ODt is the absorbance of each dilution of the analyzed compound, ODpc is the absorbance of the positive control and ODnc is the absorbance of the negative control.
2.6. Thermal analysis
Thermogravimetry (TG) and differential thermal analysis (DTA) of quinoline–chalcone (5) was registered on a thermogravimetric analyzer TGA-Q500 (TA Instruments, New Castle, DE, USA) using a nitrogen atmosphere from room temperature to 800 °C and a heating rate of 5 °C min−1, with a flow rate of 60 mL min−1. Also, 40 mL min−1 of N2 was used as protection gas into the electronic balance; 5.67 mg of 5 was placed into a Pt crucible for each analysis.
3. Computational methods
3.1. Theoretical calculations
The X-ray diffraction experimental result of the title compound was directly taken for an initial molecular geometry. The geometry optimization of the monomeric structure was conducted using the M062X functional and 6-311++G(d,p) basis set in the gas phase.24,25 M062X is a hybrid meta exchange–correlation functional with double the nonlocal exchange (2X), which is parametrized for nonmetal and long noncovalent range interactions.26 The theoretical UV-Vis electronic spectrum and optimization of the geometry of the first excited state (S1) of the monomeric structure were performed through the time-dependent density-functional theory (TDDFT) calculations at the M062X/6-311++G(d,p) level.27,28 The solvent effect of methanol on the calculated electronic properties of the studied compound was implemented using the polarizable continuum model (PCM).29
Density functional theory (DFT) was employed to optimize the ground-state geometries and compute the vibrational frequencies of compound 5. These calculations were performed with the B3LYP functional and the 6-31G(d) basis set,30–34 since this level of calculation provides a good description of electronic correlations, and exchange interactions. In addition, it is computationally more cost-effective and works quite well to theoretically simulate IR spectra. The calculated vibrational frequencies were scaled by 0.960.35
The frontier molecular orbitals (FMOs), the highest occupied molecular orbital (HOMO) and lowest unoccupied molecular orbital (LUMO), which account for the ability to donate and accept electrons, respectively, were calculated at M062X/6-311++G(d,p) level.36 Some global reactivity descriptors, such as HOMO–LUMO gap, chemical hardness (η), chemical potential (μ), electrophilicity index (ω), and chemical softness (S), which are based on the HOMO and LUMO energies were studied to measure the stability of the synthesized compound. Chemcraft (https://www.chemcraftprog.com) and GaussView 6.0 (ref. 37) programs were used to draw structures and molecular graphs, likewise, all calculations were performed using Gaussian 09 software.37
3.2. NLO properties
The electric dipole moment, linear polarizability, and the first and second hyperpolarizability tensors were evaluated using finite field (FF) approach to explore the non-lineal optical (NLO) properties of the compound 5 with a field frequency of 0.0010 au at the CAM-B3LYP/6-311G++(d,p) level.
DFT functional has shown results close to CCSD and MP2 methodologies,38,39 showing efficiency and accuracy for calculation of second order hyperpolarizability. In the FF method, when a molecule is subjected to a static field (F), the energy (E) of the molecule can be expressed as:
Where
E0 is the energy of the molecule in the absence of an electric field.
μ corresponds to the average dipole moment vector and it is given as:
Afterwards, the static or linear polarizability (α0) and total polarizability Δ(α) tensors can be defined as follows
Following the main equation, the first hyperpolarizability β can be calculated using the following equations:
So, the magnitude of first hyperpolarizability (βtot) can be calculated by following equation.
where
Therefore
βtot = [(βxxx + βxxy + βxyy)2 + (βyyy + βxxz + βyyz)2 + (βxzz + βyzz + βzzz)2]1/2 |
and
β0 was also calculated by the following relation:
Finally, the average second hyperpolarizability tensors γ, will be describe as follows:
All equations have been taken from reported published by Abbas et al.40
4. Results and discussion
4.1. Chemistry and characterization
Fig. S1† and Table 1 show the results of the experimental and simulated infrared (IR) spectra for the molecule 5 using the B3LYP/6-31G(d) method. Table 1 lists the resulting vibration frequencies for the optimized geometries, the proposed vibration assignments, and the IR intensities, in decreasing order. Comparisons of theoretical and experimental IR spectra show that strong vibrations in the experimental spectrum are also strong in the theoretical spectrum.
Table 1 Comparison of calculated and experimental vibration spectra (FTIR) of compound 5
B3LYP/6-31G(d) |
Experimental in this study |
Normal mode |
Freq (cm−1) |
Intensity (km mol−1) |
Freq (cm−1) |
Approximate assignments |
1 |
3468 |
0.03 |
3334 |
N–H stretching |
2 |
3019 |
0.09 |
3008 |
Asymmetric stretching C–H sp2 (phenyl) |
3 |
2924 |
0.37 |
2917 |
Symmetric stretching C–H sp3 |
5 |
1697 and 1671 |
0.39 and 0.21 |
1670 |
Stretching C O ketone and amide |
6 |
1584 |
0.94 |
1595 |
Stretching C C |
8 |
1395 |
0.55 |
1354 |
Rocking in the C–H (sp3) plane |
9 |
1256 |
1 |
1245 |
C–O–C stretch |
On the other hand, Table 1 shows some valuable IR frequencies to find critical functional groups in the molecule 5, which contribute to its characterization. N–H stretching of the amide residue in the molecule is seen in the range of 3334 cm−1, and gaps are also saw at 3008 and 2917 cm−1, which belong to the C–H sp2 and C–H sp3 stretches, respectively. Furthermore, at 1670 cm−1 it can be seen the C
O stretching band that corresponds to the ketone and amide groups superimposed on the same band. Meanwhile, at 1595 and 1354 cm−1 the bands corresponding to the C
C stretching and the C–H rolling in the plane appear. Furthermore, at 1670 cm−1, the C
O stretching band corresponding to ketone and amide groups can be observed, overlapping in the same region. Meanwhile, bands at 1595 and 1354 cm−1 represent the C
C stretching and C–H (sp3) rocking in the plane, respectively. Finally, at 1245 cm−1, the band corresponding to the C–O–C stretching of the 1,3-benzodioxole moiety in the structure is observed, and this is the most intense band in both the theoretical and experimental spectra. As shown in Table 1, the frequencies calculated with the B3LYP/6-31G(d) method showed a good affinity with the results obtained experimentally. Therefore, this computational method is valuable in showing important functional groups for characterizing the molecules studied here.41
4.2. Hemolysis assay
The hemolytic assay was performed with PBS 1× as negative control (pH = 7.0) and Tween 20 as 100% hemolysis causing positive control. Equal amounts of RBCs (red blood cells) suspended in varying concentrations (0.2–100 μM) of ligand displayed <5% hemolysis, which is an acceptable standard (see Scheme 2). The results of this assay are significant as they indicate that chalcone 5 does not have a hemolytic effect on human erythrocytes. Hemolysis is a process that can be detrimental to human health, as it can release hemoglobin into circulation, which, in turn, can lead to the formation of blood clots and other health issues.42 Therefore, the lack of a hemolytic effect of chalcone is an important and promising finding.
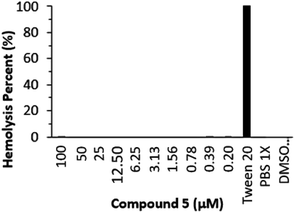 |
| Scheme 2 Hemolytic effect of compound 5 (0.2–100 μM) on human erythrocytes. | |
However, it is important to note that the absence of a hemolytic effect on human erythrocytes is not necessarily indicative of the overall safety of chalcone in all contexts. Other potential effects of chalcone on different cell types or tissues need to be more thoroughly investigated. Additionally, it is crucial to consider that biological safety is only one of many factors to consider when evaluating a substance for use in pharmaceutical or therapeutic applications.
4.3. X-ray single crystal studies
The compound 5 crystallizes in space group Pca21. There are two water molecules and two independent organic molecules in the asymmetric unit, which slightly differs in their molecular conformation, Fig. 2.
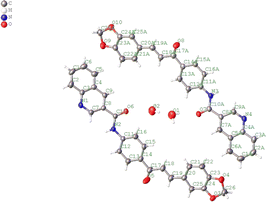 |
| Fig. 2 ORTEP view of the title compound drawn at 30% of probability thermal displacement ellipsoids. | |
Both molecules are just planar, maximum r.m.s deviation 0.095 Å and have an E conformation about the exocyclic C
C bond. The torsion is C8–C10–N2–C11/C8A–C10A–N2A–C11A are 178.7(3) and 177.3 for each molecule, respectively (178(3)° mean).
In the crystal, the molecules are anti-parallel to each other and run down the a-axis and are linked by two kind of hydrogen bond interaction: OW–H⋯O (carbonyl group), H5⋯O2 2.20, C5⋯O2 3.22(5) Å, C–H⋯O 129°; H⋯N 2.474(5) Å, N⋯N 3.297(4) Å, N–H⋯N 162(5)°; H⋯N 2.456(5) Å, C⋯N 3.362(4) Å, C–H⋯N 165(11)°, forming chains and no-centrosymmetric ring with graph-set notation S(8) and R_1^2, respectively (Bersntein), forming a three dimensional network (see Fig. 3). The crystal packing is further connected to another via weak π–π stacking interaction between benzene rings C1/C2/C3/C4/C5/C6; C1A/C2A/C3A/C4A/C5A/C6A with centroid–centroid distances of 3.724 and 3.779 Å, respectively (see Fig. 4). (Cg–Cgi; CgA–CgAii, symmetry code: (i) x − 1 + y, z; (ii) x + 1 + y, z.)
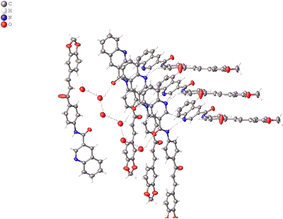 |
| Fig. 3 Hydrogen bond interactions of the title compound. Some hydrogen atoms were omitted for clarity. | |
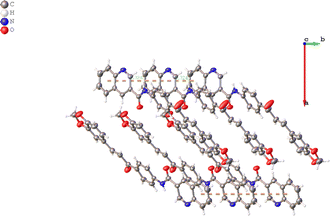 |
| Fig. 4 π–π interactions in compound 5. | |
4.4. Comparison of geometry data
A distances comparative analysis between experimental and theoretical chemical bonds are presented in Fig. 5. The root mean squared (RMS) value predicted by Chemcraft software, which accounts for the difference between the monomer from X-ray diffraction experiment and the theoretically optimized geometry, was low: 0.266 Å. Then, a good agreement was found between the optimized molecular geometrical parameters and experimental ones. As expected, the molecular geometry is practically flat, which favors conjugation and intramolecular electron transfer in the ground state (S0). Likewise, an intramolecular interaction is observed between the C
O group of the amide and the ortho hydrogens of the phenyl moiety, which would favor the coplanarity of the amide-quinoline fragment with the α,β-unsaturated system.
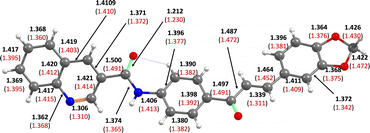 |
| Fig. 5 Chemical bond distances scheme (in Angstrom). The values in brackets correspond to experimental data. | |
4.5. Optical properties
4.5.1. Absorption spectrum. The comparison of the experimental and theoretical UV-Vis spectra of the title compound has been studied. The experimental spectrum scanned in methanol solution within the range of 190–450 nm includes two principal bands localized around 316 and 365 nm (Fig. 6a). Taking into account the cross-conjugation in the amide group, it is inferred that the maximum at 316 nm corresponds to a π → π* electronic transition of the quinoline fragment. Similarly, the maximum at 365 nm is consistent with a π → π* electronic transition of the system α,β-unsaturated (chalcone). In this sense, the bands below 250 nm correspond to n → σ* and π → σ* electronic transitions. This is in agreement with the absorption spectra of quinoline and chalcone phenyl-1,3-benzodioxole moieties reported in the literature.43,44 The theoretical electronic spectrum (see Fig. 6b) matches the experimental UV-Vis spectrum. The transitions to the first 15 singlet excited states were calculated. Table 2 presents the main electronic transitions (except for S0 → S1, transitions with f < 0.2 have been omitted). The S0 → S1 transition at 341.17 nm has a very small oscillator strength (f = 0.0060). Consequently, the S0 → S2 transition at 335.55 nm with a high f = 1.1410 and with a HOMO–LUMO contribution of 77% is correlated with the experimental absorption maximum at 365 nm. The second band located at 288.63 nm with f = 0.7703 arises from an S0 → S3 transition with the main HOMO−1–LUMO contribution of 79%, which is consistent with the experimental band at 316 nm. Additionally, the broad band between 200 and 250 nm in the experimental spectrum would correspond mainly to S0 → S12 (235.22 nm) and S0 → S13 (226.43 nm) transitions. As can also be seen in Fig. 6, the theoretical spectrum only presents values greater than 219 nm. Those values lower than this wavelength could not be simulated in our TDDFT calculations.
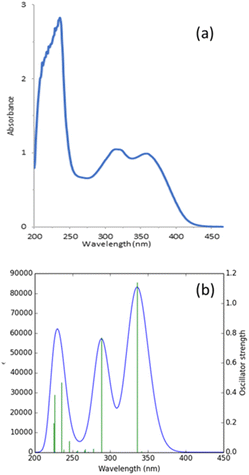 |
| Fig. 6 Experimental (a) and calculated (b) electronic absorption spectra of title compound in methanol. | |
Table 2 Main electronic transitions calculated in MeOH of compound 5
Excited state |
Excitation vertical (nm) |
Ecala (eV) |
fosb |
Major contribution (%) |
Calculated transition energy. Oscillator strength. H: HOMO, L: LUMO. |
S1 |
341.17 |
3.6341 |
0.0060 |
H−6 → L+0 (48%) |
S2 |
335.55 |
3.6949 |
1.1410 |
H+0 → L+0 (77%) |
S3 |
288.63 |
4.2957 |
0.7703 |
H−1 → L+0 (79%) |
S12 |
235.22 |
5.2709 |
0.4695 |
H+0 → L+2 (20%) |
S13 |
226.43 |
5.4757 |
0.3844 |
H−1 → L+2 (12%) |
4.5.2. Fluorescence spectrum. The experimental spectrum of fluorescence in methanol at different excitation wavelengths is presented in Fig. 7. After exciting compound 5 in the three bands of maximum absorption, the same emission spectrum was obtained with a maximum λ at 513 nm, but with different emissive intensities. This indicates that when excited at 360 nm a greater number of molecules emit radiative energy, most likely from the same excited state of lower energy S1. Furthermore, it is observed that compound 5 shows a high Stokes shift of 148 nm (7904.08 cm−1). It should be noted that the protic polar solvent does not generate a complete quenching of the fluorescence due to some intermolecular hydrogen bond interaction with compound 5.
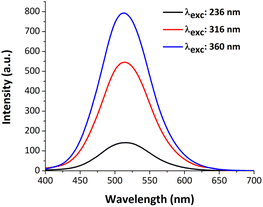 |
| Fig. 7 Fluorescence spectra of compound 5 in methanol at different excitation wavelengths (λexc). | |
To gain more insight into the excited state, the geometry of the first excited state S1 was optimized with the same level of ground state theory, but using the TDDFT formalism. Fig. 8 shows the optimized geometry of state S1, where at first glance no significant change in the geometry is observed with respect to state S0. However, Table 3 presents the geometric parameters that showed changes between each state. It is noteworthy that the changes in bond lengths and angles are mainly in the fragment of the α,β-unsaturated system, indicating that the fluorescent emission occurs from a locally excited state. Additionally, the variation of the C11–C13–C14–C19 dihedral angle indicates that the amide group tends to align with the plane of the α,β-unsaturated system so that the nitrogen donates electron density towards the electron-withdrawing carbonyl group. Beyond what was mentioned, the geometric parameters of compound 5 are not significantly altered after excitation.
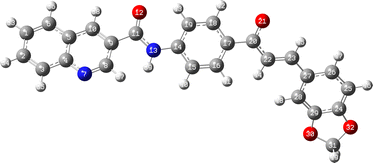 |
| Fig. 8 Optimized geometry of the excited state S1 in the gas phase. | |
Table 3 Representative bond lengths (Å) and angles (°) of the optimized geometries of compound 5 in the S0 and S1 states
Bond |
State S0 |
State S1 |
C20–O21 |
1.215 |
1.290 |
C20–C22 |
1.487 |
1.425 |
C22–C23 |
1.339 |
1.361 |
C23–C27 |
1.464 |
1.455 |
C17–C20 |
1.497 |
1.471 |
![[thin space (1/6-em)]](https://www.rsc.org/images/entities/char_2009.gif) |
Angle |
C20–C22–C23 |
119.50 |
124.21 |
C17–C20–C21 |
120.02 |
116.18 |
C21–C20–C22 |
121.30 |
119.62 |
C18–C17–C20–O21 |
12.87 |
20.69 |
O21–C20–C22–C23 |
6.09 |
5.00 |
C11–C13–C14–C19 |
7.40 |
2.61 |
4.6. Frontier molecular orbitals (FMOs) analysis and molecular reactivity
The frontier molecular orbitals (FMOs), HOMO and LUMO, are the main orbitals responsible for chemical stability or reactivity. The HOMO–LUMO plots for the title compound is shown in Fig. 9. The molecular form of the HOMO shows a high electron density located mainly in the α,β-unsaturated-1,3-benzodioxole fragment, without encompassing the quinoline-amide group. On the other hand, in the LUMO the regions with the highest electronic probability are observed in the quinoline-amide residue together with the α,β-unsaturated ketone. The above indicates that there is a good intramolecular charge transfer (ICT) from the main donor group 1,3-benzodioxole towards the acceptor α,β-unsaturated carbonyl moiety. This good ICT is extremely favorable for the NLO properties of compound 5.
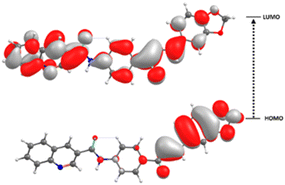 |
| Fig. 9 HOMO and LUMO frontier molecular orbitals of 5. | |
HOMO energy is associated with the electron-donating ability of a compound, while LUMO energy with electron accepting. The FMO energy gap supplies information about chemical reactivity and kinetic stability. A molecule with a small frontier orbital gap is associated with a high chemical reactivity and low kinetic stability. The HOMO and LUMO energies, and frontier orbital energy gap of the title compound were found to be −7.3259, −1.6120, and 5.7138 eV, respectively. The calculated values of the chemical hardness, chemical potential, electrophilicity index, and chemical softness parameters for the compound described above are 2.86, −4.47, 3.50 eV, and 0.18 eV−1, respectively. The 1,3-benzodioxole chalcone derivative is expected to be less polarizable and associated with a low chemical reactivity due to its larger molecular gap and chemical hardness values, saying a hard molecule with low polarizability with high kinetic stability.
4.7. Nonlinear optical (NLO) properties
The dipole moment, polarizability and hyperpolarizability results obtained at CAM-B3LYP/6-311++g(d,p) level of theory is presented in Table 4. Urea as well as two published compounds with NLO properties have been used as prototype molecules for a comparative approach to NLO properties.
Table 4 Dipole moment, polarizability and hyperpolarizability values obtained at CAM-B3LYP/6-311++g(d,p) level of theory
Substance |
μ (D) |
α0 (×10−24 esu) |
Δα (×10−24 esu) |
βtot (×10−30 esu) |
β0 (×10−30 esu) |
γ (×10−36 esu) |
Studied compound 5 |
2.911 |
56.250 |
49.839 |
30.909 |
18.546 |
212.464 |
Urea |
3.875 |
4.823 |
1.964 |
0.768 |
0.461 |
2.941 |
(E)-1-(1,3-Benzodioxol-5-yl)-4,4-dimethylpent-1-en-3-one45 |
3.546 |
27.625 |
21.645 |
21.550 |
12.930 |
44.189 |
(2E)-1-(1,3-Benzodioxol-5-yl)-3-phenylprop-2-en-1-one (SSP1)46 |
3.011 |
31.346 |
31.145 |
5.398 |
3.239 |
67.879 |
The relevance of polarizability and hyperpolarizability of a molecular system depends on electronic communication between two distinct parts of a molecule. The results showed non-zero μ values for the title compound, which should cause microscopic quadratic and cubic hyperpolarizabilities, with resulting non-zero values derived by the second numerical derivatives of electric dipole moments to the field implemented (see Table 4). Although dipole moment for compound 5 is lower than comparative molecules, the linear polarizability tensor α is the highest value reported in Table 4. It quantifies how the electron distribution within the material can be distorted or shifted when subjected to an external electric field. The high first and second hyperpolarizability (β and γ, respectively) values presented by compound 5 are indicative that the material shows strong NLO effects. This is crucial for advanced NLO applications, such as all-optical signal processing, optical switching, and the development of highly efficient NLO devices.
4.8. Thermal analysis studied
The Fig. 10 shows both TG and DTA thermograms of quinoline–chalcone 5, recorded from room temperature to 800 °C, the thermal decomposition processes occurred as several consecutive and simultaneous steps of mass loss, mainly four steps with a total mass loss of 83.2% considering a loss water content of 4.2 wt%. The four steps of mass loss were observed in the temperature range from 68.7 to 656.7 °C and mass loss values of 12.37, 8.8, 49.8 and 8.0%, respectively. The DTA curves reveals a succession of endothermic peaks located at 57.32, 183.40 and 239.62 °C, these endothermic peaks match with the decomposition I and II steps of TG curve and which are attributable to thermochemical transition and melting point, respectively.
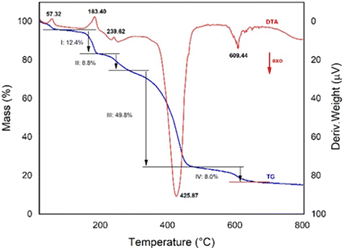 |
| Fig. 10 TG-DTA thermograms of quinoline–chalcone 5. | |
Furthermore, in the region of 317 to 672 °C of DTA thermograms are observed two exothermic peaks at 425.87 and 609.44 °C, which are found inside the greatest mass loss of 49.8% (step III) with the maximum degradation temperature (Tm/°C) of 425.87 °C, accompanied by a minor mass loss of 8.0% (step IV) with a Tm/°C of 609.44 °C.
In summary, the TG/DTA analysis corroborated the melting point (Fusiometer measurement m.p.: 237–239 °C; m.p. from DTA curve: 239.62 °C) of the chalcone 5, thus as confirmed the presence of 4.2 wt% water content that contrasts with the molecular formula (C26H19N2O4·H2O) confirmed by diffraction X-rays analysis.
5. Conclusions
In this study, the quinoline-1,3-benzodioxole chalcone 5 was synthesized and characterized. Crystallographic studies showed that compound 5 crystallized in the Pca21 space group, with two water molecules and two independent organic molecules in the asymmetric unit. Both molecules are planar, with a maximum rms deviation of 0.095 Å, and have an E conformation concerning the C
C exocyclic bond.
Theoretical studies showed good agreement with experimental data from infrared (IR) and ultraviolet-visible (UV-Vis) spectroscopy. Experimentally, chalcone 5 showed three absorption maxima in the ultraviolet region, while the fluorescent emission originated from a locally excited state, since DFT calculations did not yield significant changes in the geometry of the S0 and S1 states. Furthermore, based on the chemical descriptors obtained by DFT analysis, the chalcone 5 is expected to be a hard molecule with low polarizability and high kinetic stability.
The TG and DTA thermograms of compound 5 revealed a multi-step thermal decomposition process with a total mass loss of 83.2%, including the loss of water content. The DTA curves exhibit endothermic peaks corresponding to decomposition steps, melting point, and thermochemical transition. Additionally, exothermic peaks in the DTA thermograms align with significant mass loss, confirming the melting point and water content of the compound, as validated by X-ray diffraction analysis.
Hemolysis assay showed that chalcone 5 had no effect on human erythrocytes. The absence of hemolysis, together with the strong NLO properties of the compound, make it a promising substance for the design of highly efficient optical devices with potential applications in biomedicine.
Although our work provides great insight into the synthesis, characterization, and potential applications of chalcone 5, there are still many avenues for future research. First, given the theoretical promise of the nonlinear optical (NLO) properties of chalcone 5, further experiments are required to determine its potential in this context. Additionally, investigating structural modification of chalcone 5 to improve NLO activity or tailor its properties to specific applications could be a fruitful area of research. Additionally, since the compound lacks hemolytic activity and potential biomedical applications, future studies may further examine its biological activity and therapeutic potential, including in vitro and in vivo studies to evaluate its efficacy and safety.
Conflicts of interest
There are no conflicts to declare.
Acknowledgements
The authors acknowledge the Research Group of the Laboratory of Organic Synthesis and Biological Activity of the University of Talca. Fondecyt Projects 1200531, and 3220681. The authors also acknowledge to FONDEQUIP program (EQM 130021, 160063 and 180024). This work was supported by the Universidad de Ibagué Research Fund, Ibague, Colombia, project No. 20-002-INT.
References
- N. Sudha, R. Surendran and S. Jeyaram, Opt. Mater., 2022, 131, 112668 CrossRef CAS.
- N. V. Agrinskaya, V. A. Lukoshkin, V. V. Kudryavtsev, G. I. Nosova, N. A. Solovskaya and A. V. Yakimanskiǐ, Phys. Solid State, 1999, 41, 1914–1917 CrossRef CAS.
- R. Bano, M. Asghar, K. Ayub, T. Mahmood, J. Iqbal, S. Tabassum, R. Zakaria and M. A. Gilani, Front. Mater., 2021, 8, 783239 CrossRef.
- S. Omar, M. Shkir, M. Ajmal Khan, Z. Ahmad and S. AlFaify, Optik, 2020, 204, 164172 CrossRef CAS.
- H. Belahlou, S. Abed, M. Bouchouit, S. Taboukhat, L. Messaadia, E. E. Bendeif, A. Bouraiou, B. Sahraoui and K. Bouchouit, J. Mol. Struct., 2023, 1294, 136488 CrossRef CAS.
- A. Saha, V. Shukla, S. Choudhury and J. Jayabalan, Chem. Phys. Lett., 2016, 653, 184–189 CrossRef CAS.
- L. R. Almeida, M. M. Anjos, G. C. Ribeiro, C. Valverde, D. F. S. Machado, G. R. Oliveira, H. B. Napolitano and H. C. B. De Oliveira, New J. Chem., 2017, 41, 1744–1754 RSC.
- B. Ganapayya, A. Jayarama and S. M. Dharmaprakash, Mol. Cryst. Liq. Cryst., 2013, 571, 87–98 CrossRef CAS.
- P. J. Tejkiran, M. S. Brahma Teja, P. Sai Siva Kumar, P. Sankar, R. Philip, S. Naveen, N. K. Lokanath and G. Nageswara Rao, J. Photochem. Photobiol., A, 2016, 324, 33–39 CrossRef CAS.
- E. F. Blanco-Acuña, L. A. Vázquez-López, L. Gasque and H. García-Ortega, J. Mol. Struct., 2023, 1271, 134009 CrossRef.
- V. venkatarao, L. Kumar, A. Jha and G. Sridhar, Chem. Data Collect., 2019, 22, 100236 CrossRef CAS.
- R. Ustabaş, N. Süleymanoğlu, N. Özdemir, N. Kahriman, E. Bektaş and Y. Ünver, Lett. Org. Chem., 2018, 17, 46–53 CrossRef.
- C. Tratrat, M. Haroun, I. Xenikakis, K. Liaras, E. Tsolaki, P. Eleftheriou, A. Petrou, B. Aldhubiab, M. Attimarad, K. N. Venugopala, S. Harsha, H. S. Elsewedy, A. Geronikaki and M. Soković, Curr. Top. Med. Chem., 2019, 19, 356–375 CrossRef CAS PubMed.
- L. V. Chinh, T. N. Hung, N. T. Nga, T. T. N. Hang, T. T. N. Mai and V. A. Tarasevich, Russ. J. Org. Chem., 2014, 50, 1767–1774 CrossRef CAS.
- M. Sökmen and M. Akram Khan, Inflammopharmacology, 2016, 24, 81–86 CrossRef PubMed.
- P. Yadav and K. Shah, Bioorg. Chem., 2021, 109, 104639 CrossRef CAS PubMed.
- Z. Muhammad, J. Yau, A. U. Zezi, M. G. Magaji, A. N. Hamza, Z. Muhammad, J. Yau, A. U. Zezi, M. G. Magaji and A. N. Hamza, Am. J. Pharmacother. Pharm. Sci., 2023, 2, 11 Search PubMed.
- H. O. Saxena, U. Faridi, J. K. Kumar, S. Luqman, M. P. Darokar, K. Shanker, C. S. Chanotiya, M. M. Gupta and A. S. Negi, Steroids, 2007, 72, 892–900 CrossRef CAS PubMed.
- E. Polo, N. Ibarra-Arellano, L. Prent-Peñaloza, A. Morales-Bayuelo, J. Henao, A. Galdámez and M. Gutiérrez, Bioorg. Chem., 2019, 90, 103034 CrossRef CAS PubMed.
- E. Polo, J. Trilleras and M. G. Cabrera, Molbank, 2017, 2017, M960 CrossRef.
- O. V. Dolomanov, L. J. Bourhis, R. J. Gildea, J. A. K. Howard and H. Puschmann, J. Appl. Crystallogr., 2009, 42, 339–341 CrossRef CAS.
- G. M. Sheldrick, Acta Crystallogr., Sect. C: Struct. Chem., 2015, 71, 3–8 Search PubMed.
- Z. Chen, H. Duan, X. Tong, P. Hsu, L. Han, S. L. Morris-Natschke, S. Yang, W. Liu and K. H. Lee, J. Nat. Prod., 2018, 81, 465–474 CrossRef CAS PubMed.
- A. D. McLean and G. S. Chandler, J. Chem. Phys., 1980, 72, 5639–5648 CrossRef CAS.
- R. Krishnan, J. S. Binkley, R. Seeger and J. A. Pople, J. Chem. Phys., 1980, 72, 650–654 CrossRef CAS.
- Y. Zhao and D. G. Truhlar, Theor. Chem. Acc., 2008, 120, 215–241 Search PubMed.
- S. Miertuš, E. Scrocco and J. Tomasi, Chem. Phys., 1981, 55, 117–129 CrossRef.
- M. E. Casida, C. Jamorski, K. C. Casida and D. R. Salahub, J. Chem. Phys., 1998, 108, 4439–4449 CrossRef CAS.
- J. L. Pascual-ahuir, E. Silla and I. Tuñon, J. Comput. Chem., 1994, 15, 1127–1138 CrossRef CAS.
- A. D. Becke, J. Chem. Phys., 1993, 98, 5648–5652 CrossRef CAS.
- C. Lee, W. Yang and R. G. Parr, Phys. Rev. B: Condens. Matter Mater. Phys., 1988, 37, 785–789 CrossRef CAS PubMed.
- W. J. Hehre, K. Ditchfield and J. A. Pople, J. Chem. Phys., 1972, 56, 2257–2261 CrossRef CAS.
- P. Geerlings, F. De Proft and W. Langenaeker, Chem. Rev., 2003, 103, 1793–1873 CrossRef CAS PubMed.
- P. J. Stephens, F. J. Devlin, C. F. Chabalowski and M. J. Frisch, J. Phys. Chem., 1994, 98, 11623–11627 CrossRef CAS.
- CCCBDB listing of precalculated vibrational scaling factors, https://cccbdb.nist.gov/vibscalejustx.asp, accessed 25 January 2024 Search PubMed.
- H. Chermette, J. Comput. Chem., 1999, 20, 129–154 CrossRef CAS.
- R. Dennington, T. A. Keith and J. M. Millam, GaussView, Version 6.0, Semichem Inc., Shawnee Mission, KS, 2016 Search PubMed.
- H.-B. Zhao, Y.-Q. Qiu, C.-G. Liu, S.-L. Sun, Y. Liu and R.-S. Wang, J. Organomet. Chem., 2010, 695, 2251–2257 CrossRef CAS.
- Y. Y. Hu, S. L. Sun, S. Muhammad, H. L. Xu and Z. M. Su, J. Phys. Chem. C, 2010, 114, 19792–19798 CrossRef CAS.
- H. Abbas, M. Shkir and S. AlFaify, Arabian J. Chem., 2019, 12, 2336–2346 CrossRef CAS.
- E. Polo-Cuadrado, C. Rojas-Peña, K. Acosta-Quiroga, L. Camargo-Ayala, I. Brito, J. Cisterna, F. Moncada, J. Trilleras, Y. A. Rodríguez-Núñez and M. Gutierrez, RSC Adv., 2022, 12, 33032–33048 RSC.
- M. Frimat, I. Boudhabhay and L. T. Roumenina, Toxins, 2019, 11, 660 CrossRef CAS PubMed.
- E. Mathew and I. H. Joe, J. Mol. Liq., 2023, 392, 123415 CrossRef CAS.
- D. C. Harrowven, B. J. Sutton and S. Coulton, Tetrahedron Lett., 2001, 42, 2907–2910 CrossRef CAS.
- R. I. Al-Wabli, K. S. Resmi, Y. Sheena Mary, C. Yohannan Panicker, M. A. Attia, A. A. El-Emam and C. Van Alsenoy, J. Mol. Struct., 2016, 1123, 375–383 CrossRef CAS.
- S. Shettigar, P. Poornesh, G. Umesh, B. K. Sarojini, B. Narayana and K. Prakash Kamath, Opt. Laser Technol., 2010, 42, 1162–1166 CrossRef CAS.
|
This journal is © The Royal Society of Chemistry 2024 |
Click here to see how this site uses Cookies. View our privacy policy here.