DOI:
10.1039/D4RA02548B
(Paper)
RSC Adv., 2024,
14, 17498-17506
ZIF-8/g-C3N4 photocatalysts: enhancing CO2 reduction through improved adsorption and photocatalytic performance†
Received
4th April 2024
, Accepted 20th May 2024
First published on 30th May 2024
Abstract
Nowadays, the widespread concern over controlling CO2 emissions and mitigating the adverse effects of greenhouse gases on global climate has attracted significant attention. In this study, g-C3N4 was synthesized by thermopolymerizing urea. Subsequently, ZIF-8 was combined with g-C3N4 using an in situ deposition method, resulting in the fabrication of ZIF-8/g-C3N4 composite photocatalysts at various molar ratios. Effective incorporation of ZIF-8 into g-C3N4 suppressed the recombination of photogenerated electrons and holes, thereby enhancing CO2 capture capacity and preserving light absorption capabilities. The ZIF-8/g-C3N4 composite demonstrates excellent photocatalytic performance for CO2 reduction, where the optimized material exhibited a CO2 adsorption capacity 1.52 times that of pure g-C3N4 and increased the conversion of CO2 to CH4 by more than sevenfold. This study harnesses the superior CO2 adsorption properties of metal–organic frameworks to develop more efficient photocatalysts, enhancing CO2 conversion efficacy and offering insights for developing efficient photocatalysts that utilize CO2.
1 Introduction
Graphitic carbon nitride (g-C3N4) has emerged as a semiconductor material with significant potential in the realm of CO2 photocatalysis, attributed to its distinctive advantages. This non-metallic semiconductor is composed of carbon and nitrogen, elements abundantly available on Earth. It features a relatively small bandgap of approximately 2.7 eV, enabling a high responsiveness to visible light. Additionally, g-C3N4 exhibits superior resistance to acids and bases and maintains its integrity under illumination. Its structure and properties can be readily tailored, offering commendable photocatalytic activity.1 However, the prevalent issues currently include low photogenerated electron–hole separation efficiency and low CO2 absorption capacity.2 Therefore, the development of new materials or the enhancement of CO2 photocatalytic reduction through doping, compositing to form heterojunctions, and other methods, represents a current research focus. In fact, several adsorbents, such as graphene, activated carbon, zeolites, and certain mesoporous materials, have been employed in conjunction with specific photocatalysts to augment the reduction of CO2. However, the improvement achieved through these combinations is often quite limited. The catalysts currently in use commonly suffer from issues such as light shielding, photocapture of charges, occupation of redox active sites, and non-selective adsorption of reactants and products.3
Metal–organic frameworks (MOFs) are a new class of crystalline porous materials with periodic network structures, self-assembled from metal clusters or metal ions and organic ligands through coordination bonds. They hold significant promise in applications such as adsorption and catalysis. MOF materials have demonstrated strong capabilities in CO2 adsorption. Based on this, our experiment proposes that combining MOFs with semiconductor photocatalysts can significantly enhance CO2 capture performance, thereby increasing the efficiency of photocatalytic CO2 reduction.4 ZIF-8 (zeolitic imidazolate framework-8) is a type of MOF material with a zeolite topology structure formed by the coordination of Zn2+ ions with 2-methylimidazole. ZIF-8 possesses a large specific surface area, high porosity, regular pore channels, good thermal stability, water resistance, CO2 affinity, and the combined advantages of traditional zeolite molecular sieves and MOFs in terms of chemical stability. Furthermore, it has two other features: one aspect is associated with the inherent transparency of ZIF-8.5 The integration of ZIF-8 into semiconductor matrices scarcely detracts from their photonic capture capabilities. Another aspect is associated the selective adsorptive properties of ZIF-8. ZIF-8 exhibits a pronounced preference for adsorbing CO2, the primary reactant, while maintaining a low affinity towards the resultant reduction products, such as CH4 and CH3OH.6 Moreover, ZIF-8 can typically be synthesized through three common methods: synthesis in methanol solution;7 synthesis in a mixed solution of methanol and aqueous ammonia;8 and solvothermal synthesis in DMF.9 Research has found that ZIF-8(MeOH) has a larger external surface area and more acid–base sites, thus exhibiting significantly higher catalytic activity than ZIF-8(NH4OH) and ZIF-8 (DMF).10 Therefore, this study chose to synthesize ZIF-8 by the stirring method and introduced g-C3N4 on this basis, modifying ZIF-8 onto the surface of g-C3N4 and investigating its enhanced effect on CO2 reduction performance. The in situ prepared ZIF-8/g-C3N4 composite material has stronger light capture ability, higher charge separation efficiency, and greater CO2 absorption capacity. As a result, compared to traditional urea-thermal polymerized g-C3N4, the yields of CO and CH4 are significantly improved, with the yield of CH4 increasing more than sevenfold.
2 Experimental
2.1 Chemical
All reagents with analytical reagent grade were used as received without further purification. Urea, zinc nitrate hexahydrate, 2-methylimidazole, methanol were purchased from Sinopharm Chemical Reagent Co., Ltd. Deionized water was used throughout the experiment.
2.1.1. The synthesis of g-C3N4. g-C3N4 was prepared using a high-temperature condensation method, with urea as the precursor. The process involved placing urea in a covered Al2O3 crucible, heating from room temperature to 550 °C at a rate of 8 °C min−1, maintaining this temperature for 3 hours, and allowing the material to naturally cool, resulting in the formation of g-C3N4.11
2.1.2. The synthesis of ZIF-8/g-C3N4. Employing an in situ method to fabricate ZIF-8/g-C3N4 composites. To enhance the integration of ZIF-8 with g-C3N4, the experiment first involves the combination of Zn(NO3)2·6H2O with g-C3N4. The specific steps are as follows: 0.63 g of zinc nitrate and 1.26 g of g-C3N4 are weighed and added to 60 mL of methanol, followed by ultrasonication for 1 hour, during which the zinc nitrate dissolves. Then, 60 mL of a methanol solution containing 1.6 g of 2-methylimidazole is quickly poured into the above solution, maintained under ultrasonication for 30 minutes, and then mechanically stirred for 8 hours. The mixed solution is centrifuged, and the resultant mixture is dried at 60 °C for 6 hours to obtain the ZIF-8/g-C3N4 composite photocatalytic material. To investigate the effects of different ZIF-8 to g-C3N4 ratios on the photocatalytic CO2 reduction performance, experiments were designed with composite photocatalytic materials at different molar percentages of 20%, 40%, 60%, and 80%, denoted as Z20CN, Z40CN, Z60CN, and Z80CN, respectively Pure ZIF-8 was also prepared utilizing the procedure described previously, with the exclusion of g-C3N4 addition.
2.2 Characterization of photocatalyst
The phase structure of the catalysts obtained from the experiment was qualitatively analyzed using X-ray diffraction (XRD), with the measurements carried out using a D8-ADVANCE X-ray diffractometer from Bruker, Germany. The micro-morphology of the catalysts was observed using a dual-beam field emission scanning electron microscope (SEM), specifically a GAIA 3 XMN model from TESCAN, Czech Republic, equipped with an energy dispersive X-ray (EDX) scanner for quantitative and qualitative analysis of the elemental distribution within the catalysts. Prior to testing, gold sputtering was performed using a JFC-1600 ion sputter from JEOL Ltd, Japan. High-performance imaging X-ray photoelectron spectroscopy (XPS) was used for qualitative analysis of the composition and chemical states of the catalysts, with measurements taken using an ESCALAB 250Xi from Thermo Fisher Scientific, USA. Binding energies of all samples were calibrated to the C 1s peak at 284.8 eV. FT-IR was employed to analyze the structure and functional groups of the catalysts, using an FT-IR 1200 spectrometer from ST Japan, with pure KBr as the background and a scanning range of 4000 to 500 cm−1. Ultraviolet-visible diffuse reflectance spectroscopy (UV-vis) was used to analyze the light absorption properties of semiconductor materials, their electronic structures, and to calculate the band gap width of semiconductor materials. The measurements were carried out using a U-3900 spectrophotometer from Hitachi, Japan, with a test wavelength range of 200–800 nm. Photoluminescence spectroscopy (PL) was utilized to analyze the migration, transfer, and separation efficiency of photo-generated electron–hole pairs in semiconductors, with the characterization performed using an F-4600 high-performance imaging X-ray photoelectron spectrometer from Hitachi, Japan, obtaining photoluminescence spectra of the samples under an excitation wavelength of 370 nm. Thermogravimetric analysis was conducted in an oxidative atmosphere utilizing a DTG-60H analyzer from Shimadzu, Japan. Nitrogen adsorption–desorption isotherms were quantified using an ASAP2460 from Micromeritics, USA. CO2 adsorption measurements were performed at room temperature using Micromeritics ASAP 2460 from Micromeritics, USA. Electrochemical testing of the photoelectrochemical properties was performed using a 1260 A + 1287 A electrochemical workstation from Solartron Analytical, UK, with the excitation light source provided by a PLS-SXE300 xenon lamp from Beijing Perfectlight Technology Co., Ltd, and transient photocurrent tests were conducted at 0.1 V for a duration of 540 s.
2.3 Examination of photocatalytic CO2 reduction performance
In this experiment, a typical gas–solid CO2 reduction reaction was selected.12 It is to prevent the impact of water photolysis on the experiment. If a hydrogen source is introduced, the photogenerated charges and energy produced by the catalyst would suffice to meet the demands for both photocatalytic water splitting and CO2 reduction.13 The experimental procedure is as follows: the photocatalytic CO2 reduction performance was evaluated in a 250 mL photoreactor equipped with an optical quartz window. The opening of the reactor was sealed with a silicone stopper, and a 300 W xenon lamp simulating sunlight served as the light source, positioned approximately 15 cm above the reactor. 0.5 mL of H2O and 50 mg of catalyst powder were added to the sealed glass reactor, uniformly dispersed on a glass plate. Before illumination, the reactor was evacuated and then filled with 20 mL of high-purity CO2 (99.999%). After 3 hours of light irradiation, the products were analyzed using a gas chromatograph (GC-2014C, Shimadzu, Japan) equipped with a flame ionization detector (FID) and an automatic sampler. The reported gas volumes represent the average of three experimental trials.
3 Results and discussion
3.1 Characterization of the photocatalysts
X-ray diffraction analysis was conducted to study the crystal structure of each sample, with the results shown in (Fig. 1). g-C3N4, Z20CN, and Z40CN all exhibited characteristic diffraction peaks of g-C3N4 at 12.8° and 27.6° (JCPDS Card No. 87-1526),14 corresponding to the (100) and (002) planes of g-C3N4, respectively.40 The (100) plane reflects the in-plane periodic arrangement of the tri-s-triazine ring structure of the g-C3N4 molecular skeleton, while the (002) plane indicates the interlayer stacking of conjugated aromatic ring structures.15 The strong and pure diffraction peaks of ZIF-8 indicate its high crystallinity. Multiple characteristic crystal planes of ZIF-8, including the (010), (002), (112), and (022) planes, can be observed in the XRD pattern, consistent with previous reports.16 The standard peaks of the synthesized ZIF-8 were also compared with the simulated ZIF-8 (JCPDS 00-062-1030) and are depicted in Fig. S1.† The diffraction peaks of composites doped with different ratios of ZIF-8 show slight variations. In Z20CN and Z40CN, the (002) plane of g-C3N4 is clearly visible, due to the lower doping amount of ZIF-8; however, in Z60CN and Z80CN, the intensity of the (002) peak gradually decreases. Notably, the position of the (002) peak shifts towards a lower angle, indicating an increase in the lattice spacing due to the addition of ZIF-8.17 As the amount of ZIF-8 increases, the diffraction peaks of ZIF-8 become more prominent while the characteristic peaks of g-C3N4 gradually weaken, suggesting an effective composite formation between ZIF-8 and g-C3N4.
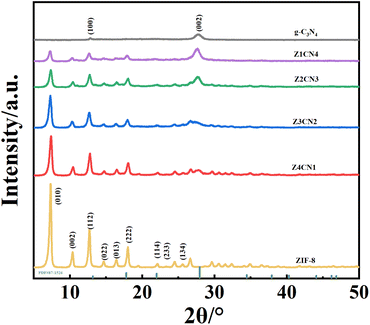 |
| Fig. 1 XRD patterns of diverse photocatalysts. | |
Field emission scanning electron microscopy (FESEM) and transmission electron microscopy (TEM) were employed to investigate the microstructure of g-C3N4, ZIF-8, and Z60CN. As depicted in (Fig. 2a), g-C3N4 exhibits a layered stacking of agglomerated clusters,18 characterized by a relatively loose structure with irregular morphology and size. (Fig. 2b) shows the rhombic dodecahedral morphology of ZIF-8, with microcrystals measuring 80–100 nm in diameter, demonstrating well-crystallized particles, regular shapes, and uniform dispersion.19 (Fig. 2c) reveals the uniform distribution of ZIF-8 octahedral cubic particles on the surface of g-C3N4, with the agglomerated clusters of g-C3N4 in the composite product undergoing fragmentation, thereby enhancing the overall particle dispersion and uniformity. The TEM image of Z60CN (Fig. 2d) further confirms the uniform deposition of ZIF-8 on g-C3N4 sheets, indicating a tight integration between the two. The energy-dispersive X-ray spectroscopy (EDS) elemental maps of Z60CN (Fig. 2e–h) clearly demonstrate that, beyond the presence of carbon (C) and nitrogen (N), zinc (Zn) is also uniformly distributed within the composite material.20 These outcomes decisively validate the formation of an effective composite between g-C3N4 and ZIF-8, aligning with the X-ray diffraction (XRD) observations detailed earlier.
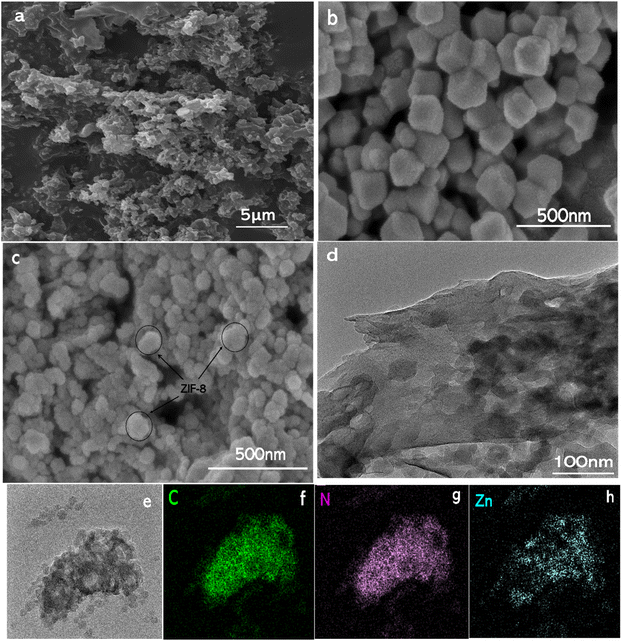 |
| Fig. 2 SEM images of (a) g-C3N4 (b) ZIF-8 (c) Z60CN; (d) TEM images of Z60CN; (e–h) the EDX elemental maps of Z60CN. | |
Thermogravimetric analysis (TGA) under air was utilized to ascertain the actual ZIF-8 loading in the samples. As Fig. 3a illustrates, the three samples demonstrated excellent thermal stability with less than 10% mass loss between 0–350 °C, likely due to the loss of impurities adhered to the surface or within pores, and water adsorbed on the surface. Decomposition of g-C3N4 commenced at approximately 550 °C, culminating in complete sublimation and decomposition. ZIF-8 exhibited a weight loss of around 70% from 350 °C to 600 °C, indicative of the metal–organic framework's collapse, leaving ZnO as the residue. Within the same temperature range, Z60CN showed an 85% weight loss, with ZnO being the remaining product, which correlates with ZIF-8 deposited on the Z60CN surface. With ZIF-8 molecular weight at 227.6 g mol−1, including one Zn atom (65.4 g mol−1), the additional ∼8% mass loss of Z60CN compared to g-C3N4 allowed for the calculation of the actual ZIF-8 loading on the composite Z60CN to be 63.3%. This confirms the actual presence of ZIF-8 in the prepared samples aligns with the designed values.
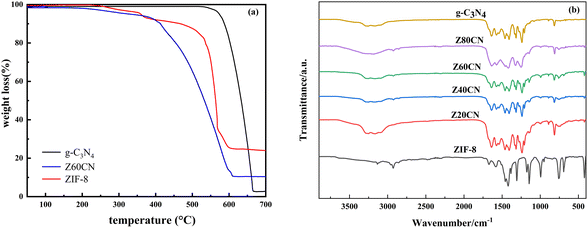 |
| Fig. 3 (a) TG analyses of sample ZIF-8, g-C3N4 and Z60CN; (b) the FT-IR spectra of different photocatalysts. | |
Fig. 3b delineates the Fourier-transform infrared (FT-IR) spectral profiles for a suite of photocatalysts. The spectral signatures of g-C3N4 and ZIF-8/g-C3N4 composites exhibit congruent stretching vibrational modes, evidencing that the quintessential lattice structure of g-C3N4 is retained after surface modification. The absorption peaks observed in the range of 3000–3500 cm−1 for g-C3N4 and the ZIF-8/g-C3N4 composite samples are attributed to the stretching vibrations of the NH and NH2 functional groups,3,21 while the peaks from 1200 to 1630 cm−1 are due to the typical stretching vibrations of the g-C3N4 heterocycles. The absorption peak at 815 cm−1 for both g-C3N4 and the composite samples corresponds to the bending vibrations of the triazine structure in g-C3N4.42 The absorption peaks in the range of 1350–900 cm−1 and at 800 cm−1 are assigned to the in-plane and out-of-plane bending vibrations of the imidazole rings.22 Notably, the absorption peak at 815 cm−1 for the ZIF-8/g-C3N4 composite samples shifts to a higher wavenumber, which may originate from structural distortions of the triazine ring units in ZIF-8/g-C3N4. Additionally, a new characteristic peak at 422 cm−1 appears for ZIF-8 and its composites, corresponding to the stretching vibration of the Zn–N bond,23 further corroborating the effective composite formation between the two materials by the in situ method.
Fig. 4a–d display the XPS spectra of g-C3N4 and Z60CN. As shown in the full scan spectrum in Fig. 4a. C, N, and O elements were detected in both g-C3N4 and Z60CN, with the O 1s peak plausibly attributable to the adsorption of CO2 and H2O molecules on the sample's surface. The characteristic peak of C 1s at 284.8 eV serves as a charge correction reference for the analysis of the elements. Fig. 4b shows the high-resolution C 1s spectrum, where the characteristic peak in g-C3N4 at a binding energy of 287.80 eV corresponds to the sp2 hybridized orbitals of aromatic N
C–N.24 In Z60CN, this peak shifts by 0.05 eV to a lower binding energy at 287.75 eV, suggesting effective composite formation between ZIF-8 and g-C3N4 and indicating charge transfer or changes in the chemical environment on the surface of the triazine rings. Fig. 4c presents the high-resolution N 1s spectrum, with g-C3N4 fitted with two characteristic peaks at 398.45 eV and 400.00 eV, corresponding to the C
N–C within the triazine rings and the bridging nitrogen atoms N–(C)3 hybridized orbitals,25,26 respectively. The peaks in Z60CN shift to 398.6 eV and 400.2 eV, indicating that the chemical state of N is influenced by the composite of g-C3N4 and ZIF-8. Fig. 4d reveals two distinct peaks at 1022.1 eV and 1045.2 eV, corresponding to Zn 2p3/2 and Zn 2p1/2, respectively. The observed chemical shifts in the carbon and nitrogen elements in Z60CN relative to g-C3N4 further indicate the effective integration of ZIF-8 into the g-C3N4 lattice, in alignment with the findings from X-ray diffraction analysis.
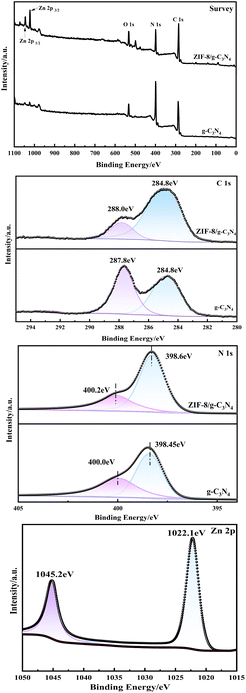 |
| Fig. 4 (a) XPS full scan spectrum of g-C3N4 and ZIF-8/g-C3N4, (b) C ls, (c) N ls, (d) Zn 2p. | |
The UV-vis absorption spectra of the samples (Fig. 5a) reveal that the absorption capacity of ZIF-8/g-C3N4 in the ultraviolet and visible light regions is enhanced compared to g-C3N4, with the absorption edge of each experiencing a red shift. Notably, the absorption edge of Z60CN shifts to 456 nm, indicating a significant improvement in visible light absorption. The bandgap energy (Eg) can be calculated using:
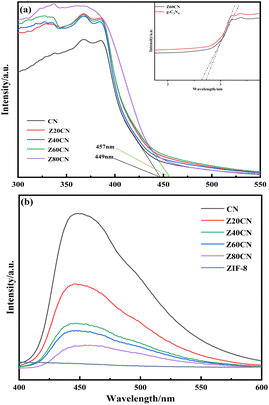 |
| Fig. 5 (a) UV-vis and corresponding relationship between optical energy changes of different samples, (b) PL. | |
The calculated bandgap energies for g-C3N4 and Z60CN are 2.76 eV and 2.71 eV, respectively, indicating that the integration of ZIF-8 reduces the bandgap of g-C3N4 by 0.05 eV. Thus, the red shift and the narrowing of the bandgap in Z60CN suggest that it can excite electrons from the valence band to the conduction band at lower energies than g-C3N4, potentially enhancing the efficiency of CO2 reduction during photocatalytic processes.
Fig. 5b presents the photoluminescence (PL) spectra of different photocatalysts. It is observed that the fluorescence intensity of ZIF-8/g-C3N4 composites is significantly lower than that of g-C3N4, indicating a much slower recombination rate of electron–hole pairs in the composites. This slow recombination facilitates more effective separation and migration of electron–hole pairs.27 Therefore, the introduction of ZIF-8 markedly suppresses the recombination of charge carriers within g-C3N4. This suppression contributes to a higher utilization rate of electron–hole pairs,41 thereby enhancing the activity of the photocatalyst and leading to a more efficient photocatalytic reaction.28 ZIF-8 not only improves the light absorption properties of g-C3N4 but also boosts the photocatalytic efficiency through the diminution of charge carrier recombination rates.
Fig. 6 displays the transient photocurrent response of g-C3N4 and Z60CN. The transient photocurrent intensity of Z60CN is notably higher than that of g-C3N4, indicating that a greater number of electrons per unit time pass through the Z60CN electrode, which suggests a higher efficiency of photogenerated carrier separation. This is in agreement with the fluorescence test results discussed earlier.
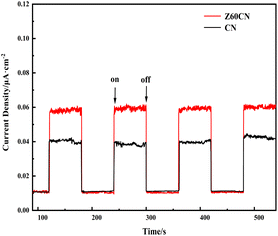 |
| Fig. 6 Transient photocurrent response graphs of g-C3N4 and Z60CN. | |
3.2 Adsorption performance
Nitrogen adsorption measurements were employed to characterize the pore structure and specific surface area of the synthesized samples. A larger specific surface area in catalysts typically indicates more adsorption and active sites, which may translate to higher catalytic performance. Fig. 7a presents the adsorption–desorption isotherms of g-C3N4 and Z60CN, both of which exhibit type IV classification.29,30 The presence of H3 hysteresis loop in the relative pressure (P/P0) range of 0.60–0.95 suggests the presence of mesopores.31 The hysteresis loop observed in the isotherms extends across a substantial range of relative pressures, signifying a heterogeneous mesopore size distribution. This is substantiated by the commensurately broad pore-size distributions delineated by the Barrett–Joyner–Halenda (BJH) analysis. Table 1 enumerates the specific surface areas, pore volumes, and average pore diameters for each of the samples. Overall, in this study, the construction of a composite photocatalyst was demonstrated to increase the specific surface area of g-C3N4, resulting in more small-sized mesopores.32
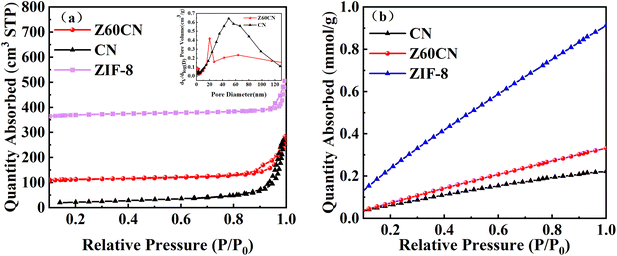 |
| Fig. 7 (a) N2 adsorption–desorption isotherms and pore size distribution curves of sample CN and Z60CN (b) CO2 adsorption curves of the sample CN, ZIF-8 and Z60CN. | |
Table 1 Comparison of the SBET, pore volume, average pore size, CO2 adsorption
Sample |
SBET (m2 g−1) |
Pore volume (cm3 g−1) |
Average pore size (nm) |
CO2 adsorption (mmol g−1) |
g-C3N4 |
73.87 |
0.41 |
22.40 |
0.21 |
Z60CN |
363.41 |
0.43 |
4.80 |
0.32 |
ZIF-8 |
1182.69 |
0.78 |
2.64 |
0.91 |
There is ample literature documenting that the adsorption of CO2 on the surface of photocatalysts is a primary key step affecting the pathways and kinetics of photocatalytic CO2 reduction reactions.33 An in-depth study of the CO2 adsorption performance of g-C3N4, Z60CN, and ZIF-8 samples was conducted via CO2 adsorption isotherms shown in Fig. 7b. Notably, all samples exhibit a linear relationship between CO2 adsorption capacity and relative pressure (P/P0) in the range of 0.1 to 1.0.34 This relationship suggests that the interactions between CO2 and the samples are predominantly physical.35 In the relative pressure (P/P0) range of 0.2–1.0, the CO2 adsorption capacity of Z60CN is significantly higher than that of pure g-C3N4, which is attributable to Z60CN higher specific surface area, thereby exhibiting more pronounced CO2 adsorption. In summary, the introduction of ZIF-8 to modify the g-C3N4 photocatalyst effectively tunes its adsorption properties.36
3.3 Photocatalytic CO2 reduction performance
In a typical gas–solid system, the photocatalytic CO2 reduction capabilities of all fabricated samples were assessed under irradiation from a 300 watt xenon lamp without an ultraviolet filter. Control experiments confirmed that in the absence of both photocatalyst and light exposure, byproducts such as CO and CH4 remained undetected. Although it has been demonstrated in recent years that some MOFs exhibit photocatalytic activity for CO2 reduction under light irradiation,37 no organic products were detected during prolonged CO2 photoreduction using pure ZIF-8 as the photocatalyst. This observation that, within the context of this investigation, the presence of both light irradiation and the photocatalyst are essential prerequisites for the effective of CO2 reduction.
As shown in Fig. 8(a) and (b), as marked increase in the photocatalytic activity for generating CO and CH4 was achieved with g-C3N4 photocatalysts composited with ZIF-8. The total yield of CO and CH4 increased with the extension of light irradiation time. For Z20CN composited with ZIF-8, the yield of CO production increased by about 70% after 3 hours (Fig. 8a). Meanwhile, for Z60CN composited with ZIF-8, the yield of CH4 production could be increased by more than 7 times after 3 hours (Fig. 8b). It is important to note that the amount of ZIF-8 composite is crucial for photocatalytic CO2 reduction. As delineated in earlier discussions, the addition of ZIF-8 substantially amplifies CO2 capture, serving as an essential preliminary phase to the ensuing photocatalytic conversion of CO2 into CO and CH4. It is generally observed that an increase in the CO2 adsorption capacity of a semiconductor photocatalyst is directly correlated with an improvement in its photocatalytic CO2 reduction efficacy. Therefore, initially, as the amount of ZIF-8 composite increases, the yield of photocatalytic CH4 continuously increases. However, the amount of ZIF-8 composite seems to have a dual effect on the performance of photocatalytic CH4 production. When the molar ratio of ZIF-8 to g-C3N4 is 6
:
4 (sample Z60CN), the highest yield of photocatalytic CH4 production after 3 hours is achieved, reaching 2.56 μmol g−1. Although the introduction of ZIF-8 can enhance CO2 adsorption, the poor conductivity of ZIF-8 may inhibit surface charge transfer, as also confirmed by the analysis of fluorescence spectra and transient photocurrent responses.43 To assess the durability of the ZIF-8/g-C3N4 composite photocatalyst, we recovered the catalyst after the reactions and conducted XRD comparisons, as shown in Fig. 8(c). The results indicate that the ZIF-8/g-C3N4 composite maintains excellent stability for the photocatalytic reduction of CO2, showing no significant changes in the crystal structure, which suggests its high durability in photocatalytic applications.
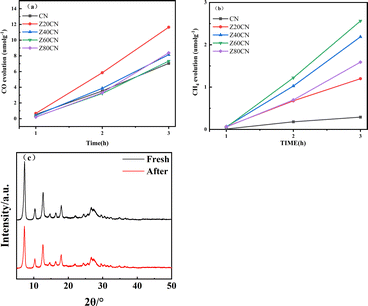 |
| Fig. 8 (a) Comparison of CO production rates for the prepared samples. (b) Comparison of CH4 production rates for the prepared samples. (c) XRD patterns of Z60CN before and after the catalytic reactions. | |
In this investigation, across the spectrum of synthesized photocatalysts, CO2 is predominantly converted into CO and CH4 as the primary outputs in the photocatalytic reduction process. The possible mechanism for the formation of CH4 can be described by the following reaction formula,38 based on the presence of different intermediate products:
2CO2 + 4e− + 4h+ → 2CO2 + O2 |
CO +6e− + 6H+ → CH4 + H2O |
Based on the results above, a possible degradation mechanism was proposed in Fig. 9. Although the ZIF-8 in the composite cannot be excited by the visible light, the g-C3N4 component can be excited. Under illumination, g-C3N4 can be excited to generate electron–hole pairs, with the excited electrons transitioning from the valence band (VB) to the conduction band (CB). The conduction band of ZIF-8 is more negative compared to that of g-C3N4, prompting the electrons to migrate towards the energetically favorable conduction band of ZIF-8. Given ZIF-8's large surface area and high porosity, it exhibits strong CO2 adsorption properties. Thus, the electrons flowing to ZIF-8 interact with the adsorbed CO2. Since the CB potential of ZIF-8 (−2.22 eV) is more negative than the potential of the CO2/CH4 redox couple,39 the accumulated electrons in the CB of ZIF-8 can effectively reduce CO2 to produce CH4 and CO.
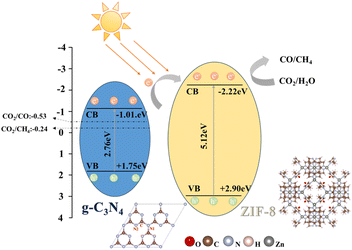 |
| Fig. 9 Mechanism diagram of ZIF-8/g-C3N4 photocatalytic CO2 reduction. | |
4 Conclusions
The ZIF-8/g-C3N4 series of composite photocatalytic materials was synthesized using an in situ deposition method. The designed composite photocatalytic materials largely inherit the optical absorption characteristics of g-C3N4, enhance photocatalytic redox capability, improve efficiency of charge separation, and possess excellent CO2 adsorption capacity. The findings demonstrate that the Z60CN composite photocatalyst displays enhanced photocatalytic performance, reaching a methane production of 2.56 μmol g−1 in 3 hours, which is 7 times that of g-C3N4, without the addition of a sacrificial agent. Z60CN shows lower photoluminescence (PL) intensity and higher photocurrent density than g-C3N4, suggesting that this structure is conducive to interfacial charge transfer. Moreover, ZIF-8's limited inherent conductivity impedes charge transfer within the system. Notably, as the proportion of ZIF-8 in the composite increases, the photocatalytic efficiency of CO2 reduction for Z80CN declines compared to Z60CN. This illustrates a trade-off between charge transfer efficiency and CO2 capture capabilities in the composites. Therefore, the precise quantification of ZIF-8 is pivotal in enhancing photocatalytic efficacy. This research offers fresh perspectives on the development of effective CO2 photocatalysts, leveraging the synergistic interactions between semiconductor nanostructures and metal–organic frameworks.
Author contributions
Y. H. S. and L. Y. synthesized the samples and performed the performance tests. R. F. W and S. L. A. conceived the idea, coordinated all stages of this research, and supervised the work. Y. H. S. and Z. H. wrote the manuscript. All authors contributed to the manuscript.
Conflicts of interest
The authors declare no competing interests.
Acknowledgements
This work was supported by the Inner Mongolia Autonomous Region Science and Technology Program (2021GG0042); Inner Mongolia Autonomous Region Youth Science and Technology Excellence in Higher Education (NJYT22064); Inner Mongolia Autonomous Region Natural Science Foundation Program (2022MS05018) Basic research business cost project in universities (0406082217).
References
- Y. Zhang, J. Zhou and Q. Feng, et al., Visible light photocatalytic degradation of MB using UiO-66/g-C3N4 heterojunction nanocatalyst, Chemosphere, 2018, 212, 523–532 CrossRef CAS PubMed.
- S. Liu, F. Chen and S. Li, et al., Enhanced photocatalytic conversion of greenhouse gas CO2 into solar fuels over g-C3N4 nanotubes with decorated transparent ZIF-8 nanoclusters, Appl. Catal., B, 2017, 211, 1–10 CrossRef CAS.
- J. Wen, J. Xie and X. Chen, et al., A review on g-C3N4-based photocatalysts, Appl. Surf. Sci., 2017, 391, 72–123 CrossRef CAS.
- A. Dhakshinamoorthy, A. M. Asiri and H. Garcia, Metal–organic framework (MOF) compounds: photocatalysts for redox reactions and solar fuel production, Angew. Chem., Int. Ed., 2016, 55, 5414–5445 CrossRef CAS PubMed.
- S. Liu, J. Wang and J. Yu, ZIF-8 derived bimodal carbon modified ZnO photocatalysts with enhanced photocatalytic CO2 reduction performance, RSC Adv., 2016, 6, 59998–60006 RSC.
- Z. Y. Yeo, P. Y. Tan and S. Chai, et al., Continuous polycrystalline ZIF-8 membrane supported on CO2-selective mixed matrix supports for CO2/CH4 separation, RSC Adv., 2014, 4, 52461–52466 RSC.
- S. R. Venna, J. B. Jasinski and M. A. Carreon, Structural evolution of zeolitic imidazolate framework-8, J. Am. Chem. Soc., 2010, 132, 18030–18033 CrossRef CAS PubMed.
- C. Chizallet, S. Lazare and D. Bazer-Bachi, et al., Catalysis of transesterification by a nonfunctionalized metal−organic framework: acido–basicity at the external surface of ZIF-8 probed by FTIR and ab initio calculations, J. Am. Chem. Soc., 2010, 132, 12365–12377 CrossRef CAS PubMed.
- U. P. Tran, K. K. Le and N. T. Phan, Expanding applications of metal−organic frameworks: zeolite imidazolate framework ZIF-8 as an efficient heterogeneous catalyst for the knoevenagel reaction, ACS Catal., 2011, 1, 120–127 CrossRef CAS.
- Y. Lee, M. Jang and H. Cho, et al., ZIF-8: A comparison of synthesis methods, Chem. Eng. J., 2015, 271, 276–280 CrossRef CAS.
- F. Saman, H. Bahruji and A. H. Mahadi, et al., Pd/g-C3N4 photocatalyst for hydrogen production: Role of experimental condition for Schottky barrier, Fuel, 2023, 349, 128725 CrossRef CAS.
- K. M. Kamal, R. Narayan and N. Chandran, et al., Synergistic enhancement of photocatalytic CO2 reduction by plasmonic Au nanoparticles on TiO2 decorated N-graphene heterostructure catalyst for high selectivity methane production, Appl. Catal., B, 2022, 307, 121181 CrossRef CAS.
- W. Dong, J. Jia and Y. Wang, et al., Visible-light-driven solvent-free photocatalytic CO2 reduction to CO by Co-MOF/Cu2O heterojunction with superior selectivity, Chem. Eng. J., 2022, 438, 135622 CrossRef CAS.
- J. Wang, J. Huang and H. Xie, et al., Synthesis of g-C3N4/TiO2 with enhanced photocatalytic activity for H2 evolution by a simple method, Int. J. Hydrogen Energy, 2014, 39, 6354–6363 CrossRef CAS.
- Y. Li, S. Wu and L. Huang, et al., Synthesis of carbon-doped g-C3N4 composites with enhanced visible-light photocatalytic activity, Mater. Lett., 2014, 137, 281–284 CrossRef CAS.
- N. Hassan, A. Shahat and A. El-Didamony, et al., Equilibrium, Kinetic and Thermodynamic studies of adsorption of cationic dyes from aqueous solution using ZIF-8, Moroccan J. Chem., 2020, 8, 3–8 Search PubMed.
- X. Guo, S. He and Z. Meng, et al., Ag@ZIF-8/g-C3N4 Z-scheme photocatalyst for the enhanced removal of multiple classes of antibiotics by integrated adsorption and photocatalytic degradation under visible light irradiation, RSC Adv., 2022, 12, 17919–17931 RSC.
- X. Li, B. Wang and W. Yin, et al., Cu2+ modified g-C3N4 photocatalysts for visible light photocatalytic properties, Acta Phys. Chim. Sin., 2020, 36, 1902001 Search PubMed.
- B. Liu, C. Bie and Y. Zhang, et al., Hierarchically porous ZnO/g-C3N4 S-scheme heterojunction photocatalyst for efficient H2O2 production, Langmuir, 2021, 37, 14114–14124 CrossRef CAS PubMed.
- Y. Zhao, Y. Li and L. Chang, et al., Bimetal doped Cu–Fe-ZIF-8/g-C3N4 nanocomposites for the adsorption of tetracycline hydrochloride from water, RSC Adv., 2024, 14, 4861–4870 RSC.
- M. Tahir, C. Cao and F. K. Butt, et al., Large scale production of novel g-C3N4 micro strings with high surface area and versatile photodegradation ability, CrystEngComm, 2014, 16, 1825–1830 RSC.
- Y. Hu, H. Kazemian and S. Rohani, et al., In situ high pressure study of ZIF-8 by FTIR spectroscopy, Chem. Commun., 2011, 47, 12694–12696 RSC.
- L. Lin, T. Zhang and H. Liu, et al., In situ fabrication of a perfect Pd/ZnO@ZIF-8 core–shell microsphere as an efficient catalyst by a ZnO support-induced ZIF-8 growth strategy, Nanoscale, 2015, 7, 7615–7623 RSC.
- C. Chen, Y. He and G. Xiao, et al., Graphic C3N4-assisted dispersion of graphene to improve the corrosion resistance of waterborne epoxy coating, Prog. Org. Coat., 2020, 139, 105448 CrossRef CAS.
- H. Cai, D. Han and X. Wang, et al., High specific surface area defective g-C3N4 nanosheets with enhanced photocatalytic activity prepared by using glyoxylic acid mediated melamine, Mater. Chem. Phys., 2020, 256, 123755 CrossRef CAS.
- C. Saka, Surface modification with oxygen doping of g-C3N4 nanoparticles by carbon vacancy for efficient dehydrogenation of sodium borohydride in methanol, Fuel, 2022, 310, 122444 CrossRef CAS.
- L. Jiang, X. Yuan and Y. Pan, et al., Doping of graphitic carbon nitride for photocatalysis: a review, Appl. Catal., B, 2017, 217, 388–406 CrossRef CAS.
- X. Liu, J. Zhang and Y. Dong, et al., A facile approach for the synthesis of Z-scheme photocatalyst ZIF-8/g-C3N4 with highly enhanced photocatalytic activity under simulated sunlight, New J. Chem., 2018, 42, 12180–12187 RSC.
- K. S. Sing, Reporting physisorption data for gas/solid systems with special reference to the determination of surface area and porosity (Recommendations 1984), Pure Appl. Chem., 1985, 57, 603–619 CrossRef CAS.
- L. Wang, P. Jin and S. Duan, et al., In-situ incorporation of Copper (II) porphyrin functionalized zirconium MOF and TiO2 for efficient photocatalytic CO2 reduction, Sci. Bull., 2019, 64, 926–933 CrossRef CAS PubMed.
- G. Tzvetkov, M. Tsvetkov and T. Spassov, Ammonia-evaporation-induced construction of three-dimensional NiO/g-C3N4 composite with enhanced adsorption and visible light-driven photocatalytic performance, Superlattices Microstruct., 2018, 119, 122–133 CrossRef CAS.
- Y. Zhou, L. Zhang and W. Huang, et al., N-doped graphitic carbon-incorporated g-C3N4 for remarkably enhanced photocatalytic H2 evolution under visible light, Carbon, 2016, 99, 111–117 CrossRef CAS.
- Q. Huang, J. Yu and S. Cao, et al., Efficient photocatalytic reduction of CO2 by amine-functionalized g-C3N4, Appl. Surf. Sci., 2015, 358, 350–355 CrossRef CAS.
- J. Yu, S. Wang and B. Cheng, et al., Noble metal-free Ni (OH)2-g-C3N4 composite photocatalyst with enhanced visible-light photocatalytic H2-production activity, Catal. Sci. Technol., 2013, 3, 1782–1789 RSC.
- Z. Sun, Z. Yang and H. Liu, et al., Visible-light CO2 photocatalytic reduction performance of ball-flower-like Bi2WO6 synthesized without organic precursor: effect of post-calcination and water vapor, Appl. Surf. Sci., 2014, 315, 360–367 CrossRef CAS.
- K. K. Han, C. C. Wang and Y. Y. Li, et al., Facile template-free synthesis of porous g-C3N4 with high photocatalytic performance under visible light, RSC Adv., 2013, 3, 9465–9469 RSC.
- D. Li, M. Kassymova and X. Cai, et al., Photocatalytic CO2 reduction over metal-organic framework-based materials, Coord. Chem. Rev., 2020, 412, 213262 CrossRef CAS.
- O. K. Varghese, M. Paulose and T. J. LaTempa, et al., High-rate solar photocatalytic conversion of CO2 and water vapor to hydrocarbon fuels, Nano Lett., 2009, 9, 731–737 CrossRef CAS PubMed.
- C. Chen, J. Jin and S. Chen, et al., In situ growth of ultrafine ZnO on g-C3N4 layer for highly active and selective CO2 photoreduction to CH4 under visible light, Mater. Res. Bull., 2021, 137, 111177 CrossRef CAS.
- H. He, Z. Luo and C. Yu, Diatomite-anchored g-C3N4 nanosheets for selective removal of organic dyes, J. Alloys Compd., 2020, 816, 152652 CrossRef CAS.
- Y. Yang, J. Wu and B. Cheng, et al., Enhanced photocatalytic H2-production activity of CdS nanoflower using single atom Pt and graphene quantum dot as dual cocatalysts, Chin. J. Struct. Chem., 2022, 41, 2206006–2206014 CAS.
- C. Yu, H. He and W. Zhou, et al., Novel rugby-ball-like Zn3 (PO4)2@C3N4 photocatalyst with highly enhanced visible-light photocatalytic performance, Sep. Purif. Technol., 2019, 217, 137–146 CrossRef CAS.
- F. Hong-Ru, Y. Jiang and L. Jia-Hua, et al., A robust heterometallic Cd(II)/Ba(II)-organic framework with exposed amino group and active sites exhibiting excellent CO2/CH4 and C2H2/CH4 separation, Chin. J. Struct. Chem., 2022, 41, 2203287–2203292 Search PubMed.
|
This journal is © The Royal Society of Chemistry 2024 |
Click here to see how this site uses Cookies. View our privacy policy here.