DOI:
10.1039/D4RA02032D
(Paper)
RSC Adv., 2024,
14, 17583-17593
Process optimization and mechanism for the selective extraction of copper(II) from polymetallic acidic solutions using a polymer inclusion membrane (PIM) with Mextral®5640H as the carrier
Received
17th March 2024
, Accepted 27th May 2024
First published on 31st May 2024
Abstract
This study introduces a novel approach by integrating solvent extraction and polymer inclusion membrane (PIM) separation technologies to engineer an innovative PIM membrane material for the selective separation and enrichment of strategic metals, particularly copper, from polymetallic acidic solutions. The primary objectives were to streamline the technological process, reduce production costs, and enhance separation coefficients between copper and other metals. The optimal extraction conditions were determined as a mass fraction of Mextral®5640H
:
PVC
:
NPOE = 3
:
3
:
4, extraction temperature of 35 °C, and 0.9 mol L−1 H2SO4 in the stripping solution. Under these conditions, we achieved remarkable extraction efficiencies, with copper reaching 100%, and the separation coefficients between Cu2+ and Ni2+, Co2+, and Zn2+ exceeding 106. To elucidate the substantial differences in extraction performance between Cu2+ and the other metals (Ni2+, Co2+, and Zn2+), we employed an integrative analytical approach that combines FT-IR spectroscopy, BET analysis, and theoretical calculations. All extracted complexes demonstrated molecular sizes compatible with PIMs, underscoring the critical role of stability and back-extraction performance in the selective extraction of these metal ions.
1. Introduction
Copper, nickel, cobalt, and zinc, along with their compounds, play crucial roles in various aspects of the national economy, daily life, defense industry, and scientific and technological advancements, serving as key strategic resources for enhancing national strength and security.1 In recent years, China has made significant progress in developing and utilizing these resources. The global demand for copper, nickel, cobalt, and zinc has steadily risen, with China consistently ranking first in consumption for fourteen consecutive years. In 2014 alone, the consumption reached 11.09 million tons, 910
000 tons, 37
000 tons, and 6.35 million tons, respectively, representing 41%, 48.9%, 50.3%, and 46% of the world's total.2
Currently, the majority of non-ferrous metal resources in China consist of low-grade oxide minerals and polymetallic ores. With the depletion of high-grade traditional mineral resources the mineral grade is declining, resulting in increased mining challenges. Consequently, the self-sufficiency rate of strategic metals like copper, nickel, cobalt, and zinc has gradually decreased, leading to a growing dependence on external resources and highlighting a supply-demand imbalance.3,4 Therefore, it is imperative to develop sustainable methods for the extraction of precious metals from difficult-to-process mineral resources, such as symbiotic ore, associated ore, poor ore, tailings, and slag ore.5
As the quality of copper, nickel, cobalt, and zinc resources in China decreases over time, it becomes challenging and costly to use traditional methods to efficiently extract these valuable metals from the leaching solutions. Consequently, the leachates and raffinates obtained from processes like leaching, electroplating, and electric industries often contain a mix of target metal ions and other impurities. This makes it crucial to directly separate and concentrate target ions in hydrometallurgical raffinates.6
Due to the intricate nature of hydrometallurgical raffinates, there is an urgent need for a method that can selectively separate and recover valuable metal ions from various input solutions simultaneously. In addition, this approach should offer benefits such as improved separation efficiency, a shorter technological process, and significant reductions in production costs and environmental pollution.7
In recent decades, several methods have been used to extract and recover valuable metals from polymetallic solutions. These include chemical precipitation,8 resin adsorption,9 ion exchange,10 and solvent extraction.11–13 Among these, solvent extraction is widely applied to selectively recover and separate valuable metals from complex mixtures. For example, Cheng and his colleagues14–19 focused on the separation of precious metals using a combination of two extractants, Versatic10 and Lix63. They successfully extracted Cu2+, Co2+, Ni2+ and Zn2+ into the organic phase, leaving impurities in the aqueous phase.20
While solvent extraction has been widely used for decades to separate Cu2+, Co2+, and Ni2+ from Ca2+, Mg2+, Mn2+, and Fe3+, there are still some drawbacks. For instance, Cheng15,21,22 and Zhu23 achieved high separation coefficients for Ni/Co, Ni/Mn, and Co/Mn of 675, 576, and 3065, respectively, using a synergistic combination of Lix63 and Versatic10. However, the nickel stripping rate was only 32% within 5 minutes. Li24 also discovered that the HDNNS/pyridinecarboxylate synergistic system can selectively extract copper, nickel, and cobalt from the acidic polymetallic solutions containing impurities like Ca2+, Mg2+, Mn2+, Cr2+, Fe3+, Al3+, etc. Although improving the structure of the pyridinecarboxylate carbon chain and purifying the commercial extractant HDNNS enhanced the phase separation in the HDNNS/pyridinecarboxylate co-extraction system, increasing the extraction clarification rate from 0.4 m3 m−2 h−1 to 1.0 m3 m−2 h−1, it still falls short of meeting the industrial design demands of 2.4 m3 m−2 h−1 for mixed clarification equipment.
Although solvent extraction has been successful in recovering essential non-ferrous metals, it faces challenges such as slow extraction, difficult phase separation, and low efficiency. Additionally, the need for sequential extraction and stripping steps, along with the use of large amounts of potentially toxic and flammable organic solvents and extractants, raises environmental and safety concerns.25 As alternatives, emulsion liquid membrane, bulk liquid membrane, supported liquid membrane (SLM), hollow fiber renewal liquid membrane and other separation technologies have been applied in hydrometallurgy for Cu2+, Co2+, Ni2+ and Zn2+ recovery and separation.26–28 However, a drawback is the loss of membrane solvent and/or carrier into the aqueous phase during prolonged processes, leading to reduced lifespan of the membranes and hindering the industrial application of membrane separation technologies.
Fortunately, polymer inclusion membranes (PIMs) offer a solution to the problem of losing membrane solvent or carrier during long processes. They come with several advantages, including high selectivity, easy preparation, and high extraction efficiency.29–31 In the PIMs extraction, specific metal ions move through a reversible coordination–dissociation reaction between metal ions and extractants immobilized in the base polymer.32 Studies have shown that PIMs exhibit good durability, long-term stability, and higher selectivity compared to SLMs.33 The efficiency and selectivity of PIMs rely on the carrier used, making the choice of an appropriate carrier crucial for recovering and separating specific metal ions.34 Recent research has identified Aliquat 336, Acorga M5640, LIX84-I and 2-aminomethylpyridineas effective extractants for removing and recovering of strategic non-ferrous metal ions.35–38 However, understanding the intricate relationship between the microstructure and separation mechanism of PIMs remains a complex and active area of research. This complexity depends on factors such as the size of substances to be separated, the pore size of PIMs, operating conditions, and the membrane's specific material and design.35,38
In this study, we aim to combine solvent extraction and PIM separation technologies to create a novel PIM material designed for selectively separating and concentrating copper(II) from polymetallic acidic solutions. Our goal is to streamline the technological process, cut production costs, and enhance the separation coefficients between copper(II) and other metals. We assessed the efficiency of selectively extracting copper(II) under different H2SO4 concentrations in stripping solutions, extraction temperatures, and membrane components in the presence of Co2+, Ni2+, and Zn2+. To understand the mechanism of selective separation and enrichment of copper(II) ions, we employed techniques such as Brunauer–Emmett–Teller (BET) and density functional theory (DFT) calculations.
2. Experimental
To optimize the PIM separation process, we studied the effects of H2SO4 concentration in the stripping solution, extraction temperature, and the components of the membrane on the efficiency of copper(II) extraction. Simultaneously, Brunauer–Emmett–Teller (BET) measurements and density functional theory (DFT) calculations were employed into the investigation of the mechanism of selective separation and enrichment of copper(II) ions.
2.1 Reagents and materials
2-Nitrophenyl-n-octyl ether (NPOE, Fig. 1a) and poly(vinyl) chloride (PVC) were purchased from Shanghai Aladdin Co., Ltd as utilized without additional purification. NPOE served as the plasticizer in this work to maintain the softness of the PIM membranes and enhance their mechanical strength, while PVC was used as the base material for the membranes. Mextral®5640H (Fig. 1b) functioned as an extractant/carrier and was kindly provided by Chongqing KOPPERCHEM Industry Co., Ltd (China). CuSO4·5H2O, NiSO4·6H2O, ZnSO4·7H2O and CoSO4·7H2O were supplied by Shanghai Aladdin Co., Ltd and used without any pretreatment. Tetrahydrofuran (THF) served as a solvent for the plasticizer and was purchased from Shanghai Aladdin Co., Ltd. Throughout the experiment, ultrapure water with a resistance of 18.2 MΩ cm, prepared using a Milli-Q System, was used.
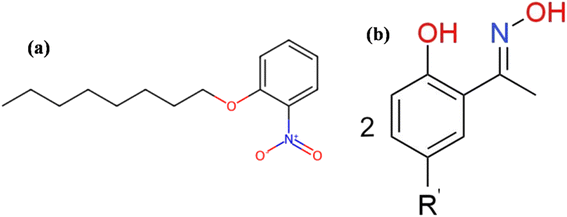 |
| Fig. 1 The chemical structure of NPOE (a) and Mextral®5640H (b). | |
2.2 Methods
2.2.1 Polymer inclusion membranes (PIMs) and feed solutions preparation. The solvent evaporation method30 was utilized to create PIMs. Initially, distinct membrane components (PVC, NPOE, and Mextral®5640H) with different ratios and weighing 0.5 g in total were dissolved in 10 mL THF. These solutions were then transferred into a glass culture dish with a diameter of 6 cm. Allowing THF to slowly evaporate of at ambient temperature for 12 h resulted in flexible, colorless, and transparent membranes with robust mechanical properties, referred to as Mextral®5640H-based PIMs. Subsequently, the obtained membranes were then carefully peeled off from a glass culture dish and stored for subsequent experiments. For the feed solutions, accurate masses of CuSO4·5H2O, NiSO4·6H2O, ZnSO4·7H2O and CoSO4·7H2O were weighed, dissolved in ultrapure water, and diluted to a specific volume. The pH of the resulting feed solution was adjusted to 5.0 by diluted sulfuric acid.
2.2.2 Selective extraction of metal ions. The optimization of the selective metal ion extraction process was carried out using an in-house separation apparatus depicted in Fig. 2, with an effective area of the membrane of 13.27 cm2. In a thermostatic water bath, equal volumes (200 mL) of both the feed and stripping solutions were maintained at a constant temperature. Peristaltic pumps were employed as the power device to circulate the feed solution and stripping solution at a flow rate of 100 mL min−1 during the membrane extraction process. At specific one-hour intervals, 0.2 mL aliquots were transferred from the compartment of the stripping solution to determine the metal ions concentration using ICP-OES (AGILENT 725-ES, USA). The metal ion concentrations in the organic phase were determined by calculating the difference.
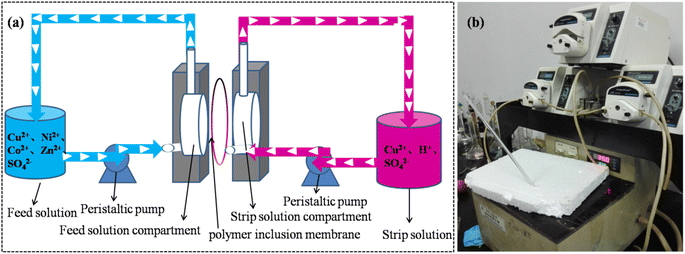 |
| Fig. 2 The schematic diagram (a) and digital image (b) of the selective extraction apparatus. | |
As depicted in Fig. 2, the feed solution containing Cu2+, Co2+, Ni2+ and Zn2+ was passed through the feed solution compartment. Here, the extractable metal ions were selectively transferred into the membrane matrix at the membrane/feed solution interface. This transfer occurred due to the differences in the coordination ability and stability of the coordinated complexes between Mextral®5640H and Cu2+, Co2+, Ni2+ and Zn2+. Subsequently, the organometallic complexes diffused to the opposite side of the membrane surface. These complexes were further stripped with diluted sulfuric acid in the stripping solution compartment at the solid/liquid interface. Finally, Mextral®5640H in the membrane matrix was regenerated through stripping and diffused to the membrane/feed solution interface. This process allowed for the selective extraction of metal ions from the feed solution compartment, followed by continuous stripping with diluted sulfuric acid, achieving the separation of valuable metal ions using Mextral®5640H-based PIMs.
The extraction efficiency (E), distribution ratio (D), and separation coefficient (β) were calculated as follows:
|
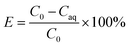 | (1) |
|
 | (2) |
|
 | (3) |
In these equations, C0 (mg L−1) represents the concentration of Cu2+, Co2+, Ni2+ and Zn2+ in the feed solution, and Caq represents the concentration of these ions in the tripping solution at specific point in time, respectively.
2.2.3 Computational details. The theoretical calculations were performed using the Gaussian 16 suite of programs.39 The structures of the studied molecules and their complexes with four metal cations (Co2+, Cu2+, Ni2+, Zn2+) were fully optimized at the PBE0-D3BJ/def2-SVP level of theory. The vibrational frequencies of the optimized structures were computed at the same level. Each structure was confirmed as a local energy minimum on the potential energy surface by verifying that all the vibrational frequencies were real.
2.2.4 Membranes characterization. The FT-IR spectra of Mextral®5640H were recorded on a Nicolet 6700 FT-IR spectrophotometer (Thermo Fisher Scientific, USA) using KBr pellets. 32 scans at a resolution of 4.0 cm−1 were averaged to obtain each spectrum over the wavenumber range of 400–4000 cm−1. The FT-IR spectra of the membranes were recorded directly. All spectrum measurements were performed at ambient temperature. The full aperture size distribution of the membranes were determined using the Brunauer–Emmett–Teller (BET) model from nitrogen adsorption and desorption isotherms. These isotherms were measured at the temperature of liquid nitrogen on an ASAP 2460 (Mcmurretik Instrument Co., Ltd (Shanghai)) surface area analyzer with adsorption data in the relative pressure range from 0.05 to 0.995.40,41
3. Results and discussion
3.1 Optimization of the membrane extraction process and stability tests
3.1.1 Effects of membrane components on efficiency and selectivity of copper extraction. The efficiency and selectivity of copper extraction greatly depend on the membrane properties and the amount of carrier molecules incorporated within the membrane. The extraction of copper(II) largely depend on how it interacts with the carrier (Mextral®5640H), so, it is important to use the right amount of this carrier.42 In the present work, we used PVC as the frame work of polymer membrane. If there is too much PVC, the mass transfer rate is reduced, but too little PVC can decrease the mechanical performance of the membrane. Therefore, we keep a mass fraction of PVC between 30 and 40%.42 In addition, NPOE was used as the plasticizer. When its content goes above 40% (by mass), the membranes become too sticky and soft, which makes them unsuitable for selectively extraction of metal ions.42 However, if the NPOE content is below 40%, increasing its amount does improve copper extraction efficiency. Therefore, we also keep the content of NPOE between 30 and 40%. Additionally, since the amount of carrier we can use is limited, we should keep the concentration of metal ions in the feed solution low to optimize the extraction process.Therefore, to determine the optimal components for the efficient extraction of Co2+, Cu2+, Ni2+, and Zn2+, we varied the mass fraction of Mextral®5640H, PVC and NPOE. The amount of Mextral®5640H was kept constant at 30%, and the mass fraction of NPOE and PVC was adjusted. Fig. 3 illustrates that the Cu2+ extraction efficiency and membrane selectivity increased with a higher amount of NPOE plasticizer. At 40% NPOE, cooper was nearly 100% extracted, and the separation coefficients β with respect to Ni2+, Zn2+, and Co2+ were calculated to be 210
357, 98
148 and 171
904, respectively, after 7 hours of equilibration. The separation coefficients βCu/Ni in Fig. 3a and both βCu/Ni and βCu/Co in Fig. 3b fluctuate slightly with the increase of extraction time. This may be due to differences in the extraction kinetics of Cu2+ compared with those of Ni2+ and Co2+.43 However, when the NPOE plasticizer exceeded 40%, the resulting PIMs became too soft and sticky, impeding the transport of extractable metal ions. Therefore, we propose the optimal mass fraction between Mextral®5640H, PVC and NPOE to be 3
:
3
:
4.
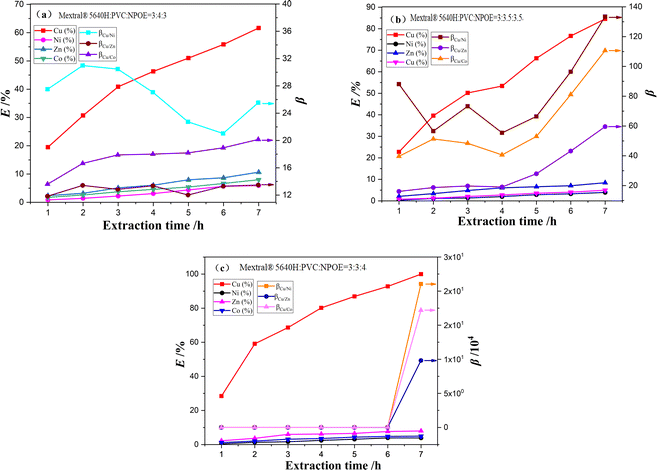 |
| Fig. 3 The effects of membrane composition on the extraction efficiency (E) and separation coefficient (β) of PIMs. | |
3.1.2 Effect of H2SO4 concentration on efficiency and selectivity of copper extraction. The back-extraction process plays an important role in the extraction of metal ions. Therefore, we investigated the impact of H2SO4 concentration in the stripping solutions on extraction efficiency (E) and separation coefficient (β). All extraction experiments were conducted at 25 °C with a fixed mass fraction between Mextral®5640H, PVC and NPOE at 3
:
3
:
4. Due to strong coordination and the formation of a stable complex between Mextral®5640H and Cu2+, the stripping solution required relatively high concentration of H2SO4 to achieve an efficient back-extraction.As depicted in Fig. 4, an increase in H2SO4 concentration in the stripping solution from 0.3 to 0.9 mol L−1 led to a rise in the extraction efficiency of Cu2+ after 7 hours from 40.31% to 99.98%. Simultaneously, the separation coefficient between Cu2+and Ni2+, Zn2+, and Co2+ reached 921
255, 485
027 and 834
601, respectively. The significant fluctuations of separation coefficients occur when the H2SO4 concentration in the stripping solution exceeds 0.9 mol L−1. These fluctuations could be attributed to the low extraction efficiency of Ni2+, Zn2+, and Co2+ in the early stage of extraction. Meanwhile, the extraction efficiency of Cu2+ rapidly increases with extraction time, while other metals are extracted negligibly. This results in high selectivity for copper extraction. However, at higher sulfuric acid concentrations, the extraction efficiency of Cu2+ decreased from 100% to 21.57%, while the extraction efficiency of Ni2+, Zn2+, and Co2+ reached 3.23%, 3.83% and 2.77%, resulting in a decrease in the separation coefficient between Cu2+and Ni2+, Zn2+, and Co2+ to 8.23, 6.91 and 9.93, respectively. The lower extraction efficiency of Cu2+ at higher H2SO4 concentrations in the stripping solution can be attributed to the decreased stability of carriers incorporated into polymer inclusion membranes, while the increased extraction efficiency of Ni2+, Zn2+, and Co2+(ref. 38) contributes to this effect. Hence, we chose 0.9 mol L−1 H2SO4 in the stripping solution for the subsequent experiments.
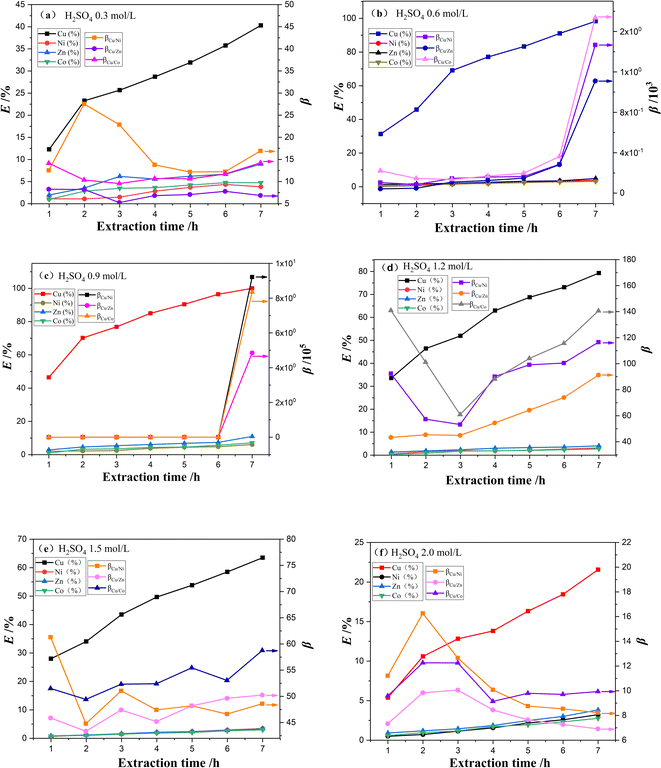 |
| Fig. 4 The effect of H2SO4 concentration in the stripping solution on E and β values of four precious metal cations. | |
3.1.3 Effects of temperature on extraction efficiency and separation coefficient. Higher temperatures accelerate chemical reactions, potentially aiding the extraction of copper from the aqueous medium. Therefore, we investigated the effects of the temperature of stripping and feed solutions on the extraction efficiency (E) and separation coefficient (β). All extraction experiments were conducted with a 0.9 mol L−1 H2SO4 concentration in the stripping solution and a 3
:
3
:
4 mass fraction of Mextral®5640H, PVC and NPOE in the PIM membrane. The temperature of stripping and feed solutions was controlled between 25 and 65 °C using a thermostatic water bath.As depicted in Fig. 5, the extraction efficiency of Cu2+ and separation coefficients increased significantly. The extraction efficiency of Cu2+ increased from 96.47% to 100%, and the separation coefficient between Cu2+ and Ni2+, Zn2+, and Co2+ increased about 104 times as the extraction temperature rose from 25 °C to 35 °C after 6 hours of extraction. However, with further increases in extraction temperature from 35 °C to 65 °C, the extraction efficiency of other valuable metals increased slowly, resulting in decrease in the separation coefficient between Cu2+ and Ni2+, Zn2+, and Co2+. This can be attributed to the increased mass transfer rate of Ni2+, Zn2+ and Co2+ at elevated temperatures and the decreased efficiency copper extraction. Therefore, the optimal extraction temperature was determined to be 35 °C.
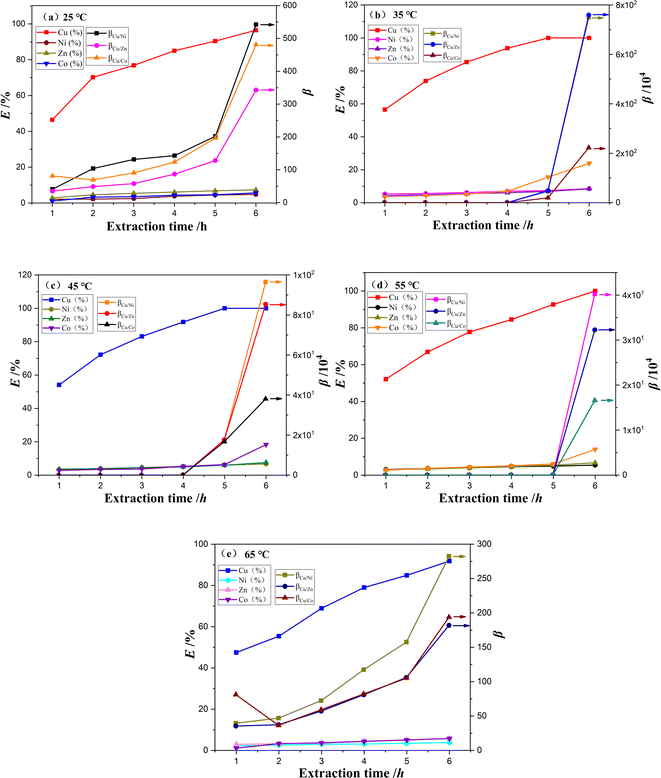 |
| Fig. 5 The effect of temperature on the extraction efficiency (E) and separation coefficient (β). | |
3.1.4 Chemical stability of PIM. We next evaluated the chemical stability PIMs during the extraction process. After selectively extracting copper from acidic solutions containing multiple metals for 300 hours, no significant weakening of the mechanical properties or reduction of extraction efficiency was observed. However, with the extended extraction time, a light-green color appeared on the exposed surface of the PIM. This color may originate from the reaction of Mextral®5640H in the PIM with Cu2+.43 Washing with a dilute sulfuric acid solution, removed the color, resulting from the back extraction of Cu2+.44
3.2 FT-IR studies of the extraction mechanism of PIMs
To understand the membrane extraction mechanism, we recorded the FT-IR spectra of the extractant (Mextral®5640H), the unloaded polymer inclusion membrane (PIM), and the PIM loaded with metal ions.
As displayed in Fig. 6, the strong vibration peaks located around 2963–2856 cm−1 (Fig. 6a–c) corresponded to the C–H groups of aliphatic and phenol groups in Mextral®5640H and NPOE.45 The band at 1624 cm−1 (Fig. 6a) assigned to the C
N stretching vibration in Mextral®5640H shifted to 1608 cm−1 (Fig. 6b) due to interactions between Mextral®5640H and NPOE. Furthermore, the interaction between the Mextral®5640H oxime group with metal ions induced a more pronounced peak shift to 1582 cm−1 (Fig. 6c).30,45 The absorption peak at 1384 cm−1 originating from the deformation vibration of the phenolic –O–H group in Mextral®5640H disappeared in PIMs (Fig. 6b and c) due to intermolecular hydrogen-bonding with the plasticizer.45 The vibration band at 3398 cm−1 (Fig. 6a), attributed to –OH stretching vibrations of the extractant, was preserved in the unloaded PIM (Fig. 6b). However, the peak broadened and shifted to 3405 cm−1 due to hydrogen-bonding between Mextral®5640H and NPOE. This peak disappeared in the loaded PIM (Fig. 6c) due to the coordination of the extractant with metal ions, which disrupted the inter- and intramolecular hydrogen bonding of Mextral®5640H.30,45 Additional FT-IR peaks observed at 1161 cm−1, 1353 cm−1 and1527 cm−1 were attributed to C–O–C, C–N, and C–NO2 groups of NPOE (Fig. 6b and c).45 Considering all these results, we propose the structure of extracted complexes as displayed in Fig. 7, where M represents Cu2+, Ni2+, Co2+ and Zn2+, respectively.
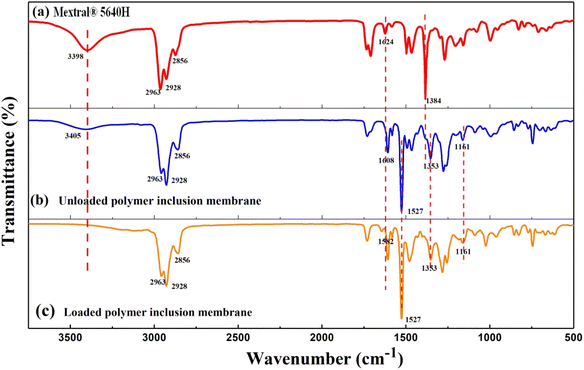 |
| Fig. 6 FT-IR spectra of Mextral®5640H (a), unloaded PIM (b), and loaded PIM (c). | |
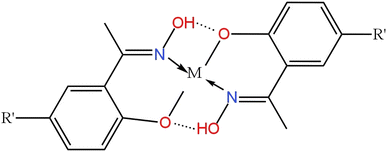 |
| Fig. 7 The plausible structure of the extracted complexes. M represents Cu, Ni, Co, or Zn ions. | |
3.3 Pore size distribution of unloaded PIM
The microstructure of membranes, including factors like pore size, distribution, and membrane thickness, plays a pivotal role in their separation performance. These characteristics are particularly important in processes like microfiltration and ultrafiltration, where separation is mainly determined by pore size. Larger substances are retained while smaller substances pass through, highlighting the crucial role of microstructure in the separation mechanism. In this context, we utilized the BET model based on nitrogen adsorption and desorption isotherms to investigate the pore size of the PIM. The analyzed sample consisted of a membrane synthesized under optimum composition for maximizing extraction yield, i.e. 3
:
4
:
3 mass ratio of Mextral®5640H, NPOE and PVC. The results are shown in Fig. 8.
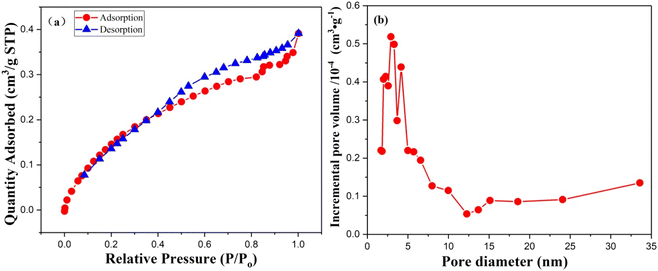 |
| Fig. 8 BET isotherms (a) and pore size distribution (b) of unloaded PIM. | |
The gas adsorption isotherms presented in Fig. 9 indicate a type IV isotherms, as illustrated in Fig. 7a. Moreover, examining the hysteresis loops and their corresponding pore shapes (Fig. 10) classifies ring the PIM membrane's hysteresis ring (Fig. 8a) as type A. A smaller hysteresis ring suggests relatively high membrane porosity, characterized by cylindrically-shaped pores. Fig. 8b illustrates that the majority of the membrane pores have diameters ranging from 2.0 to 5.0 nm. The calculated average pore diameter is 3.2686 nm, with numerous pores larger exceeding 3.5 nm. If pore size significantly influences extraction efficiency and separation coefficient, the diameter of the Cu2+–Mextral®5640H complex should be smaller than the average pore size. Conversely, complexes of the other three metal cations (Ni2+, Zn2+, and Co2+) with the extractant should be larger than the membrane's pore size.
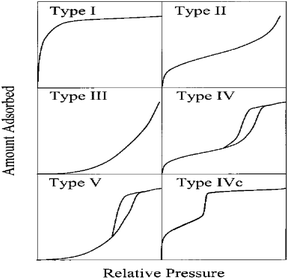 |
| Fig. 9 Classification of gas adsorption isotherms.46 | |
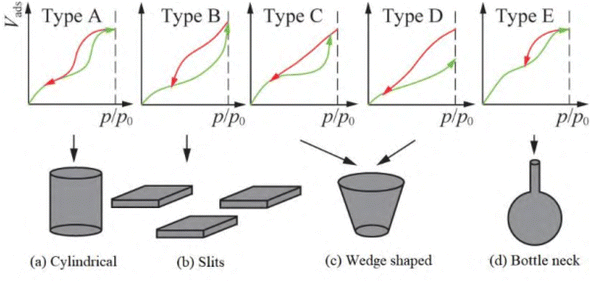 |
| Fig. 10 Classification of hysteresis loops of adsorption isotherms and their corresponding pore shapes.46 | |
3.4 Structural analysis of the extractant-metal ion complexes
The metal extraction experiments revealed a significant difference in the extraction performance of PIMs toward Cu2+ compared with Ni2+, Zn2+, and Co2+. To gain more insights into the structural basis of this trend, we optimized the structures of the four hypothesized coordination compounds to obtain information on their molecular size and stability. The results of quantum chemical calculations are illustrated in Fig. 11 and summarized in Tables 1 and 2.
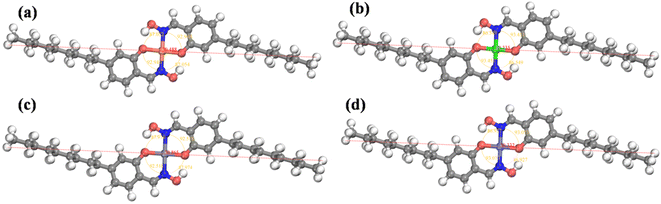 |
| Fig. 11 The optimized geometry and molecular size of the extractant-metal ion complexes for Cu (a), Ni (b), Zn (c), and Co (d). | |
Table 1 The total energy, interaction energy, and molecular size of complexes of each of the four metal cations with the extractant
System |
Energy/Hartree |
Interaction energy/Hartree |
Interaction energy/kcal mol−1 |
Molecular size/Å |
Mextral®5640H + Cu2+ |
−3038.12450 |
−0.05255 |
−32.97756 |
30.448 |
Mextral®5640H + Ni2+ |
−3163.64661 |
−0.10503 |
−65.90732 |
30.335 |
Mextral®5640H + Zn2+ |
−3434.72654 |
−0.03891 |
−24.41724 |
30.865 |
Mextral®5640H + Co2+ |
−3295.79823 |
−0.04226 |
−26.51646 |
30.332 |
Table 2 Enthalpy, Gibbs free energy and entropy- of the formation of metal extractant complexes
System |
ΔH/kcal mol−1 |
ΔG/kcal mol−1 |
ΔS/cal K−1 |
Mextral®5640H + Cu2+ |
−23.801075 |
−7.60787345 |
−0.054339603 |
Mextral®5640H + Ni2+ |
−27.3597875 |
−13.4705002 |
−0.046608347 |
Mextral®5640H + Co2+ |
−37.22597 |
−21.8255475 |
−0.05167927 |
Mextral®5640H + Zn2+ |
−40.56427 |
−26.76592875 |
−0.046303159 |
Evidently, the sizes of all four organometallic compounds, ranging from 30.3 to 30.9 Å, were smaller than the average pore diameter (3.2686 nm). This implies that molecular size is not the main factor influencing the separation mechanism of these metal cations. The calculated potential energies for the four complexes (Table 1) highlighted that the complex with Cu2+ ions has the largest overall energy, i.e. the lowest stability, which is beneficial for the back-extraction of Cu2+–Mextral®5640H complex. The enthalpy and entropy of the reaction as well as Gibbs free energy of the complexes shown in Table 2 indicate that the complex with Cu2+ has lower stability compared to those with Ni2+, Zn2+, and Co2+. As discussed in Section 3.1.2, the increased acid concentration in the stripping solution improved the extraction of Ni2+, Zn2+, and Co2+ and thereby reduced the separation coefficients. The results of theoretical calculations support these results, as more stable complexes correlate with lower extraction rates and poorer extraction performance. Therefore, the stability and back-extraction performance played a significant role in the selective extraction of copper from the feed solution.
4. Conclusions
In summary, our study proposes an innovative integration of solvent extraction and PIM membrane separation technology to fabricate a novel PIM membrane material. This material demonstrates efficient and selective separation and enrichment of strategic valuable metals, particularly copper(II), from polymetallic acidic solutions. We systematically explored the impact of PIM composition, extraction temperature, and H2SO4 concentration in the stripping solution on extraction and separation performance.
The optimization process identified the ideal extraction conditions as a mass fraction of Mextral®5640H
:
PVC
:
NPOE = 3
:
3
:
4, extraction temperature at 35 °C, and 0.9 mol L−1 H2SO4 in the stripping solution. Under these conditions, we achieved impressive extraction efficiencies of 100% for Cu2+, 8.53% for Ni2+, 8.43% for Co2+, and 23.89% for Zn2+.
To explain the substantial variation in extraction performance between Cu and the other metals, we employed the comprehensive approach. The FT-IR spectra, BET analysis, and theoretical calculations provided crucial structural insights, including molecular size and potential energy, for copper and the other metal-ion extracted complexes within the PIM. The results underscored that all the extracted complexes molecular could pass through the PIMs in terms of molecular size. Hence, stability and back-extraction performance were identified as crucial for the selective extraction of copper with respect to the other three metal ions. The success of this synergistic approach lays the foundation for advancements in the field of metal separation technologies. Future research directions could be oriented towards scaling up this methodology for industrial applications, with a focus on optimizing process parameters for enhanced efficiency and environmental sustainability.
Conflicts of interest
There are no conflicts to declare.
Acknowledgements
This work was financially supported by Basic Research Project of Key Field Project of Natural Science Research of Guizhou Provincial Department of Education (Qian Jiao He KY Zi [2020]049), Scientific Research (Cultivation) Project of Liupanshui Normal University (LPSSY2023KJZDPY05), Liupanshui Science and Technology Project (52020-2023-0-2-17), Carbon Neutral Engineering Research Center of Guizhou colleges and universities in Coal Industry (Qian Jiao Ji [2023]044), Guizhou Province first-class professional construction point (GZSylzy202103) and Innovation Team Foundation of Education of Guizhou Province (Qian Jiao Ji [2023]087).
Notes and references
- Z. H. Liu, Present situation and development of non ferrous metallurgy technology, World Nonferrous Metals, 2020,(12), 13–14 CAS.
- China Nonferrous Metals Industry Association, China Nonferrous Metals Industry Year Book, China Nonferrous Metals Industry Association, Beijing, 2014 Search PubMed.
- R. H. Lin, The current situation and prospects of the development of non-ferrous metal mineral resources in China, China Metal Bulletin, 2011,(35), 2–7 CAS.
- R. G. Cui, S. C. Liu and J. Guo, et al., The characteristics of the situation of mineral resources in China in 2012, China Mining Magazine, 2013, 22(1), 1–4 Search PubMed.
- L. G. Meng and Z. Q. Sun, Development of Mineral Resources and Sustainable Development, Mod. Min., 2010,(10), 10–12 Search PubMed.
- X. Li, B. Li and S. Wu, et al., Silica-Based 2-Aminomethylpyridine Functionalized Adsorbent for Hydrometallurgical Extraction of Low-Grade Copper Ore, Ind. Eng. Chem. Res., 2012, 51(46), 15224–15232 CrossRef CAS.
- B. Pospiech, Synergistic Solvent Extraction and Transport of Zn(II) and Cu(II) across Polymer Inclusion Membranes with a Mixture of TOPO and Aliquat 336, Sep. Sci. Technol., 2014, 49(11), 1706–1712 CrossRef CAS.
- J. T. Li and A. L. Chen, Deep removal of copper from nickel electrolyte using manganese sulfide, Trans. Nonferrous Met. Soc. China, 2015, 25(11), 3802–3807 CrossRef CAS.
- X. Qiu, H. Hu and J. Yang, et al., Selective removal of copper from simulated nickel electrolyte by polystyrene-supported 2-aminomethylpyridine chelating resin, Chem. Pap., 2018, 72(8), 2071–2085 CrossRef CAS.
- L. Bai, H. Hu and W. Zhang, et al., Amine/acid catalyzed synthesis of a new silica-aminomethyl pyridine material as a selective adsorbent of copper, J. Mater. Chem., 2012, 22(33), 17293–17301 RSC.
- M. Fu, J. Hu and Y. Li, et al., A novel strategy achieving enrichment of metal values from and into ammoniacal solutions, Sep. Purif. Technol., 2015, 151, 97–101 CrossRef CAS.
- X. Wu, S. Zhu and S. Li, Synergistic Extraction Mechanism of Versatic10 and LIX63 for Ni2+, Co2+, Mn2+, Hydrometallguty, 2020, 39(01), 34–40 Search PubMed.
- S. Zhu, H. Hu, S. Li and C. Wang, The application of structural analysis in the investigation of solvent extraction mechanism, J. Coord. Chem., 2022, 5–6(75), 549–572 CrossRef.
- W. Zhang, C. Y. Cheng, M. Rodriguez, et al., Separation of copper, iron and zinc from cobalt and nickel with mixed Cyanex 272 and LIX84 extractants, in Proceedings International Solvent Extraction Conference ISEC, 2008, vol. 1, pp. 177–182 Search PubMed.
- C. Y. Cheng, W. Zhang and Y. Pranolo, Separation of Cobalt and Zinc from Manganese, Magnesium, and Calcium Using a Synergistic Solvent Extraction System Consisting of Versatic 10 and LIX 63, Solvent Extr. Ion Exch., 2010, 28(5), 608–624 CrossRef CAS.
- D. Dreisinger, C. Y. Cheng, W. S. Zhang, et al., Development of the Boleo process flowsheet and the Direct Solvent Extraction (DSX) circuit for cobalt and zinc recovery, in Proceedings XXV International Mineral Processing Congress, 2010, pp. 309–317 Search PubMed.
- C. Y. Cheng, K. R. Barnard and W. Zhang, et al., Synergistic Solvent Extraction of Nickel and Cobalt: A Review of Recent Developments, Solvent Extr. Ion Exch., 2011, 29(5–6), 719–754 CrossRef CAS.
- S. Zhu, H. Hu and J. Hu, et al., Insights into the extraction mechanism from the coordination chemistry of copper(II) with a synergistic mixture which mimics Versatic10 and 2-ethylhexyl 4-pyridinecarboxylate ester, J. Coord. Chem., 2017, 19(70), 3325–3337 CrossRef.
- S. Zhu, H. P. Hu, J. Y Li and F. Hu, et al., The Coordination Structure of the Extracted Nickel(II) Complex with a Synergistic Mixture Containing Lix63 and Versatic10, J. Chin. Chem. Soc., 2017, 64, 205–216 CrossRef.
- C. Y. Cheng, G. Boddy and W. Zhang, et al., Recovery of nickel and cobalt from laterite leach solutions using direct solvent extraction: part 2: semi- and fully-continuous tests, Hydrometallurgy, 2010, 104(1), 53–60 CrossRef CAS.
- C. Y. Cheng, M. D. Urbani and M. G. Davies, et al., Recovery of nickel and cobalt from leach solutions of nickel laterites using a synergistic system consisting of Versatic 10 and Acorga CLX 50, Miner. Eng., 2015, 77, 17–24 CrossRef CAS.
- C. Y. Cheng, K. R. Barnard and W. Zhang, et al., Recovery of nickel, cobalt, copper and zinc in sulphate and chloride solutions using synergistic solvent extraction, Chin. J. Chem. Eng., 2016, 24(2), 237–248 CrossRef CAS.
- Z. Zhu, W. Zhang and Y. Pranolo, et al., Separation and recovery of copper, nickel, cobalt and zinc in chloride solutions by synergistic solvent extraction, Hydrometallurgy, 2012, 127–128(18), 1–7 CrossRef CAS.
- J. Y. Li, Study on the Selective Extraction of Nickel from Acid Leach Liquor of Laterite Nickel Ore and Its Synergistic Extraction Mechanism, Central South University, Changsha, 2017 Search PubMed.
- D. Wang, Q. Chen and J. Hu, et al., High Flux Recovery of Copper(II) from Ammoniacal Solution with Stable Sandwich Supported Liquid Membrane, Ind. Eng. Chem. Res., 2015, 54(17), 4823–4831 CrossRef CAS.
- M. Baczyńska, M. Waszak and M. Nowicki, et al., Characterization of Polymer Inclusion Membranes (PIMs) Containing Phosphonium Ionic Liquids as Zn(II) Carriers, Ind. Eng. Chem. Res., 2018, 57(14), 5070–5082 CrossRef.
- X. Lin, J. Tang and Y. Liu, et al., Membrane-assisted enrichment of zinc(II) from and into ammoniacal media through non-dispersive synergistic extraction, Sep. Purif. Technol., 2019, 210, 268–275 CrossRef CAS.
- A. Yadollahi, M. Torab-Mostaedi and K. Saberyan, et al., Intensification of zirconium and hafnium separation through the hollow fiber renewal liquid membrane technique using synergistic mixture of TBP and Cyanex-272 as extractant, Chin. J. Chem. Eng., 2019, 27(8), 1817–1827 CrossRef CAS.
- H. Duan, X. Yuan and Q. Zhang, et al., Separation of Ni2+ from ammonia solution through a supported liquid membrane impregnated with Acorga M5640, Chem. Pap., 2017, 71(3), 597–606 CrossRef CAS.
- D. Wang, J. Hu and D. Liu, et al., Selective transport and simultaneous separation of Cu(II), Zn(II) and Mg(II) using a dual polymer inclusion membrane system, J. Membr. Sci., 2016, 524, 205–213 CrossRef.
- T. Tasaki, T. Oshima and Y. Baba, Selective extraction and transport of copper(II) with new alkylated pyridinecarboxylic acid derivatives, Talanta, 2007, 73(2), 387–393 CrossRef CAS PubMed.
- M. I. G. S. Almeida, R. W. Cattrall and S. D. Kolev, Recent trends in extraction and transport of metal ions using polymer inclusion membranes (PIMs), J. Membr. Sci., 2012, 415–416, 9–23 CrossRef CAS.
- R. Tayeb, C. Fontas and M. Dhahbi, et al., Cd(II) transport across supported liquid membranes (SLM) and polymeric plasticized membranes (PPM) mediated by Lasalocid A, Sep. Purif. Technol., 2005, 42(2), 189–193 CrossRef CAS.
- M. Ulewicz and E. Radzyminska-Lenarcik, Supported Liquid (SLM) and Polymer Inclusion (PIM) Membranes Pertraction of Copper(II) from Aqueous Nitrate Solutions by 1-Hexyl-2-Methylimidazole, Sep. Sci. Technol., 2012, 47(9), 1383–1389 CrossRef CAS.
- R. Vera, L. Gelde and E. Anticó, et al., Tuning physicochemical, electrochemical and transport characteristics of polymer inclusion membrane by varying the counter-anion of the ionic liquid Aliquat 336, J. Membr. Sci., 2017, 529, 87–94 CrossRef CAS.
- F. J. Alguacil, F. A. Lopez and I. Garcia-Diaz, Copper removal from acidic wastewaters using 2-hydroxy-5-nonylbenzaldehyde oxime as ionophore in pseudo-emulsion membrane with strip dispersion (PEMSD) technology, J. Ind. Eng. Chem., 2012, 18(1), 255–259 CrossRef CAS.
- D. Wang, J. Hu and Y. Li, et al., Evidence on the 2-nitrophenyl octyl ether (NPOE) facilitating copper(II) transport through polymer inclusion membranes, J. Membr. Sci., 2016, 501, 228–235 CrossRef CAS.
- J. P. Yang, H. P. Hu and Z. Y. Cheng, et al., Structural insights into the coordination and selective extraction of copper(II) by tertiary amine ligands derived from 2-aminomethylpyridine, Polyhedron, 2017, 128, 76–84 CrossRef CAS.
- M. J. Frisch, G. W. Tnucks, H. B. Schlegel, et al., Gaussian 16 Revision A.03, Gaussian Inc., Wallingford, CT, 2016 Search PubMed.
- L. Zhang, L. Lin and Y. Zhang, et al., Nickel catalysts supported on MgO with different
specific surface area for carbon dioxide reforming of methane, J. Energy Chem., 2014, 23(1), 66–72 CrossRef CAS.
- X. Li, L. Jiang and C. Zhou, et al., Integrating large specific surface area and high conductivity in hydrogenated NiCo2O4 double-shell hollow spheres to improve supercapacitors, NPG Asia Mater., 2015, 7(3), 165–172 CrossRef.
- D. Wang, R. W. Cattrall and J. Li, et al., A poly(vinylidene fluoride-co-hexafluoropropylene) (PVDF-HFP)-based polymer inclusion membrane (PIM) containing LIX84I for the extraction and transport of Cu(II) from its ammonium sulfate/ammonia solutions, J. Membr. Sci., 2017,(542), 272–279 CrossRef CAS.
- Z. Y. Zhu, W. S. Zhang and Y. Pranolo, et al., Separation and recovery of copper, nickel, cobalt and zinc in chloride solutions by synergistic solvent extraction, Hydrometallurgy, 2012, 127–128, 1–7 CrossRef CAS.
- D. Wang, J. Hu and D. Liu, et al., Selective transport and simultaneous separation of Cu(II), Zn(II) and Mg(II) using a dual polymer inclusion membrane system, J. Membr. Sci., 2017, 524, 205–213 CrossRef CAS.
- L. V. Daimay, B. C. Norman, G. F. William, et al., The Handbook of Infrared and Raman Characteristic Frequencies of Organic Molecules, Academic Press, New York, 1991 Search PubMed.
- S. Zhu, J. Hu, C. Zhang, S. Li and N. An, Study on optimization and mechanism of mechanical activation process of titanium-bearing blast furnace slag, J. Mater. Res. Technol., 2022, 19, 3130–3144 CrossRef CAS.
|
This journal is © The Royal Society of Chemistry 2024 |
Click here to see how this site uses Cookies. View our privacy policy here.