DOI:
10.1039/D4RA02277G
(Review Article)
RSC Adv., 2024,
14, 20837-20855
Porphyrin-based porous organic polymers synthesized using the Alder–Longo method: the most traditional synthetic strategy with exceptional capacity
Received
25th March 2024
, Accepted 27th May 2024
First published on 1st July 2024
Abstract
Porphyrin is a typical tetrapyrrole chromophore-based pigment with a special electronic structure and functionalities, which is frequently introduced into various porous organic polymers (POPs). Porphyrin-based POPs are widely used in various fields ranging from environmental and energy to biomedicine-related fields. Currently, most porphyrin-based POPs are prepared via the copolymerization of specific-group-functionalized porphyrins with other building blocks, in which the tedious and inefficient synthesis procedure for the porphyrin greatly hinders the development of such materials. This review aimed to summarize information on porphyrin-based POPs synthesized using the Alder–Longo method, thereby skipping the complex synthesis of porphyrin-bearing monomers, in which the porphyrin macrocycles are formed directly via the cyclic tetramerization of pyrrole with monomers containing multiple aldehyde groups during the polymerization process. The representative applications of porphyrin-based POPs derived using the Alder–Longo method are finally introduced, which pinpoints a clear relationship between the structure and function from the aspect of the building blocks used and porous structures. This review is therefore valuable for the rational design of efficient porphyrin-based porous organic polymer systems that may be utilized in various fields from energy-related conversion/storage technologies to biomedical science.
1. Introduction
As a new emerging class of multi-dimensional network materials linked via covalent bonds, porous organic polymers (POPs) have attracted a great deal of worldwide research interest.1–3 The intrinsic features of POPs, such as light weight, customized functionality, tunable pores, large specific surface areas, and super stability, as well as excellent structural compatibility, render POPs promising platforms for various applications.4–6 Hitherto, thousands of POPs with various geometries and functions have been developed, including crystalline covalent organic frameworks (COFs) and covalent triazine frameworks (CTFs), as well as amorphous conjugated microporous polymers (CMPs), hyper-crosslinked polymers (HCPs), and polymers of intrinsic microporosity (PIMs). These series of POPs have been vastly used, including in sewage treatment, gas adsorption and separation, heterogeneous catalysis, sensing, energy, and biomedicine fields in the past decades.7–9
As one of the key natural heterocyclic molecules in extant life, porphyrins with unique electronic structures and photophysical properties play vital roles in diverse biological functions such as O2 transport, light harvesting, and electron transfer as well as numerous enzyme-catalytic transformations.10,11 Noteworthily, as a polar, large-conjugated, and highly delocalized organic macrocycle molecule, porphyrin features a strong ability to finely coordinate with various metal cations into the macrocycle center for forming complexes.12 Given these particular properties, porphyrins, especially those modified by aromatic moieties with special functional groups, have been frequently incorporated into various porous materials such as metal–organic frameworks (MOFs), periodic mesoporous silicas (PMSs), graphene, carbon nanotubes (CNTs), and POPs.13–16 Among these porphyrin-bearing porous materials, porphyrin-based POPs with concurrent structural compatibility and strong stability in extreme conditions stand out and have been widely reported on.17
With decades of development, significant achievements have been made in the controlled synthesis of porphyrin-based POPs. To yield multifunctional materials, varieties of organic building blocks bearing special functional properties have been successfully incorporated into porphyrin-bearing POPs via various reactions such as the Yamamoto reaction, Suzuki–Miyaura reaction, Sonogashira–Hagihara reaction, alkyne–alkyne reaction, cyclic trimerization reaction, Schiff-base reaction, and Knoevenagel condensation reaction, as well as the FeCl3-promoted or electrochemical oxidative coupling reaction.18 Hitherto, hundreds of porphyrin-based POPs with diverse structures and functions have been successfully developed.19 Yet, the industrial application of such materials are still fraught with challenges.20 Most of these porous organic polymers are achieved via the self-polymerization or copolymerization of specific-groups-functionalized porphyrins with other building blocks. However, the tedious and inefficient synthesis procedure of the porphyrin-bearing building blocks greatly hampers the development of such materials,21 while the harsh reaction conditions (anhydrous and oxygen-free conditions), together with the usage of noble metal catalysts with long reaction times, greatly increase the production cost of these materials, which also significantly impede the real application of such materials.22 Additionally, the synthesis of other reactive monomers containing special reactive groups that could react with porphyrin-based monomers is also a demanding and laborious process, and their yields are often quite low.23
To address such issues, low-cost, simple, and scalable preparation methods are urgently needed to accelerate the development and application potential of such materials. Recently, the Alder–Longo method has emerged as one of the most promising methods for the targeted preparation of porphyrin-based POPs.24 Different from other reactions, the Alder–Longo method allows realizing the synthesis of porphyrin-based POPs from the cyclic tetramerization of pyrrole with monomers containing multiple aldehyde groups. Porphyrin macrocycles can be formed directly during the polymerization process without needing to use any high-cost porphyrin-containing monomers, or noble metal catalysts, and with an ultrahigh yield.25 Similar to other reactions, the Alder–Longo method can also realize the effective design and adjustment of the structures and functions of porphyrin-based POPs, which could also hold a wealth of potential applications across various fields.
This review aimed to highlight the advances in the design and synthesis of porphyrin-based POPs, from the direct cyclic tetramerization of pyrrole with polyaldehyde monomers via the Alder–Longo method, in which the complex synthesis procedure of the porphyrin monomers could be skipped. Additionally, this work aimed to introduce the representative synthesis and applications of the obtained porphyrin-based POPs, pinpointing a clear relationship between the structure and function from the aspect of the building blocks used and porous structures. Also, we tried to introduce the specific sustainable applications of those materials in different fields, such as industrial catalysts, therapeutic agents for drug-resistant diseases, or key components in energy-storage and -conversion devices. Some original perspectives toward both fundamental research and the application efforts are briefly discussed at the end of this review.
2. Alder–Longo method for the synthesis of porphyrin-based POPs
Numerous studies have validated that, as cutting-edge porous materials, POPs with a high specific surface area and backbone robustness are excellent adsorbents materials for heavy metal ions and toxic gases. The Alder–Longo synthesis method, also known as the direct cyclic tetramerization of pyrroles with aromatic monomers bearing di/tris/tetra/penta/hexa aldehydes, offers stable yields and a relatively simple synthesis procedure (Scheme 1) for the preparation of porphyrin-based POPs. This approach is based on the acidic condensation of aryl aldehydes with pyrrole at a relatively high temperature, followed by oxidation, and this has been known as the Adler–Longo porphyrin synthesis since 1967.26 This special synthesis method involves simple aromatic electrophilic substitution on pyrrole with aromatic aldehydes-containing blocks, in which the porphyrin units are formed in situ during the one-pot polymerization, which provides a facile, low-cost, bottom-up one-pot self-polymerization strategy for porphyrin-based POPs, rendering these materials suitable for practical applications.
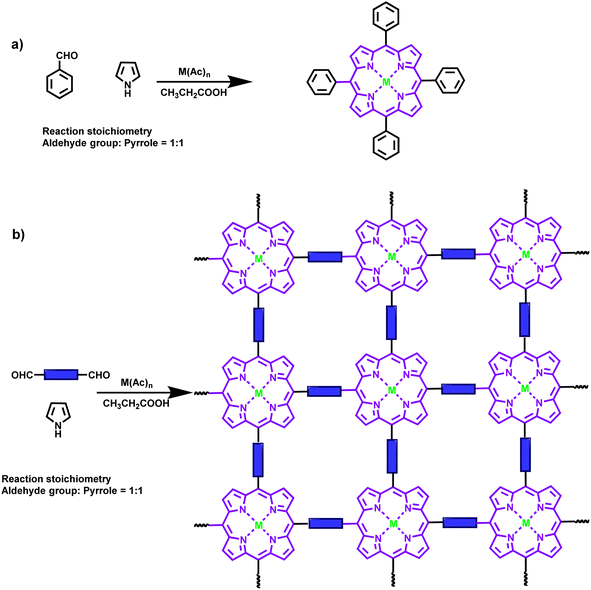 |
| Scheme 1 Schematic route for the synthesis of (a) porphyrin and (b) porphyrin-based POPs via the Alder–Longo method and the ideal structures of these series of POPs. | |
With years of development, great advances and innovations have been achieved in the further design, synthesis, and application of porphyrin-based POPs via the Alder–Longo method. Since the first report by Asim Bhaumik's group in 2012, the last decade has witnessed an upsurge in the development and synthesis of porphyrin-based POPs via the Adler–Longo method.27 Hitherto, various multiple-aldehyde-containing building blocks with different functional substituents, geometries, and conjugation sizes have been used for the synthesis of POPs with diverse pore sizes and functionalities. The Alder–Longo method is regarded as a scalable synthetic method for the preparation of POPs with specific structures and functions, which have been widely used in numerous fields ranging from the environment, energy, organic catalysis, and biomedical fields.
3. Applications of porphyrin-based POPs obtained from the Alder–Longo method
As a new class of artificial porous materials, porphyrin-based POPs with repetitive porphyrin units and open porous structure have attracted considerable research interest for various applications.28 Just as other materials, the properties of porphyrin-based POPs are highly dependent on the characteristic of their skeleton structure and composition. Also, the composition of porphyrin-based POPs is not only decided by the aldehyde-containing building blocks, but also the metal centers coordinated in the porphyrin skeleton. It was reported that the N-rich structure and π-conjugated skeleton in the porous channel can act as adsorbates to capture radiative iodine pollutants form the environment.29 The unique structure of porphyrin renders the material with photo-, electro-, and intrinsic catalytic activity through the porous skeleton. The aldehyde-containing building blocks decide the final skeleton of POPs, such as the porosity and conjugated structure, which are highly relevant for the absorption, mass transfer, conductivity, charge transport, as well as the catalytic efficiency of materials. For example, the inherent porous skeleton offers adsorption sites for various gas and non-gaseous pollutants from the environment.30 The metal center decides the catalytic activity of the materials, which is also influenced by the porosity and skeleton structure of the POPs. Furthermore, the remaining component of the aldehyde-containing units can not only work synergistically with the porphyrin units to achieve additional effects, but can also introduce other desired functions into the porous skeleton. Thereby, porphyrin-based porous organic polymers with brand new structures and functions can be easily obtained via the fine adjustment of the structures and functions of the aldehyde-containing units, as well as the species of metal ion utilized during the polymerization.
Recently, these series of POPs have been demonstrated to be suitable for the removal of various gaseous and non-gaseous pollution, selectively for organic-, photo-, and electronic catalysis, as well as for antibacterial and anticancer treatments.31,32 This section introduces the applications of porphyrin-based POPs, focusing on the structural design and preparation of porphyrin-based POPs obtained via the Alder–Longo method and highlighting the relationship between the structure and functions. It is expected this will provide guidance and direction for the structural design of porphyrin-based POPs with different functions.
3.1 Pollution treatment
3.1.1 CO2 uptake. Carbon dioxide (CO2) is the main greenhouse gas inducing global warming, leading to climate change in the last decades.33 However, frequent reports have also demonstrated that CO2 is also a cheap and abundant non-toxic carbon source for the modern chemical industry.34,35 In recent years, the highly selective chemical conversion of CO2 to value-added industrial products, such as methane, alcohol, formaldehyde, or formic acid, has attracted increasing research attention, and is now regarded as one of the most promising methods to realize carbon neutrality.36–40 Porphyrin-based POPs with high BET surface areas and a N-rich skeletal structure, such as tunable metal catalytic domains, offer an excellent application potential for the capture and conversion of CO2.As can be seen from Fig. 1a, Bhaumik's group developed a series of Fe–porphyrin-based POPs (POP-1 to POP-3) via the Alder–Longo method using freshly distilled pyrrole with dialdehyde aromatic monomers (terephthaldehyde, biphenyl-dicarboxaldehyde, and p-terphenyl-dicarboxaldehyde) in the presence of a small amount of Fe(III).27 This series of Fe-POPs possessed relatively high BET surface areas (750 to 875 m2 g−1). These series of POPs exhibited an excellent CO2 capture capacity, not only for absorbing large amounts of CO2 from the atmosphere, but also providing the reversible release of an equivalent amount of CO2 when reducing the pressure.
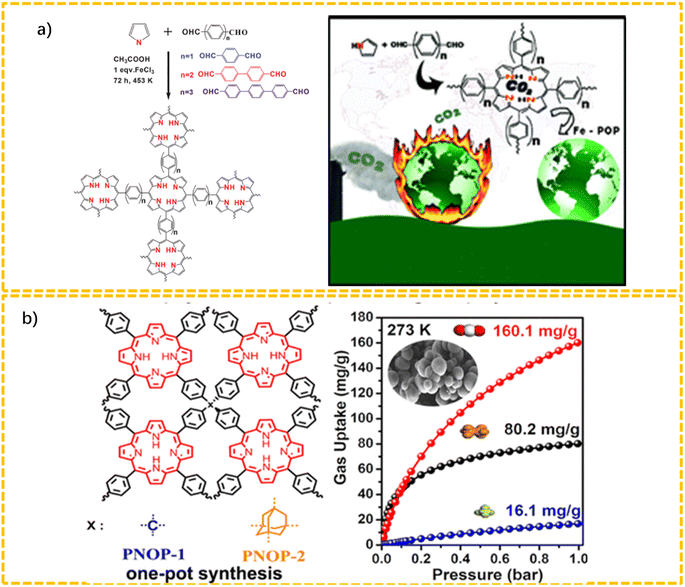 |
| Fig. 1 Porphyrin-based porous organic polymers obtained from the Alder–Longo method for CO2 capture. (a) Schematic route for the synthesis of POP-1 to POP-3; (b) schematic route for the synthesis of PNOPs. | |
As displayed in Fig. 1b, Wang's group designed and synthesized two three-dimensional porphyrin-based nanoporous organic polymers (PNOPs, denoted as PNOP-1 and PNOP-2) via the Alder–Longo method using tetrahedral-structured building blocks, including tetrakis(4-aldehydephenyl)methane (TFPM) and 1,3,5,7-tetrakis(4′-aldehydephenyl)adamantane (TFPAd), respectively.41 The as-prepared materials featured high BET surface areas, which suggested they could serve as ideal materials for the adsorption and separation of CO2.
3.1.2 CO2 conversion. Removing CO2 from the atmosphere and selectively converting it into value-added products, in particular desired commercial products, is a potential strategy for the modern chemical industry.42,43 It is regarded as one of the most effective ways to achieve carbon neutrality, alleviating the pressure caused by rapid economic development on the ecology.44,45 Cyclic carbonates are very common value-added commercial products obtained via the cycloaddition reactions of CO2 and epoxides under the catalysis of homogeneous and heterogeneous catalysts.46Porphyrin-based porous organic polymers with integrated metal catalytic sites are frequently investigated as typical catalysts for the CO2 cycloaddition reaction.47 Li's group synthesized two Co-based porphyrin POPs (Co-Por-POPs) via the Alder–Longo method (Fig. 2a). The as-synthesized Co-Por-POPs presented high catalytic performances in solvent-free CO2 cycloaddition reactions under ambient conditions (25 °C, 1 bar).48 Yang et al. developed a porous zinc-porphyrin-based framework (P-POF-Zn) via a combination of the Alder–Longo method with a post-metallization reaction, and this could be used as a catalyst that worked cooperatively with a thermoresponsive ionic liquid to catalyze the cycloaddition reaction of CO2.49 Also, P-POF-Zn demonstrated excellent recycling ability for the efficient synthesis of cyclic carbonates (Fig. 2b). This study revealed the importance of the combination of a co-catalyst with a heterogeneous POP-based catalyst to effectively catalyze chemical reactions in a synergistic manner.
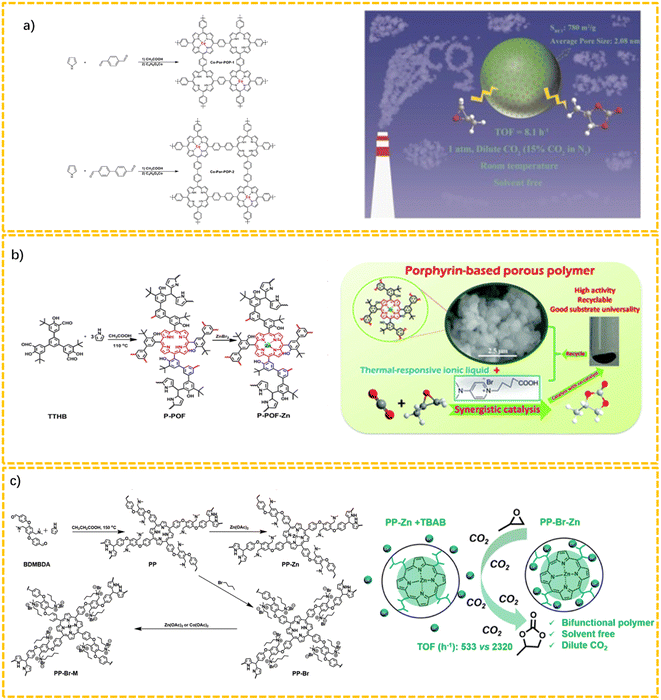 |
| Fig. 2 Fabrication of metal–porphyrin-based porous organic polymers for CO2 cycloaddition reactions. (a) Schematic route for the synthesis of Co-Por-POPs; (b) schematic route for the synthesis of P-POF-Zn; (c) schematic route for the synthesis of PP–Br–Zn. | |
As displayed in Fig. 2c, Liu et al. reported a metal–porphyrin-based cationic polymer (PP–Br–M) through the combination of the Adler–Longo methodology with the post-metalation of quaternary ammonium salts and metal ions using task-specific building blocks (4,4′((2,5-bis((dimethylamino)methyl)-1,4-phenylene)bis-(oxy))dibenzaldehyde, abbreviated as BDMBDA).50 The concurrent existence of Lewis acid active sites (zinc porphyrin) and Br− as nucleophile anions rendered PP–Br–Zn a bifunctional porous organic polymer for the cycloaddition reaction, which could exert a synergistic effect activating both the substrates and related intermediates. The PP–Br–Zn with an optimized Br− and Zn ratio could afford a concurrent high conversion and selectivity (99%) for the cycloaddition of CO2.
3.1.3 Selective adsorption and photocatalytic degradation of cationic dyes. Water pollution, related with both modern industrial productions and pathogen contamination, especially pollution caused by highly toxic, potentially carcinogenic, and mutagenic organic dyes, has become a global safety concern, which pose seriously threats to the both the balance of the ecological environment and the health of living beings.51,52 With years of development, numerous coping strategies have been proposed for the treatment and disposal of cationic dyes, such as ion exchange, ozone treatment, membrane filtration, chemical oxidation, physical absorption, and photocatalytic degradation methods. Among these techniques, the physical absorption and photocatalytic degradation methods, with the inherent characteristics of low-cost, easy operation, and high efficiency, are considered as the two most promising methods.53–56 The inherent structure with porphyrin endows materials with photocatalytic activity, which can then serve as a candidate for the photocatalytic degradation of pollutants.57 Thereby, porphyrin-based POPs hold great potential to realize the concurrent adsorption and photocatalytic degradation of organic pollutants, thereby realizing wastewater detoxification to combat harmful environmental impacts.Liu et al. exploited the application of a porphyrin-based porous organic polymer (Py-POP) for the concurrent adsorption and photocatalysis degradation of cationic dyes, in which methylene blue (MB) and rhodamine B (RhB) were used as model dyes.58 Py-POP was obtained via the polymerization of pyrrole with 4,4′-diformyl-1,10-biphenyl in the presence of ferric chloride. The corresponding experimental results confirmed that Py-POP could be an efficient multifunctional platform that simultaneously integrates adsorption and photocatalysis, and could effectively catalyze the degradation of both dyes under visible-light irradiation (Fig. 3a).
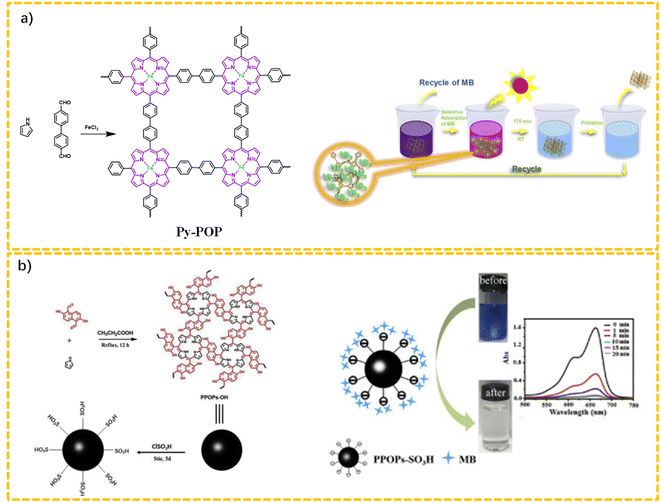 |
| Fig. 3 Typical porphyrin-based porous organic polymers for the capture or degradation of organic dyes. (a) Route for the synthesis of Py-POP; (b) route for the preparation of PPOPs-SO3H. | |
Pala's group reported a hydroxyl-rich porphyrin-based POP (PPOPs-OH) via the Alder–Longo method using 2,6-dihydroxynaphthalene-1,5-dicarbaldehyde (abbreviated as DHNDA) as the building blocks (Fig. 3b).59 The simultaneous introduction of a hydroxyl group endowed the system with reactivity with chlorosulfonic acid to obtain a sulfonic-acid-functionalized porous polymer (PPOPs-SO3H). The sulfonation could significantly increase the electronegativity and hydrophilicity of the porous skeleton. As a result, PPOPs-SO3H displayed a superb high adsorption capacity toward methylene blue (MB) from water (980.4 mg g−1). Additionally, PPOPs-SO3H also presented an excellent selectivity for MB in the co-presence of methyl orange (MO) and rhodamine B (RhB). Noteworthily, recycling experiments revealed the absorption properties of PPOPs-SO3H could be finely retained after several cycles.
3.1.4 Heavy metal removal. Heavy metal pollution is now widely regarded as one of the most pressing environmental and health problems to modern society, continuously affecting our lives for decades. As one of the most famous toxic heavy metals, Hg2+, with a special binding capacity with proteins, can induce serious damage to the nervous system and kidneys, thus threatening public health.60 Thereby, there is an urgent need to exploit low-cost but highly efficient technologies to realize the selective capture of Hg2+ from aqueous solution.61 It is well known that sulfur-bearing functional groups, such as thioether (–S–R), dithioglycol (HS–CH2CH2–SH), and sulfhydryl (–SH), can coordinate strongly with Hg2+, and so have been demonstrated to be especially useful for capturing Hg2+ from water.62Huang's group developed three thioether-functionalized porphyrin-based POPs, denoted as TPP1, TPP2, and TPP3, constructed respectively by “bottom-up” and “top-down” strategies, and were then used for capturing Hg2+ from aqueous solution (Fig. 4).63 TPP1, constructed by the “bottom-up” strategy, was obtained via the one-step polycondensation of 2,5-bis(methylthio)terephthalaldehyde (BMTA) with pyrrole. TPP1 featured a high BET surface area (554 m2 g−1) and pore volume (0.32 cm3 g−1), and sulfur content (16.8%), which enabled a high Hg2+ capture (913 mg g−1) and removal efficiency (>99%), concurrently. By contrast, TPP2 and TPP-3 obtained according to the “top-down” strategy were fabricated via a post thioether modification of as-synthesized porphyrin-based POPs. Compared with TPP1, TPP2 possessed a much lower SBET, Vtotal, as well as sulfur content. As a result, TPP2 obtained via the post-functionalized method showed a much lower Hg2+ capture efficiency (555 mg g−1 and 609 mg g−1). This work demonstrated the advantage of the bottom-up strategy for the highly efficient construction of thioether-functionalized POPs, which provides guidance for the rational construction of other thioether-functionalized POPs for specific applications.
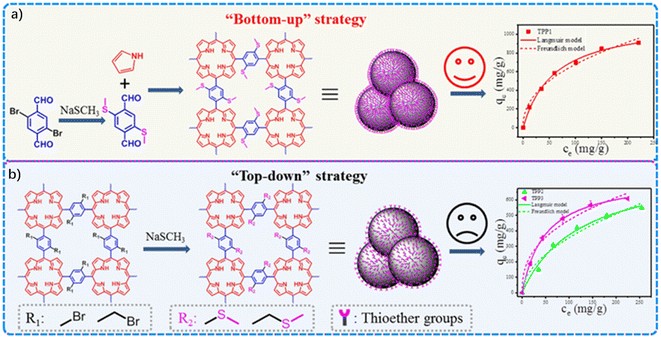 |
| Fig. 4 Synthetic procedures for thioether-functionalized porphyrin-based polymers: (a) TPP1 according to a “bottom-up” strategy; (b) TPP2 and TPP3 according to a “top-down” strategy. | |
3.1.5 Selective recovery of gold. Gold is one of the most representative precious metals, with the inherent characteristics of superb electrical conductivity, outstanding corrosion resistance, and ductility, which has seen it commonly applied in sophisticated instruments and equipment.64,65 The wide-scale usage of gold is heavily hindered by the complex mining and smelting process required.66 However, with the continuous development of science and technology, the demand is ever rising and solutions are being investigated.67To effectively solve these issues, Zhang's group developed cationic porphyrin-based porous organic polymers (Imi-PPOPs-Br) to retrieve gold from electronic waste.68 As shown in Fig. 5, Imi-PPOPs-Br possessing porphyrin and cationic imidazolium, simultaneously, was prepared via an Alder–Longo synthesis method using pyrrole and imidazolium-functionalized trialdehydes as the reaction monomers. Imi-PPOPs-Br presented a high Au(III) adsorption capacity of 1543 mg g−1, together with super reusability. Noteworthily, owing to the strong electrostatic interaction between the cationic porous skeleton and the AuCl4− anions, Imi-PPOPs-Br presented a specific selectivity for >80% removal efficiency in a solution containing various interfering metal ions [Co(II), Cr(VI), Ni(II), Hg(II), and Cd(II)]. These results revealed that Imi-PPOPs-Br could be used as an absorbent toward the highly efficient and selective recovery of gold.
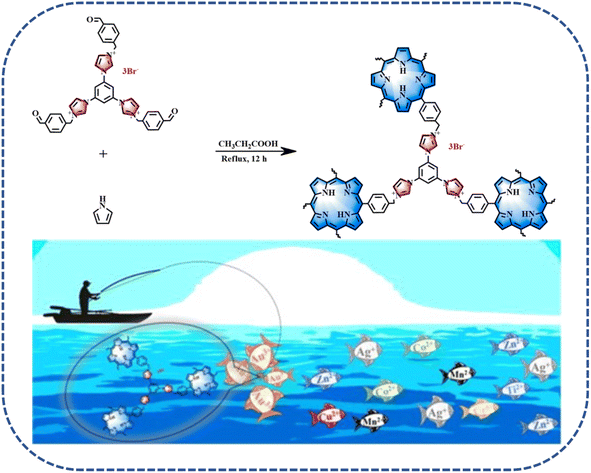 |
| Fig. 5 Schematic route for the preparation of Imi-PPOPs-Br. | |
3.2 Synthetic organic chemistry
The unique backbone and the tunable metal centers endow porphyrin-based POPs with excellent photoelectric properties.69 Easy accessible heteroatom doping, metallization, and ion functionalization can enable the π-electron conjugated structure, band gap, as well as the active groups to be easily tuned by changing the type of central metal.70,71 Hitherto, various reports have demonstrated the use of POPs in the synthesis of heterogeneous catalysts based on POPs. Porphyrin-based POPs are used in a wide range of catalytic related applications, such as chemical catalysis, photocatalysis, electrocatalysis, and enzyme catalysis.72–74 The POP-based catalyst can be recovered by simple cleaning, and the catalyst can be reused, maintaining a very high catalytic activity.
3.2.1 Carbon–carbon cross-coupling. As one of most useful transformations in organic synthesis, carbon–carbon cross-coupling reactions have aroused wide research interests in both the academic and industrial fields.75 With years of development, large amounts of catalysts with excellent activity, good selectivity, as well as a high turnover frequency have been developed, including homogeneous and heterogeneous catalysts bearing well-defined active sites.76,77 However, homogeneous catalysts are hindered by various issues, including a high production cost, poor recyclability, as well as serious metal leaching, which greatly restrict their industrial applications.78 By contrast, heterogeneous catalysts, especially those with an inherent porous structure, are regarded as catalysts with the most potential for highly efficient and recyclable organic catalysis.79 Their highly porous structures can not only realize the maximum exposure of catalytic sites, but also provide sufficient access for mass transfer.80 The structural designability and adjustable pores enable a high catalytic conversion selectivity and are beneficial for the smooth diffusion of substances and the timely release of products.Wu's group reported a salen-porphyrin-based conjugated microporous polymer (SP-CMP) obtained via the polycondensation of pyrrole and a salen-dialdehyde derivative through the Alder–Longo method.81 As displayed in Fig. 6a, SP-CMP featured not only excellent chemical and thermal stability, but also a high BET specific surface area and permanent porosity, which allow it to be used as a carrier for the ordered encapsulation of Pd-based nanoparticles via the combination of coordinate interactions and reduction with NaBH4. The as-synthesized composite, denoted as Pd@SP-CMP, showed remarkable catalytic activity toward the Suzuki–Miyaura and Heck–Mizoroki coupling reactions. Apart from the excellent catalytic selectivity, the as-synthesized samples also presented outstanding stability and recyclability, with no loss in activity over 10 continuous reactions.
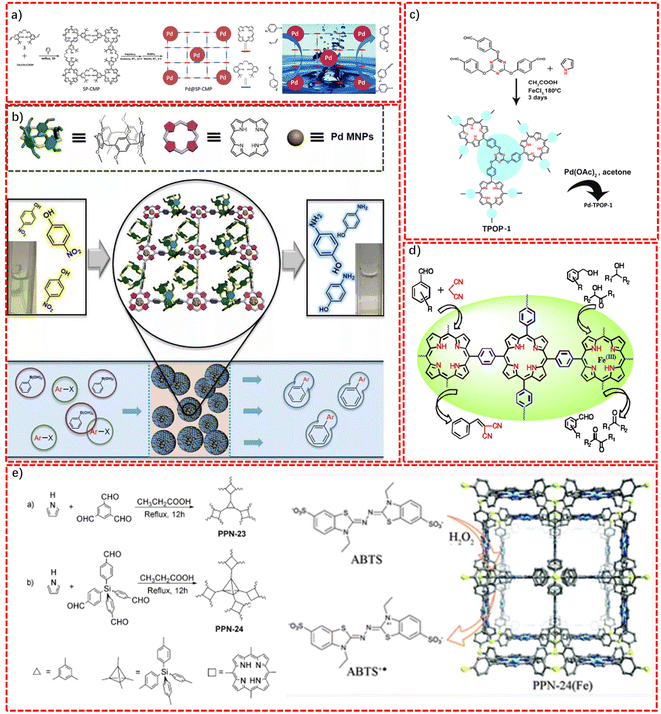 |
| Fig. 6 Porphyrin-based POP or POP composites used in synthetic organic chemistry. (a) Typical route for the synthesis of Pd@SP-CMP; (b) schematic route for the preparation of Pd@DMP[5]-TPP-CMP; (c) route for the synthesis of Pd-TPOP-1; (d) typical procedure for the synthesis of Fe-POP-1; (e) route for the synthesis of PPN-23 and PPN-24. | |
Recently, Yang's group reported porphyrin-based conjugated macrocycle polymers (CMPs) via the Alder–Longo method, using aldehyde-modified pillar[n]arenes (pillarenes) as the building blocks (Fig. 6b).82 The final product, denoted as DMP[5]-TPP-CMP, integrated the macrocyclic confinement effect and porphyrin coordination ability, simultaneously, and could realize the implantation of metal nanoparticles with a confined size and high dispersion. The Pd-nanoparticles-encapsulated composite Pd@DMP[5]-TPP-CMP, abbreviated as Pd@CMP, displayed not only remarkable catalytic activity, but also superb recyclability without deactivation in the Suzuki–Miyaura coupling (SMC) reduction over at least 5 cycles, exceeding the capability of most the reported palladium supports. Significantly, the introduction of a supramolecular chemistry macrocycle significantly enriches the family of CMPs.
Asim Bhaumik's group reported a triazine-functionalized porphyrin-based porous organic polymer (TPOP-1) featuring a hierarchical porous structure, which could be used as the ideal support for the encapsulation of Pd nanoparticles.83 TPOP-1 was manufactured via a one-pot Alder–Longo method synthesis, using 4,4′,4′′-(1,3,5-triazine-2,4,6-triyl)tris(oxy)tribenzaldehyde and pyrrole as the reaction monomers in the presence of FeCl3 (Fig. 6c). The decoration of palladium nanoparticles in the TPOP-1 endowed the Pd-TPOP-1 complex with high catalytic activity for the Sonogashira cross-coupling of aryl alkynes with aryl halides forming C–C bonds.
3.2.2 Mimicking biological oxidation and Knoevenagel condensation reactions. The most prominent feature of metalloporphyrins is their enzyme-like catalytic center with cytochrome P-450 enzyme, which can catalyze the oxidation of a series of substances.84 Inspired by this, an Fe–porphyrin-based microporous organic polymer (Fe-POP-1) with a high BET surface area was developed by Asim Bhaumik's group to mimic biological oxidation pathways.85 Different with previous reports, Fe-POP-1 featured both iron-free porphyrin and Fe(III)-containing porphyrin sites over the porous skeleton (Fig. 6d). The concurrent existence of the two kinds of porphyrin moieties renders Fe-POP-1 with bifunctional catalytic activity, in which the iron-free porphyrin moieties could catalyze the Knoevenagel condensation at room temperature, and the Fe(III)-bound sites could selectively catalyze the oxidation of alcohols to the corresponding aldehydes or ketones using TBHP as oxidants. Noteworthily, Fe-POP-1 also possessed a concurrently super reusability and excellent selectivity, which could enable it to serve as a promising bifunctional heterogeneous catalyst for the highly selective production of fine organic chemicals. Subsequently, Asim Bhaumik's group also realized a green and environmentally friendly production of 2,5-furandicarboxylic acid (FDCA) using Fe-POP-1 as the catalyst and oxygen as the oxidant.86Zhou's group synthesized two stable porphyrin-based porous organic polymers (PPN-23 and PPN-24), through the direct aromatic substitution of pyrrole with monomers bearing multiple aldehydes (Fig. 6e).87 The porphyrin center could be used as the coordination sites for the accurate anchoring of Fe, for mimicking biocatalysis. PPN-24 (Fe) could act as a high efficiency biomimetic catalyst for realizing the oxidation reaction of 2,2′-azino-bis(3-ethylbenzthiazoline-6-sulfonic acid) (ABTS) in the presence of a low concentration of hydrogen peroxide (H2O2).
3.3 Bacterial detection and elimination
Similar to environmental pollution, infection caused by bacteria also poses a great threat to public health.88 Meanwhile, a large proportion of diseases are related to bacterial infection, and have always been leading causes of human death, especially in developing and underdeveloped countries.89 At present, the main clinical therapeutic option for bacterial infections is antibiotic therapy.90 However, the appearance of drug-resistant bacteria greatly discounts the effect of antibiotics.91 Meanwhile, the overuse of antibiotics could also cause serious environmental pollution.92 Thereby, to combat bacterial infection, antibiotic-free multifunctional antibacterial materials, especially those obtained via low-cost and simple preparation methods are highly desired.
Zhang's group developed an Fe–porphyrin-based porous organic polymer, denoted as FePPOPBFPB. The FePPOPBFPB with a 3D interconnected porous structure was prepared via the Alder–Longo method through the copolymerization of pyrrole with a C-centric tetrahedral-structured multiple aldehydes monomer, namely, 4-{2,2-bis[(4-formylphenoxy)methyl]-3-(4-formylphenoxy)propoxy} benzaldehyde (BFPB) (Fig. 7).93 The as-synthesized POP featured a high specific surface area, and plentiful metal-based catalytic active sites. The introduction of a flexible alkyl chain structure significantly enhanced the light-absorption capability in the near-infrared region (NIR), which could effectively convert light energy into heat. Meanwhile, the introduction of Fe endowed the system with a peroxidase-like activity. FePPOPBFPB amalgamated enzyme catalysis with photothermal activity, which could effectively catalyze the decomposition of low concentrations of H2O2 into highly toxic hydroxyl radicals (˙OH) under NIR irradiation. As a result, FePPOPBFPB demonstrated a conspicuous bactericidal performance against S. aureus under NIR laser irradiation.
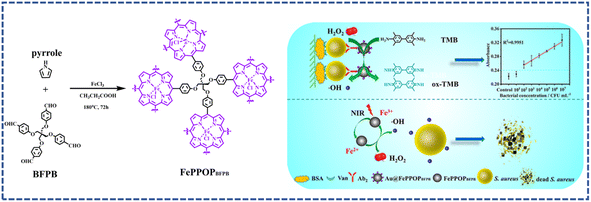 |
| Fig. 7 Fe–porphyrin-based POP for NIR-induced antibacterial applications. | |
3.4 Colorimetric detection of H2O2 and glucose
Zhang's group also reported a sulfonated metal-based porous organic polymer (FePPOPs-SO3H) synthesized via a combination of the Alder–Longo method and sulfonation, which was intended to mimic the function of natural enzymes (Fig. 8).94 The sulfonation rendered FePPOPs-SO3H with fine water dispersity, and it displayed remarkable peroxidase-mimicking performance, outperforming the normal nano Fe3O4 and ferric porphyrin monomer. FePPOPs-SO3H could also be applied as a colorimetric sensor for the ultrafast detection of H2O2 and glucose.
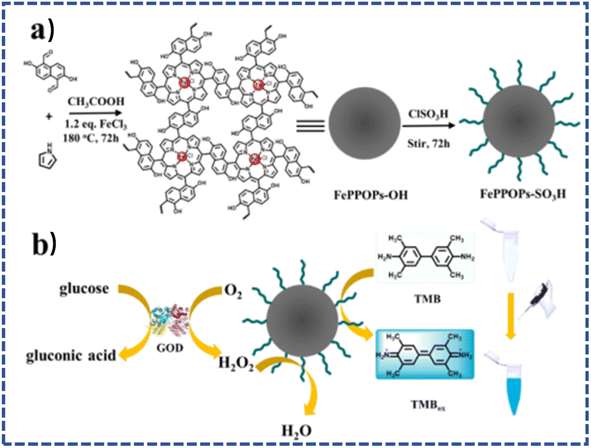 |
| Fig. 8 (a) Typical route for the synthesis of FePPOPs-SO3H and (b) schematic mechanism for the colorimetric detection of H2O2 and glucose. | |
3.5 Energy field
The depletion of consumable resources, such as coal and oil, poses a great challenge to the development of economical societies.95 To meet the ever-growing demand for a safe and stable energy supply, green and sustainable energy supply and storage are desired, such as rechargeable Zn–air batteries, Li–S batteries, as well as supercapacitors.96–98 However, the development of new energy-storage technology requires the support of materials science, for which stable and highly efficient catalysts are highly desired.99 POPs with customizable structures provide tunable surface properties in terms of specific surface area, pore size, and functionality, which are especially important for electrocatalysis.
Porphyrin-based porous organic polymers with a tunable skeleton structure and metal sites, as well as inherent rigid porous make porphyrin-based POPs, are ideal materials for the energy field. On the one hand, the tunable metal–N4 sites endow the porphyrin with redox activity, which could enable it to be used directly as an electrocatalyst.100 On the other hand, porphyrin-based POPs could also be applied as precursors for the targeted preparation of metal-based catalysts with well-defined heteroatoms doping, whose porous skeleton could be well-retained after pyrolysis.101 These features highlight porphyrin-based POPs together with their derivative porphyrin-based POPs as very promising candidates for electrocatalysis. Hitherto, porphyrin-based POPs have demonstrated infinite application potentials in various energy fields, especially in current hot spot fields, ranging from energy-related storage technologies to industry production as sustainable heterogeneous mono/bi/or even multiple functional catalysts, including for the oxygen evolution reaction (OER), the oxygen reduction reaction (ORR), and the CO2 reduction reaction (CO2RR), which are key reactions commonly involved in renewable energy systems, such as rechargeable Zn–air batteries and water-splitting devices.102
Zinc–air batteries have attracted much attention due to their high energy density, safety, and environmental friendliness.103 However, the sluggish reaction kinetics in the two key processes, i.e., the oxygen precipitation reaction (OER) and oxygen reduction reaction (ORR), greatly limit their charging and discharging efficiencies during charging and discharging processes.104
Zhou's group developed a N-doped porous carbon/Co3Fe7 alloy composite, denoted as FP-950, via the direct pyrolysis of a low-cost and readily available bimetallic conjugated microporous polymer (CMP), namely Fc–Por. Different with previous reports, Fc–Por, composed of ferrocene (Fc) and Co–porphyrin (Co–Por), was manufactured through the copolymerization of pyrrole and ferrocenedicarboxy aldehyde in the presence of cobaltous acetate, which contained two kinds of metal elements, simultaneously.105 As seen from Fig. 9a, the unique polymer structure facilitated the homogeneous distribution of metal elements throughout the skeleton, ensuring the formation of a carbon encapsulation structure of nanohybrids after carbonization. The special structure significantly promoted the synergistic effect of Co3Fe7 and Co5.47N, notably protecting the as-formed nanoparticles from harsh environments (acidic and alkaline). Moreover, the porous skeleton could be well-retained after carbonization. As a result, FP-950 also featured not only ultrahigh specific surface areas, but also a hierarchical pore structure, which is beneficial for fully exposing the catalytic active sites. Hence, FP-950 displayed robust and efficient trifunctional electrocatalytic activities toward the ORR, OER, and HER concurrently in alkaline media. Thereby, FP-950 could not only be applied as a catalyst for rechargeable Zn–air batteries, but also a highly efficient catalyst for overall water splitting realized in a self-powered manner. This work paves an easy accessible pathway for the rational preparation of low-cost multifunctional electrocatalysts for sustainable energy technologies.
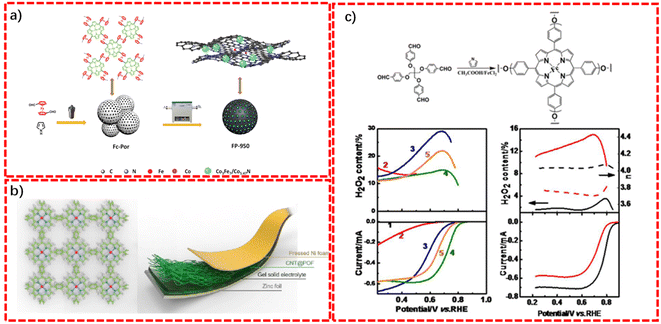 |
| Fig. 9 Porphyrin-based porous organic polymers and their corresponding derivatives for sustainable energy applications. (a) Route for the synthesis of Fc–Por and corresponding carbonized derivatives FP-950; (b) schematic route for the synthesis of CNT@POF; (c) schematic route for the synthesis of HBY–COF and HBY–COF-900. | |
Zhang's group reported a porphyrin-based POP-coated carbon nanotube (CNT) composite (CNT@POF) via the Alder–Longo method using an acid-catalyzed dehydration reaction between benzene-1,4-dialdehyde (BDA) and pyrrole in the presence of Co(CH3COO)2·4H20, in which the CNT was used as the template for enhancing the conductivity of the composite (Fig. 9b).106 The combination of POP with CNT yielded a conductive scaffold with satisfactory mechanical strength and excellent chemical stability, simultaneously, which could be directly used as a free-standing flexible film with ultrahigh durability. The periodic distribution of Co–porphyrin-based active sites in the robust and flexible film make the CNT@POF hybrid an ideal material for flexible Zn–air batteries. Specifically, a liquid Zn–air battery using the as-synthesized CNT@POF as the cathode presented a small voltage gap (0.71 V). Meanwhile, a CNT@POF based flexible all-solid-state Zn–air battery also demonstrated an outstanding recycle stability with negligible performance decay for consecutive 200 cycles.
Ren et al. fabricated an Fe-encapsulated nitrogen-doped porous carbonaceous material (HBY–COF-900) with highly effective oxygen reduction electrocatalytic activity, which was derived from the carbonation of an Fe–porphyrin-based porous organic polymer (HBY–COF) at 900 °C (Fig. 9c). HBY–COF was fabricated via the copolymerization of tetrakis(4-formyloxymethylene)methane and pyrrole using FeCl2·4H2O as the metal source.107 HBY–COF-900, with abundant FeN4 active sites, presented remarkable ORR performance and running stability in acidic media, which was even better than that of the benchmark 20% Pt/C.
3.6 Biomedical science
The development of POP-based functional materials for anti-infection applications has attracted considerable attention.108 Compared with other antibacterial materials, POP-based antibacterial materials have incomparable advantages.109 POPs feature a structural composition similar to biological macromolecules, which ensure the high biosafety of POPs.109 The electronic structure can be accurately regulated by adjusting the construction units. The chemical environment around the active site can be accurately adjusted at the molecular or even atomic level.110 The conjugated system gives it unique photoelectric properties, which can reduce the band gap and enhance the light absorption.111 Therefore, POPs provide a platform to integrate structural and performance controllability, which is conducive to the design and synthesis of therapeutic agents for different applications, and for investigating the relationship between the structure and function in-depth and the source of performance differences.112
It is worth noting that porphyrin-containing POPs could also be applied as biomedical agents in healthcare, including for drug delivery, phototherapy, chemical dynamic therapy, and enzyme therapy in diverse O2 and H2O2 related biological processes, especially cancer and bacterial treatment, realizing single mode or combination therapy.113 Their activities could be finely tuned via regulating the surrounding chemical environment of the porphyrin units. Thereby, tremendous interest has been shown in the specific preparation of porphyrin-based POPs.
3.6.1 Antibacterial therapy. Bacterial infections are one of the major threats to human health and one of the leading causes of death in both developing and less developed countries.114 There is a wide variety of bacteria in water, food, and the environment, which are difficult to see with the naked eye but can cause serious damage to life and health.115,116Zhou's group reported a multifunctional porous polymer (Fc–PP–POP) bearing repetitive porphyrin (PP) and ferrocene (Fc) unites, concurrently (Fig. 10). The Fc–PP–POP was facilely fabricated via the simple but highly efficient Alder–Longo method through the direct polymerization of ferrocene dialdehyde with fresh distilled pyrrole.117 Fc–PP–POP featured a fully conjugated structure, which combined the properties of porphyrin (photoactivity) and ferrocene (enzymatic activity), concurrently, and could be used as a broad-spectrum material-based antibacterial agent, realizing synergistic photothermal and enzyme-catalyzed therapy. On the one hand, Fc–PP–POP could convert light energy into local thermal for photothermal therapy (PTT). On the other hand, Fc–PP–POP could transform low-concentration hydrogen peroxide into highly toxic ˙OH for enzymatic therapy. Noteworthily, as a temperature-dependent catalytic reaction, the enzyme activity could be significantly enhanced via a local rise of the temperature. Thereby, Fc–PP–POP could exert self-enhanced synergistic antimicrobial therapy. Also, Fc–PP–POP featured excellent biosafety, which could also significantly accelerate the recovery of open wounds with bacterial infection. Meanwhile, Fc–PP–POP could effectively capture iodine from water, which indicates it could be applied as an advanced environmental remediation material.
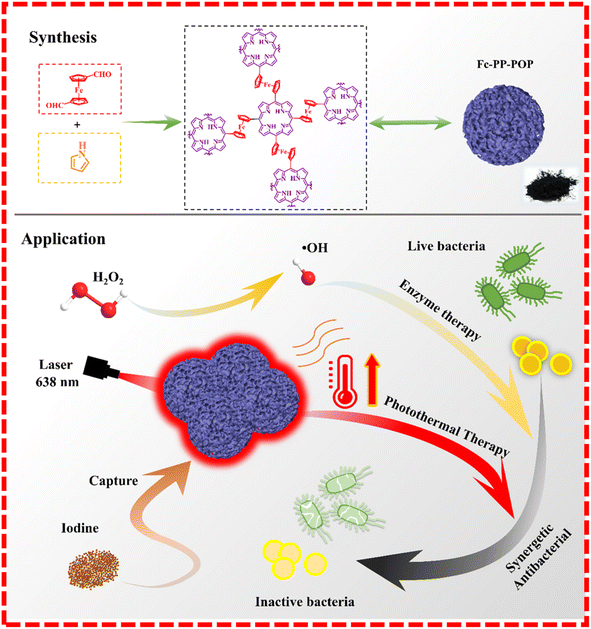 |
| Fig. 10 Schematic route for the synthesis of Fc–PP–POPs as environmental remediation materials for self-enhanced synergetic photothermal-enzymatic antibacterial activity and reversible iodine capture. | |
3.6.2 Cancer therapy. Enzymatic therapy exerts therapeutic effects through enzymic catalytic critical biochemical reactions and is regarded as one of the most methods with the greatest potential for disease treatment, which could make full use of the characteristics of the microenvironments at lesion sites to produce curative effects in the body, especially for various cancer therapies.118 However, natural enzymes face issues with their low stability, whereby the catalytic activity can be easily diminished in external environmental conditions.119 Furthermore, natural enzymes are commonly protein extracts, which are seriously hindered by their high cost and manufacturing difficulties.120 Therefore, the real application of enzymatic therapy is heavily blocked by natural enzymes.To solve this issue, Zhou's group developed an artificial nanoenzyme, denoted as HF-900, which was obtained via the direct pyrolysis of a porphyrin-based POP (HF-POP). HF-POP was prepared via the Alder–Longo method using pyrrole and hexaldehyde-phenylcyclotriphosphonitrile as the reaction monomers in the presence of FeCl2.121 The direct carbonization of the porphyrin-POP could be used as a plausible way to improve the activity of nanozymes (Fig. 11). HF-POP featured a hierarchical porous structure, which contained Fe2P and FeNx sites, and simultaneously presented prominent peroxidase-like mimicking activity, converting excessive H2O2 in the tumor microenvironment into toxic ˙OH, thereby enhancing oxidative stress in solid tumors, and thereby effectively inhibiting the proliferation and development of cancer cells. It is worth noting that HF-900 could also function as a porous carrier for the encapsulation of doxorubicin (DOX). Accordingly, the HF-900-DOX composite realized the amalgamation of an enzyme and chemotherapy for cancer, significantly enhancing the therapeutic effects. This work opens up a new direction for the development and application of POPs for future cancer treatments.
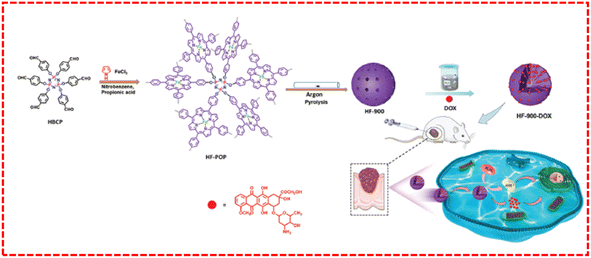 |
| Fig. 11 Schematic route for the synthesis of HF-POP, as well corresponding derivatives for cancer joint therapy. | |
4. Challenges and opportunities for future development
The Alder–Longo method arguably is a straightforward method for the preparation of functional porphyrin-based POPs, which are experiencing rapid development. Hitherto, great achievements have been made in the preparation and application of this intriguing class of materials toward diverse application areas, ranging from environmental remediation to biomedical fields. However, to use porphyrin-based POPs rationally for various applications, there are still numerous opportunities and challenges that lie ahead.
Initially, the one-pot Alder–Longo method has issues around the formation of incomplete porphyrin units during the polymerization process, which led to actual structures differing greatly from the ideal structure. Therefore, the designs of porphyrin-based skeleton structure are much more difficult to control. Second, different with ordered covalent organic frameworks (COFs), porphyrin-based POPs from the Alder–Longo method are noncrystalline materials with nonuniform pores, which are typically somewhat ill-defined. Next, the metallization is hard to be guaranteed as 100%, which induces performance differences. Thereby, the real behaviors in enzyme systems and various catalysis reactions are commonly much more difficult to understand. Third, confusion around the skeleton structure is very high, which leads to difficulties in the accurately characterization of the structure, including in solid-state 13C NMR, and Fourier transform infrared spectroscopy (FTIR). Then, it is always a huge challenge to effectively control the particle size or the morphology of porphyrin-based POPs. Furthermore, the shaping and processing problems have also severely hindered the development of this series of non-soluble powdery materials. Finally, the solvents adopted for the synthesis are usually propionic acid and nitrobenzene, which are not only irritating, but also toxic; hence, it is necessary to develop a new solvent system.
5. Conclusion
This review highlights the recent developments in the design and synthesis of porphyrin-based POPs from the direct cyclic tetramerization of pyrrole with polyaldehyde monomers through the Alder–Longo method, avoiding the preparation of high-cost porphyrin monomers. Moreover, specific sustainable applications of these materials have also been introduced in different fields, such as industrial catalysts, and therapeutic agents for drug-resistant diseases, as well as key components in energy-storage and -conversion devices. There are also specific applications in pollution treatment, such as CO2 uptake and conversion, the selective adsorption and photocatalytic degradation of cationic dyes, and heavy metal removal, as well as selective recovery of gold. The potential application of these series of functionalized POPs for different catalytic reactions were summarized in the report, namely, carbon–carbon cross-coupling, and Knoevenagel condensation reactions, as well as mimicking biological oxidation for the production of value-added products. The application of metal-coordinated porphyrin-based POPs for chemical sensing, bacterial detection, and elimination were also discussed. Furthermore, the application of these materials as therapeutic agents for cancer and bacterial infection therapy were also highlighted. Finally, we offered our perspectives on the challenge and opportunities for the future development of porphyrin-based POPs obtained from the Alder–Longo method. We strongly believe that our review could inspire further additional research in the near future to develop environmental friendly and economically viable porphyrin-based POPs with multiple functions toward advanced applications.
Conflicts of interest
There are no conflicts to declare.
Acknowledgements
This work was supported by the National Natural Science Foundation of China (22202155), the Natural Science Foundation of Shandong Province (ZR2021QB092, ZR2020QB067), and the Science and Technology Support Plan for Youth Innovation in Universities of Shandong Province (2022KJ264).
References
- D. H. Yang, Y. Tao, X. Ding and B. H. Han, Porous organic polymers for electrocatalysis, Chem. Soc. Rev., 2022, 51(2), 761–791 RSC.
- S. Wang, H. Li, H. Huang, X. Cao, X. Chen and D. Cao, Porous organic polymers as a platform for sensing applications, Chem. Soc. Rev., 2022, 51(6), 2031–2080 RSC.
- M. G. Mohamed, A. F. EL-Mahdy, M. G. Kotp and S. W. Kuo, Advances in porous organic polymers: Syntheses, structures, and diverse applications, Mater. Adv., 2022, 3(2), 707–733 RSC.
- W. Chen, P. Chen, G. Zhang, G. Xing, Y. Feng, Y. W. Yang and L. Chen, Macrocycle-derived hierarchical porous organic polymers: synthesis and applications, Chem. Soc. Rev., 2021, 50(20), 11684–11714 RSC.
- W. Wang, M. Zhou and D. Yuan, Carbon dioxide capture in amorphous porous organic polymers, J. Mater. Chem. A, 2017, 5(4), 1334–1347 RSC.
- Q. Sun, B. Aguila, Y. Song and S. Ma, Tailored porous organic polymers for task-specific water purification, Acc. Chem. Res., 2020, 53(4), 812–821 CrossRef CAS.
- S. Fajal, S. Dutta and S. K. Ghosh, Porous organic polymers (POPs) for environmental remediation, Mater. Horiz., 2023, 10, 4083–4138 RSC.
- S. Kramer, N. R. Bennedsen and S. Kegnæs, Porous organic polymers containing active metal centers as catalysts for synthetic organic chemistry, ACS Catal., 2018, 8, 6961–6982 CrossRef CAS.
- N. Singh, S. Son, J. An, I. Kim, M. Choi, N. Kong and J. S. Kim, Nanoscale porous organic polymers for drug delivery and advanced cancer theranostics, Chem. Soc. Rev., 2021, 50(23), 12883–12896 RSC.
- X. Xue, A. Lindstrom and Y. Li, Porphyrin-based nanomedicines for cancer treatment, Bioconjugate Chem., 2019, 30(6), 1585–1603 CrossRef CAS PubMed.
- T. Chatterjee, V. S. Shetti, R. Sharma and M. Ravikanth, Heteroatom-containing porphyrin analogues, Chem. Rev., 2017, 117, 3254–3328 CrossRef CAS PubMed.
- H. M. Castro-Cruz and N. A. Macias-Ruvalcaba, Porphyrin-catalyzed electrochemical hydrogen evolution reaction. Metal-centered and ligand-centered mechanisms, Coord. Chem. Rev., 2022, 458, 214430 CrossRef CAS.
- W. Ji, T. X. Wang, X. Ding, S. Lei and B. H. Han, Porphyrin-and phthalocyanine-based porous organic polymers: From synthesis to application, Coord. Chem. Rev., 2021, 439, 213875 CrossRef CAS.
- L. Jin, S. Lv, Y. Miao, D. Liu and F. Song, Recent development of porous porphyrin-based nanomaterials for photocatalysis, ChemCatChem, 2021, 13(1), 140–152 CrossRef CAS.
- L. Feng, K. Y. Wang, E. Joseph and H. C. Zhou, Catalytic porphyrin framework compounds, Trends Chem., 2020, 2(6), 555–568 CrossRef CAS.
- J. Tian, B. Huang, M. H. Nawaz and W. Zhang, Recent advances of multi-dimensional porphyrin-based functional materials in photodynamic therapy, Coord. Chem. Rev., 2020, 420, 213410 CrossRef CAS.
- Y. Wang, X. Cui, P. Zhang, Y. Wang and W. Lu, Synthesis of porphyrin porous organic polymers and their application of water pollution treatment: A review, Environ. Technol. Innovation, 2023, 29, 102972 CrossRef CAS.
- Q. Liu, H. Li, Y. Zhang, W. Chen, S. Yu and Y. Chen, Porphyrin/phthalocyanine-based porous organic polymers for pollutant removal and detection: Synthesis, mechanisms, and challenges, Environ. Res., 2023, 117406 CrossRef CAS PubMed.
- W. Ji, T. X. Wang, X. Ding, S. Lei and B. H. Han, Porphyrin-and phthalocyanine-based porous organic polymers: From synthesis to application, Coord. Chem. Rev., 2021, 439, 213875 CrossRef CAS.
- P. Kaur, J. T. Hupp and S. T. Nguyen, Porous organic polymers in catalysis: opportunities and challenges, ACS Catal., 2011, 1(7), 819–835 CrossRef CAS.
- D. Tao, L. Feng, Y. Chao, C. Liang, X. Song, H. Wang, Y. K. and Z. Liu, Covalent organic polymers based on fluorinated porphyrin as oxygen nanoshuttles for tumor hypoxia relief and enhanced photodynamic therapy, Adv. Funct. Mater., 2018, 28(43), 1804901 CrossRef.
- R. Chen, J. L. Shi, Y. Ma, G. Lin, X. Lang and C. Wang, Designed synthesis of a 2D porphyrin-based sp2 carbon-conjugated covalent organic framework for heterogeneous photocatalysis, Angew. Chem., Int. Ed., 2019, 58(19), 6430–6434 CrossRef CAS PubMed.
- G. Lin, H. Ding, R. Chen, Z. Peng, B. Wang and C. Wang, 3D porphyrin-based covalent organic frameworks, J. Am. Chem. Soc., 2017, 139(25), 8705–8709 CrossRef CAS PubMed.
- P. Schweyen, M. Hoffmann, J. Krumsieck, B. Wolfram, X. Xie and M. Bröring, Metal-Assisted One-Pot Synthesis of Isoporphyrin Complexes, Angew. Chem., Int. Ed., 2016, 55(34), 10118–10121 CrossRef CAS PubMed.
- X. Luo, J. Wan, N. Meckbach, V. Strehmel, S. Li, Z. Chen and B. Strehmel, A Porphyrin-Based Organic Network Comprising Sustainable Carbon Dots for Photopolymerization, Angew. Chem., Int. Ed., 2022, 61(40), e202208180 CrossRef CAS PubMed.
- K. M. Smith, Development of porphyrin syntheses, New J. Chem., 2016, 40(7), 5644–5649 RSC.
- A. Modak, M. Nandi, J. Mondal and A. Bhaumik, Porphyrin based porous organic polymers: novel synthetic strategy and exceptionally high CO2 adsorption capacity, Chem. Commun., 2012, 48(2), 248–250 RSC.
- R. Ding, J. Liu, T. Wang and X. Zhang, Bottom-up synthesis of cationic porphyrin-based porous organic polymers for highly efficient and selective recovery of gold, Chem. Eng. J., 2022, 449, 137758 CrossRef CAS.
- S. Liu, Z. Liu, Q. Meng, C. Chen and M. Pang, Facile synthesis of a cubic porphyrin-based covalent organic framework for combined breast cancer therapy, ACS Appl. Mater. Interfaces, 2021, 13(48), 56873–56880 CrossRef CAS.
- G. Jiangfei, W. Lizhi, D. Zhang and J. Huang, Amino-functionalized porphyrin-based porous organic polymers for CO2 capture and Hg2+ removal, Energy Fuels, 2020, 34(8), 9771–9778 CrossRef CAS.
- M. Chen, H. Li, C. Liu, J. Liu, Y. Feng, A. G. Wee and B. Zhang, Porphyrin-and porphyrinoid-based covalent organic frameworks (COFs): From design, synthesis to applications, Coord. Chem. Rev., 2021, 435, 213778 CrossRef CAS.
- W. Hao, D. Chen, Y. Li, Z. Yang, G. Xing, J. Li and L. Chen, Facile synthesis of porphyrin based covalent organic frameworks via an A2B2 monomer for highly efficient heterogeneous catalysis, Chem. Mater., 2019, 31(19), 8100–8105 CrossRef CAS.
- B. Zhou, L. Liu, Z. Yang, X. Li, Z. Wen and L. Chen, Porous organic polymer gel derived electrocatalysts for efficient oxygen reduction, ChemElectroChem, 2019, 6(2), 485–492 CrossRef CAS.
- S. Kumar, M. Y. Wani, C. T. Arranja, J. D. A. e Silva, B. Avula and A. J. Sobral, Porphyrins as nanoreactors in the carbon dioxide capture and conversion: a review, J. Mater. Chem. A, 2015, 3(39), 19615–19637 RSC.
- Z. Su, J. Li, X. Bai, J. Wei, Y. Xu, Z. Zhang, N. Wang and J. Li, Synthesis of Mn (III)–porphyrin porous coordination polymers as heterogeneous catalysts for CO2 cycloaddition reaction, Appl. Organomet. Chem., 2021, 35(7), e6228 CrossRef CAS.
- D. Guo, C. Li, J. Zhang, G. Liu, X. Luo and F. Wu, Metalloporphyrin-based porous organic polymer as an efficient catalyst for cycloaddition of epoxides and CO2, J. Solid State Chem., 2021, 293, 121770 CrossRef CAS.
- X. Yao, K. Chen, L. Q. Qiu, Z. W. Yang and L. N. He, Ferric porphyrin-based porous organic polymers for CO2 photocatalytic reduction to syngas with selectivity control, Chem. Mater., 2021, 33(22), 8863–8872 CrossRef CAS.
- S. Lin, C. S. Diercks, Y. B. Zhang, N. Kornienko, E. M. Nichols, Y. Zhao, A. R. Paris, D. Kim, P. Yang, O. M. Yaghi and C. J. Chang, Covalent organic frameworks comprising cobalt porphyrins for catalytic CO2 reduction in water, Science, 2015, 349(6253), 1208–1213 CrossRef CAS PubMed.
- W. Liu, X. Li, C. Wang, H. Pan, W. Liu, K. Wang, Q. Zeng, R. Wang and J. Jiang, A scalable general synthetic approach toward ultrathin imine-linked two-dimensional covalent organic framework nanosheets for photocatalytic CO2 reduction, J. Am. Chem. Soc., 2019, 141(43), 17431–17440 CrossRef CAS PubMed.
- C. Y. Lin, D. Zhang, Z. Zhao and Z. Xia, Covalent organic framework electrocatalysts for clean energy conversion, Adv. Mater., 2018, 30(5), 1703646 CrossRef PubMed.
- J. Yan, B. Zhang, S. Guo and Z. Wang, Porphyrin-based nanoporous organic polymers for adsorption of carbon dioxide, ethane, and methane, ACS Appl. Nano Mater., 2021, 4(10), 10565–10574 CrossRef CAS.
- G. Wang, J. Chen, Y. Ding, P. Cai, L. Yi, Y. Li, C. Tu, Y. Hou, Z. Wen and L. Dai, Electrocatalysis for CO 2 conversion: from fundamentals to value-added products, Chem. Soc. Rev., 2021, 50(8), 4993–5061 RSC.
- Y. Quan, J. Zhu and G. Zheng, Electrocatalytic reactions for converting CO2 to value-added products, Small Sci., 2021, 1(10), 2100043 CrossRef CAS.
- M. M. Zain and A. R. Mohamed, An overview on conversion technologies to produce value added products from CH4 and CO2 as major biogas constituents, Renewable Sustainable Energy Rev., 2018, 98, 56–63 CrossRef CAS.
- N. Yusuf, F. Almomani and H. Qiblawey, Catalytic CO2 conversion to C1 value-added products: Review on latest catalytic and process developments, Fuel, 2023, 345, 128178 CrossRef CAS.
- X. Liu, F. Zhou, M. Chen, W. Xu, H. Liu, J. Zhong and R. Luo, Synergistically Converting Carbon Dioxide into Cyclic Carbonates by Metalloporphyrin-Based Cationic Polymers with Imidazolium Functionality, ChemistrySelect, 2021, 6(4), 583–588 CrossRef CAS.
- S. Jayakumar, H. Li, L. Tao, C. Li, L. Liu, J. Chen and Q. Yang, Cationic Zn-porphyrin immobilized in mesoporous silicas as bifunctional catalyst for CO2 cycloaddition reaction under cocatalyst free conditions, ACS Sustain. Chem. Eng., 2018, 6(7), 9237–9245 CrossRef CAS.
- X. Zhang, J. Wang, Y. Bian, H. Lv, B. Qiu, Y. Zhang, R. Qin, D. Zhu, S. Zhang, D. Li, S. Wang, W. Mai, Y. Li and T. Li, A novel conjugated microporous polymer microspheres comprising cobalt porphyrins for efficient catalytic CO2 cycloaddition under ambient conditions, J. CO2 Util., 2022, 58, 101924 CrossRef CAS.
- J. Chen, M. Zhong, L. Tao, L. Liu, S. Jayakumar, C. Li, H. Li and Q. Yang, The cooperation of porphyrin-based porous polymer and thermal-responsive ionic liquid for efficient CO2 cycloaddition reaction, Green Chem., 2018, 20(4), 903–911 RSC.
- L. Liu, S. Jayakumar, J. Chen, L. Tao, H. Li, Q. Yang and C. Li, Synthesis of bifunctional porphyrin polymers for catalytic conversion of dilute CO2 to cyclic carbonates, ACS Appl. Mater. Interfaces, 2021, 13(25), 29522–29531 CrossRef CAS PubMed.
- F. N. Chaudhry and M. F. Malik, Factors affecting water pollution: a review, J. Ecosyst. Ecography, 2017, 7(1), 225–231 Search PubMed.
- A. K. Dwivedi, Researches in water pollution: A review, Int. Res. J. Basic Appl. Sci., 2017, 4(1), 118–142 Search PubMed.
- M. Wang, M. Webber, B. Finlayson and J. Barnett, Rural industries and water pollution in China, J. Environ. Manage., 2008, 86(4), 648–659 CrossRef CAS PubMed.
- R. P. Schwarzenbach, T. Egli, T. B. Hofstetter, U. Von Gunten and B. Wehrli, Global water pollution and human health, Annu. Rev. Environ. Resour., 2010, 35, 109–136 CrossRef.
- P. Samanta, A. V. Desai, S. Let and S. K. Ghosh, Advanced porous materials for sensing, capture and detoxification of organic pollutants toward water remediation, ACS Sustain. Chem. Eng., 2019, 7(8), 7456–7478 CrossRef CAS.
- Y. Cheng, Y. Ying, S. Japip, S. D. Jiang, T. S. Chung, S. Zhang and D. Zhao, Advanced porous materials in mixed matrix membranes, Adv. Mater., 2018, 30(47), 1802401 CrossRef PubMed.
- S. P. Fernandes, V. Romero, B. Espiña and L. M. Salonen, Tailoring covalent organic frameworks to capture water contaminants, Chem.–Eur. J., 2019, 25(26), 6461–6473 CrossRef CAS PubMed.
- T. Liu, L. Jing, L. Cui, Q. Liu and X. Zhang, Facile one-pot synthesis of a porphyrin-based hydrophilic porous organic polymer and application as recyclable absorbent for selective separation of methylene blue, Chemosphere, 2018, 212, 1038–1046 CrossRef CAS PubMed.
- I. R. Pala and S. L. Brock, ZnS nanoparticle gels for remediation of Pb2+ and Hg2+ polluted water, ACS Appl. Mater. Interfaces, 2012, 4(4), 2160–2167 CrossRef CAS PubMed.
- W. Shen, Y. Fang, M. Azeem, Y. Gao, X. Li, P. Zhao, A. Ali, M. Li and R. Li, Chitosan crosslinked with polyamine-co-melamine for adsorption of Hg2+: Application in purification of polluted water, Int. J. Biol. Macromol., 2021, 181, 778–785 CrossRef CAS PubMed.
- A. Modak, P. Bhanja, M. Selvaraj and A. Bhaumik, Functionalized porous organic materials as efficient media for the adsorptive removal of Hg (II) ions, Environ. Sci.: Nano, 2020, 7(10), 2887–2923 RSC.
- F. Pan, C. Tong, Z. Wang, F. Xu, X. Wang, B. Weng, D. Pan and R. Zhu, Novel sulfhydryl functionalized covalent organic frameworks for ultra-trace Hg2+ removal from aqueous solution, J. Mater. Sci. Technol., 2021, 93, 89–95 CrossRef CAS.
- L. Wang, J. Wang, Y. Wang, F. Zhou and J. Huang, Thioether-functionalized porphyrin-based polymers for Hg2+ efficient removal in aqueous solution, J. Hazard. Mater., 2022, 429, 128303 CrossRef CAS PubMed.
- B. Hammer and J. K. Norskov, Why gold is the noblest of all the metals, Nature, 1995, 376(6537), 238–240 CrossRef CAS.
- C. W. Corti and R. J. Holliday, Commercial aspects of gold applications: from materials science to chemical science, Gold Bull., 2004, 37, 20–26 CrossRef CAS.
- Y. Lebsir, S. Boroviks, M. Thomaschewski, S. I. Bozhevolnyi and V. A. Zenin, Ultimate limit for optical losses in gold, revealed by quantitative near-field microscopy, Nano Lett., 2022, 22(14), 5759–5764 CrossRef CAS PubMed.
- A. G. Rad, H. Abbasi and M. H. Afzali, Gold nanoparticles: synthesising, characterizing and reviewing novel application in recent years, Phys. Procedia, 2011, 22, 203–208 CrossRef.
- R. Ding, J. Liu, T. Wang and X. Zhang, Bottom-up synthesis of cationic porphyrin-based porous organic polymers for highly efficient and selective recovery of gold, Chem. Eng. J., 2022, 449, 137758 CrossRef CAS.
- J. Ban, S. Xu and L. Pan, Novel porphyrin polymers: synthesis and photoelectric property, J. Mater. Sci., 2021, 56, 1814–1826 CrossRef.
- A. Harriman and J. P. Sauvage, A strategy for constructing photosynthetic models: porphyrin-containing modules assembled around transition metals, Chem. Soc. Rev., 1996, 25(1), 41–48 RSC.
- Q. Zha, X. Rui, T. Wei and Y. Xie, Recent advances in the design strategies for porphyrin-based coordination polymers, CrystEngComm, 2014, 16(32), 7371–7384 RSC.
- A. R. Oveisi, K. Zhang, A. Khorramabadi-Zad, O. K. Farha and J. T. Hupp, Stable and catalytically active iron porphyrin-based porous organic polymer: Activity as both a redox and Lewis acid catalyst, Sci. Rep., 2015, 5(1), 10621 CrossRef CAS PubMed.
- K. Zhang, O. K. Farha, J. T. Hupp and S. T. Nguyen, Complete double epoxidation of divinylbenzene using Mn(porphyrin)-based porous organic polymers, ACS Catal., 2015, 5(8), 4859–4866 CrossRef CAS.
- Z. Dai, Q. Sun, X. Liu, C. Bian, Q. Wu, S. Pan, L. Wang, X. Meng, F. Deng and F. S. Xiao, Metalated porous porphyrin polymers as efficient heterogeneous catalysts for cycloaddition of epoxides with CO2 under ambient conditions, J. Catal., 2016, 338, 202–209 CrossRef CAS.
- L. Xue and Z. Lin, Theoretical aspects of palladium-catalysed carbon-carbon cross-coupling reactions, Chem. Soc. Rev., 2010, 39(5), 1692–1705 RSC.
- Á. Molnár, Efficient, selective, and recyclable palladium catalysts in carbon-carbon coupling reactions, Chem. Rev., 2011, 111(3), 2251–2320 CrossRef PubMed.
- L. Yin and J. Liebscher, Carbon-carbon coupling reactions catalyzed by heterogeneous palladium catalysts, Chem. Rev., 2007, 107(1), 133–173 CrossRef CAS PubMed.
- Y. Sohtome, K. Kanomata and M. Sodeoka, Cross-coupling reactions of persistent tertiary carbon radicals, Bull. Chem. Soc. Jpn., 2021, 94(3), 1066–1079 CrossRef CAS.
- Á. Mastalir and Á. Molnár, Palladium Nanoparticles Supported on Porous Silica Materials as Heterogeneous Catalysts of C-C Coupling and Cross-Coupling Reactions, ChemCatChem, 2023, 15(19), e202300643 CrossRef.
- U. P. Patil, Porous carbon catalysis in sustainable synthesis of functional heterocycles: An overview, Chin. Chem. Lett., 2024, 109472 CrossRef CAS.
- P. Ju, S. Wu, Q. Su, X. Li, Z. Liu, G. Li and Q. Wu, Salen–porphyrin-based conjugated microporous polymer supported Pd nanoparticles: highly efficient heterogeneous catalysts for aqueous C–C coupling reactions, J. Mater. Chem. A, 2019, 7(6), 2660–2666 RSC.
- Z. Li, X. Li and Y. W. Yang, Conjugated macrocycle polymer nanoparticles with alternating pillarenes and porphyrins as struts and cyclic nodes, Small, 2019, 15(12), 1805509 CrossRef PubMed.
- A. Modak, M. Pramanik, S. Inagaki and A. Bhaumik, A triazine functionalized porous organic polymer: excellent CO2 storage material and support for designing Pd nanocatalyst for C–C cross-coupling reactions, J. Mater. Chem. A, 2014, 2(30), 11642–11650 RSC.
- F. Esteves, J. Rueff and M. Kranendonk, The central role of cytochrome P450 in xenobiotic metabolism-a brief review on a fascinating enzyme family, J. Xenobiot., 2021, 11(3), 94–114 CrossRef CAS PubMed.
- A. Modak, J. Mondal and A. Bhaumik, Porphyrin based porous organic polymer as bi-functional catalyst for selective oxidation and Knoevenagel condensation reactions, Appl. Catal., A, 2013, 459, 41–51 CrossRef CAS.
- B. Saha, D. Gupta, M. M. Abu-Omar, A. Modak and A. Bhaumik, Porphyrin-based porous organic polymer-supported iron (III) catalyst for efficient aerobic oxidation of 5-hydroxymethyl-furfural into 2, 5-furandicarboxylic acid, J. Catal., 2013, 299, 316–320 CrossRef CAS.
- L. Zou, D. Feng, T. F. Liu, Y. P. Chen, S. Fordham, S. Yuan, J. Tian and H. C. Zhou, Facile one-pot synthesis of porphyrin based porous polymer networks (PPNs) as biomimetic catalysts, Chem. Commun., 2015, 51(19), 4005–4008 RSC.
- H. Luo, W. Ji, W. Guo, P. Chen, Z. Zhang, X. Xu, B. Yue, W. Tan and B. Zhou, A photoactive Dual-cationic Covalent Organic Framework Encapsulated Sodium Nitroprusside as controllable NO-releasing material for joint cation/photothermal/NO antibacterial therapy, Microporous Mesoporous Mater., 2022, 346, 112281 CrossRef CAS.
- L. Wang, L. Shi, T. Guo, J. Yuan, B. Zhou and J. Zhang, Near-infrared active ferrocenyl porous organic polymer with photothermal enhanced enzymatic activity for combination antibacterial application, RSC Adv., 2023, 13(38), 26445–26454 RSC.
- H. Luo, T. Huang, X. Li, J. Wang, T. Lv, W. Tan, F. Gao, J. Zhang and B. Zhou, Synergistic antibacterial and wound-healing applications of an imidazole-based porous organic polymer encapsulated silver nanoparticles composite, Microporous Mesoporous Mater., 2022, 337, 111925 CrossRef CAS.
- Z. Zhang, L. Shi, L. Chu, P. Chen, P. Sun, Z. Chen, L. Wei and B. Zhou, Crown ether-based porous organic polymer encapsulated Ag2[Fe(CN)5NO] composite towards ultra-low dose efficient sterilization and wound healing application, Mater. Today Chem., 2023, 34, 101794 CrossRef CAS.
- P. Chen, R. Ze, X. Xia, Z. Zhang, K. Lu, L. Wei and B. Zhou, Composite porphyrin-based conjugated microporous polymer/graphene oxide capable of photo-triggered combinational antibacterial therapy and wound healing, Biomater. Adv., 2023, 154, 213662 CrossRef CAS PubMed.
- D. Li, Y. Fang and X. Zhang, Bacterial detection and elimination using a dual-functional porphyrin-based porous organic polymer with peroxidase-like and high near-infrared-light-enhanced antibacterial activity, ACS Appl. Mater. Interfaces, 2020, 12(8), 8989–8999 CrossRef CAS PubMed.
- T. Liu, J. Tian, L. Cui, Q. Liu, L. Wu and X. Zhang, Facile strategy to prepare a metalloporphyrin-based hydrophilic porous organic polymer with enhanced peroxidase-like activity and high stability for colorimetric detection of H2O2 and glucose, Colloids Surf., B, 2019, 178, 137–145 CrossRef CAS PubMed.
- T. Zhang, S. Mao, P. Sun, X. Gao, H. Fang, H. Luo, W. Zhang and B. Zhou, Nanosized FeS/ZnS heterojunctions derived using zeolitic imidazolate Framework-8 (ZIF-8) for pH-universal oxygen reduction and High-efficiency Zn–air battery, J. Colloid Interface Sci., 2022, 608, 446–458 CrossRef CAS PubMed.
- B. Zhou, F. Yan, X. Li, J. Zhou and W. Zhang, An interpenetrating porous organic polymer as a precursor for FeP/Fe2P-embedded porous carbon toward a pH-universal ORR catalyst, ChemSusChem, 2019, 12(4), 915–923 CrossRef CAS PubMed.
- J. Dou, M. Qi, H. Wang, W. Shi, X. Luan, W. Guo, L. Xiao, C. Zhao, D. Cheng, T. Jiang, W. Zhang, W. Bian and B. Zhou, SiO2-templated ferrocenyl porous organic polymer gel derived porous Carbon/Fe2C nanohybrids with interpenetrating macropores as oxygen reduction electrocatalyst for Zn-air batteries, Microporous Mesoporous Mater., 2021, 320, 111101 CrossRef CAS.
- B. Zhou, X. Hu, G. Zeng, S. Li, Z. Wen and L. Chen, Bottom-Up Construction of Porous Organic Frameworks with Built-In TEMPO as a Cathode for Lithium–Sulfur Batteries, ChemSusChem, 2017, 10(14), 2955–2961 CrossRef CAS PubMed.
- M. Zhang, T. Zhao, J. Dou, Z. Xu, W. Zhang, X. Chen, X. Wang and B. Zhou, Bottom-up construction of conjugated microporous polyporphyrin-coated graphene hydrogel composites with hierarchical pores for high-performance capacitors, ChemElectroChem, 2019, 6(24), 5946–5950 CrossRef CAS.
- X. Li, Q. Yao, Z. Li, H. Li, Q. L. Zhu and Z. H. Lu, Porphyrin framework-derived N-doped porous carbon-confined Ru for NH3 BH3 methanolysis: the more pyridinic-N, the better, J. Mater. Chem. A, 2022, 10(1), 326–336 RSC.
- J. Meng, Z. Xu, H. Li, D. James Young, C. Hu and Y. Yang, Porphyrin-based NiFe Porous Organic Polymer Catalysts for the Oxygen Evolution Reaction, ChemCatChem, 2021, 13(5), 1396–1402 CrossRef CAS.
- C. Li, Z. Qiu, H. Sun, Y. Yang and C. P. Li, Recent progress in covalent organic frameworks (COFs) for electrocatalysis, Chin. J. Struct. Chem., 2022, 41(11), 2211084–2211099 CAS.
- Z. Zhu, Z. Yang, Y. Fan, C. Liu, H. Sun, W. Liang and A. Li, Calcination of porphyrin-based conjugated microporous polymers nanotubes as nanoporous N-rich metal-free electrocatalysts for efficient oxygen reduction reaction, ACS Appl. Energy Mater., 2020, 3(6), 5260–5268 CrossRef CAS.
- J. Tang, Z. Liang, H. Qin, X. Liu, B. Zhai, Z. Su, Q. Liu, H. Lei, K. Liu, C. Zhao, R. Cao and Y. Fang, Large-area free-standing metalloporphyrin-based covalent organic framework films by liquid-air interfacial polymerization for oxygen electrocatalysis, Angew. Chem., Int. Ed., 2023, 135(1), e202214449 CrossRef.
- W. Guo, X. Luan, P. Sun, T. Wang, H. Luo, X. Li, C. Li, W. Tan, J. Bai, Q. Wang and B. Zhou, A porous carbon layer wrapped Co3Fe7 alloy derived from a bimetallic conjugated microporous polymer as a trifunctional electrocatalyst for rechargeable Zn-air batteries and self-powered overall water splitting, Sustainable Energy Fuels, 2021, 5(23), 6085–6096 RSC.
- B. Q. Li, S. Y. Zhang, B. Wang, Z. J. Xia, C. Tang and Q. Zhang, A porphyrin covalent organic framework cathode for flexible Zn–air batteries, Energy Environ. Sci., 2018, 11(7), 1723–1729 RSC.
- S. B. Ren, J. Wang and X. H. Xia, Highly efficient oxygen reduction electrocatalyst derived from a new three-dimensional polyporphyrin, ACS Appl. Mater. Interfaces, 2016, 8(39), 25875–25880 CrossRef CAS PubMed.
- Y. Zhu, P. Xu, X. Zhang and D. Wu, Emerging porous organic polymers for biomedical applications, Chem. Soc. Rev., 2022, 51(4), 1377–1414 RSC.
- Z. Li and Y. W. Yang, Creation and bioapplications of porous organic polymer materials, J. Mater. Chem. B, 2017, 5(47), 9278–9290 RSC.
- S. Wang, J. Zhang, L. Chu, H. Xiao, C. Miao, Z. Pan, Z. Wang and B. Zhou, Crown-ether threaded covalent organic polyrotaxane framework (COPF) towards synergistic crown/Zn2+/photothermal/photodynamic antibacterial and infected wound healing therapy, Biomater. Adv., 2024, 213814 CrossRef CAS PubMed.
- Y. Wang, N. Tang, T. Zhao, F. Gao, X. Teng, L. Xu, C. Zhao, W. Li, W. Tan and B. Zhou, Bacterial Structure-Inspired Synthesis of Tumor-Microenvironment-Activated Artificial Enzyme toward Photothermal Enhanced Chemodynamic Cancer Therapy, ChemistrySelect, 2023, 8(41), e202302303 CrossRef CAS.
- S. Bhunia, K. A. Deo and A. K. Gaharwar, 2D covalent organic frameworks for biomedical applications, Adv. Funct. Mater., 2020, 30(27), 2002046 CrossRef CAS.
- H. Lou, L. Chu, W. Zhou, J. Dou, X. Teng, W. Tan and B. Zhou, A diselenium-bridged covalent organic framework with pH/GSH/photo-triple-responsiveness for highly controlled drug release toward joint chemo/photothermal/chemodynamic cancer therapy, J. Mater. Chem. B, 2022, 10(39), 7955–7966 RSC.
- Y. Tian, Z. Ding, X. Zheng, Y. Li, X. Teng, G. Guo, J. Wang, W. Tan and B. Zhou, Porphyrin-based porous organic polymer coated ZIF-8 nanoparticles as tumor targeted photosensitizer for combination cancer photodynamic/photothermal therapy, Microporous Mesoporous Mater., 2023, 355, 112562 CrossRef CAS.
- D. M. Morens and A. S. Fauci, Emerging infectious diseases: threats to human health and global stability, PLoS Pathog., 2013, 9(7), e1003467 CrossRef CAS PubMed.
- A. J. Waldman and E. P. Balskus, The human microbiota, infectious disease, and global health: challenges and opportunities, ACS Infect. Dis., 2018, 4(1), 14–26 CrossRef CAS PubMed.
- C. Miao, L. Chu, D. Guo, X. Ding, W. Guo, S. Wang, J. Sheng, J. Zhang, Z. Wang and B. Zhou, Ferrocene-based porous polymer as advanced environmental remediation material for self-enhanced synergetic photothermal-enzymatic antibacterial and reversible iodine capture, J. Environ. Chem. Eng., 2023, 11(5), 110514 CrossRef CAS.
- Q. Wang, J. Jiang and L. Gao, Nanozyme-based medicine for enzymatic therapy: progress and challenges, Biomed. Mater., 2021, 16(4), 042002 CrossRef CAS PubMed.
- D. Wang, H. Wu, C. Wang, L. Gu, H. Chen, D. Jana, L. Feng, J. Liu, X. Wang, P. Xu, Z. Guo, Q. Chen and Y. Zhao, Self-assembled single-site nanozyme for tumorspecific amplified cascade enzymatic therapy, Biomed. Mater., 2021, 133(6), 3038–3044 Search PubMed.
- R. Zhang, X. Yan and K. Fan, Nanozymes inspired by natural enzymes, Acc. Mater. Res., 2021, 2(7), 534–547 CrossRef CAS.
- Z. Xu, T. Wang, J. Li, F. Zhang, H. Lou, J. Zhang, W. Zhang, W. Zhang and B. Zhou, Nanosized porous artificial enzyme as a pH-sensitive doxorubicin delivery system for joint enzymatic and chemotherapy towards tumor treatment, New J. Chem., 2022, 46(30), 14565–14577 RSC.
Footnote |
† These authors contributed equally to this work. |
|
This journal is © The Royal Society of Chemistry 2024 |
Click here to see how this site uses Cookies. View our privacy policy here.