DOI:
10.1039/D4RA03156C
(Paper)
RSC Adv., 2024,
14, 16678-16684
Revisiting the 1,3-azadiene-succinic anhydride annulation reaction for the stereocontrolled synthesis of allylic 2-oxopyrrolidines bearing up to four contiguous stereocenters†
Received
28th April 2024
, Accepted 15th May 2024
First published on 22nd May 2024
Abstract
Polysubstituted 2-oxopyrrolidines bearing at least two contiguous stereocenters constitute the core of several pharmaceuticals, including clausenamide (antidementia). Here, we describe a flexible annulation strategy, which unites succinic anhydride and 1,3-azadienes to produce allylic 2-oxopyrrolidines bearing contiguous stereocenters. The approach is chemoselective, efficient, modular, scalable, and diastereoselective. The scalable nature of the reactions offers the opportunity for post-diversification, leading to incorporation of motifs with either known pharmaceutical value or that permit subsequent conversion to medicinally relevant entities.
Introduction
There are high incentives for the construction of sp3-rich azaheterocyclic scaffolds.1 It is well established that two compounds with the same molecular formula and connectivity, but with different orientations in space (i.e., stereoisomers) can produce vastly different biological responses.2 Specifically, functionalized 2-oxopyrrolidines (i.e., γ-lactams) and pyrrolidines bearing contiguous stereocenters constitute the core of several alkaloid natural products and pharmaceuticals (Fig. 1). The γ-lactam topology also presents an ideal platform for systematic scaffolding owing to its latent reactivity and the diverse number of transformations it can undergo.3 Notable strategies for the construction of functionalized 2-oxopyrrolidines include ring-opening of aziridines,4 the aza-Heck reaction,5 and cascade/multicomponent reactions.6 These methodological advances notwithstanding, approaches for the stereocontrolled synthesis of polysubstituted γ-lactams bearing contiguous stereocenters are still underdeveloped.7 One strategy to vicinally functionalized and alkenylated γ-lactams that caught our attention, given its reliance on 1,3-azadienes of type A, is the strategy developed and popularized by Rovis and co-workers (Fig. 2A).8
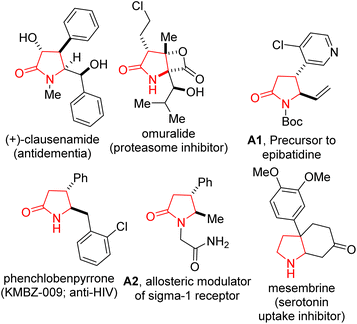 |
| Fig. 1 Examples of bioactive 2-oxopyrrolidines and pyrrolidines bearing vicinal stereocenters. | |
 |
| Fig. 2 (A) Prior synthesis of allylic 2-pyrrolidinones featuring 1,3-azadienes, (B) proposed plan for the synthesis of allylic 2-pyrrolidinones and potential synthetic applications, (C) possible chemoselective challenges. | |
Cyclic anhydrides are more than just acylating agents given that they also serve as useful dipolar synthons for the synthesis of complex organic molecules through formal cycloaddition reactions. Our efforts in the last decade to popularize the 1,3-azadiene-anhydride annulation reaction and its synthetic versatility led to discoveries that several 6-membered ring anhydrides (e.g., glutaric anhydride, 2,2-dimethylglutaric anhydride, 3-methylglutaric anhydride, diglycolic anhydride, thiodiglycolic anhydride, and MIDA anhydride) react competently with appropriately substituted 1,3-azadienes.9a–g Recently, we revealed that highly reactive phenylsuccinic anhydride is amenable to chemoselective and stereocontrolled pentannulation with 1,3-azadienes at room temperature.9h–j
Considering the pervasiveness of 2-pyrrolidinones bearing contiguous stereocenters, the synthetic versatility of allylic cyclic amines, and our long-standing interest in vicinally functionalized azaheterocycles,10 we sought to evaluate the performance (with respect to reactivity, diastereoselectivity, and chemoselectivity) of succinic anhydride (2) toward 1,3-azadienes of type 1 (Fig. 2B). Intrinsic to our design was the prospect of being able to rapidly construct 3 and elaborate it to other synthetically attractive topologies such as 4–8. We were however not oblivious to the challenges associated with the proposed 1,3-azadiene-succinic anhydride annulation reaction. For instance, from a chemoselectivity standpoint alone, the reaction between 1 and 2 is capable of producing at least four products (Fig. 2C, see 3′, 9, 11, and 12).11 Product 3′ would arise from the desired imine–anhydride reaction. Conversely, Tamura adduct 9 would be formed if the alkene–anhydride reaction out-competes the imine–anhydride reaction. If the alkene–anhydride reaction is accompanied by tautomerization and concomitant acylation of the anhydride by the resulting enamine, unsaturated caprolactam 11 (see blue arrows) or dihydropyridone 12 (see red arrows) would be formed. We have embraced these challenges and herein disclose our findings on the successful construction of lactam-tethered alkenoic acids of type 3′ and subsequent post-diversification. We employ a ring-forming reaction, which is sustainable, diastereoselective, regioselective, functional group-selective (i.e., chemoselective), modular, and scalable.
Results and discussion
We initiated studies toward the construction of 5-alkenylated-2-oxopyrrolidines and subsequent fragment growth by attempting to find suitable conditions for the annulation reaction between 1,3-azadienes9a of type 1 and anhydride 2. Despite its rich history, the anhydride-imine reaction featuring 2 is still mostly limited to aryl aldimines.12 Nevertheless, we were aware of one unsuccessful attempt involving 1,3-azadiene 1a and 2 (see 3a′, Fig. 3).13 Unfortunately, the authors provided little information with respect to the remaining mass balance and possible side products. N-aryl imines of type 1a are reluctant substrates for the anhydride-imine reaction,14 presumably due to the relatively reduced nucleophilicity of the nitrogen atom as well as their hydrolytic instability. In our hands, the reaction of 1a with 2, in toluene, equally afforded 3a′ in unattractive yield (<10%). However, we found that 1a is fragile leading to the formation of amidoacid G, imide H, and enal I.
 |
| Fig. 3 Unsuccessful attempt at annulation of 1,3-azadiene 1a with 2. | |
Knowing that N-alkyl azadienes are more charitable annulation partners than their N-aryl counterparts,9d we decided to perform optimization studies using azadiene 1b (Table 1). Encouragingly, when an equimolar mixture of 1b and 2, in toluene, was heated to 100 °C, a clean chemoselective annulation reaction occurred and product 3b′ was obtained along with unreacted starting materials (Table 1, entry 1). Reaction optimization was carried out and it was established that 2,2,5,5-tetramethyloxolane (TMO) out-performs other reaction media (e.g., toluene, xylene, THF, 2-MeTHF, DMF, and diphenylether). The chemoselective formation of 2-oxopyrrolidine 3′ indicates a scenario whereby the anhydride–imine pathway out-competes the anhydride–alkene pathways (see Fig. 2C). The reaction proceeds with high stereochemical control, leading to the formation of predominantly one stereoisomer (as judged by GC-MS and 1H NMR analyses of the crude mixture). The atom efficiency of this transformation is commendable, given that with the exception of the water formed during the formation of the 1,3-azadiene (i.e., 1b), all atoms originating from the enal, primary amine, and anhydride 2 are incorporated into the product structure. Although the crucial philosophical criterion of green chemistry (i.e., atom economy) is clearly fulfilled in this 1,3-azadiene-anhydride reaction, it is yet to be considered as a tool for sustainable synthetic chemistry.15 This is presumably because the reaction is typically performed in a high-boiling aromatic hydrocarbon solvent such as toluene. Toluene is also suspected of causing damage to fertility, among other health hazards, while also being a hazardous air pollutant and harmful to aquatic life with long-lasting effects.16 The replacement of toluene by greener and safer solvents is all the more important now that it has been added to the REACH restricted substance list.17,18 We anticipate that the successful deployment of TMO (a green ethereal solvent that does not form peroxides) as the reaction medium in these studies, would confer the 1,3-azadiene-anhydride annulation protocol the coveted ‘green chemistry status’.
Table 1 Optimization of the annulation of 1b with 2

|
Entry |
Solvent |
Temp (°C) |
% yield of 3b′ |
After 2 h. After 12 h. After 22 h. |
1 |
Toluene |
100 |
70 |
2 |
O-xylene |
120 |
27 |
3 |
THF |
60 |
54 |
4 |
2-Me THF |
60 |
69 |
5 |
TMO |
90 |
88 |
6 |
DMF |
90 |
0 |
7 |
Ph2O |
90 |
12 |
8 |
TMO |
100 |
71a |
9 |
TMO |
80 |
77b |
10 |
TMO |
70 |
54c |
11 |
None |
110 |
16 |
The scope of the reaction with respect to the alkenyl moiety as well as the N-substituent has been explored. For purposes of easier isolation and purification, the initially formed carboxylic acid (i.e., 3′) has been converted to the corresponding methyl ester in most cases. The 1H NMR spectra of 3 revealed coupling constants (3JA,B) ranging from 1.0 to 6.0 Hz for protons HA and HB (see Table 1 for depiction of the protons). According to the literature data for similar 4,5-disubstituted 2-pyrrolidinones, the coupling constant, 3JA,B is in the range of 3.4–6.9 Hz for trans-compounds and in the range of 8.0–9.0 Hz for cis-pyrrolidin-2-ones.19 The observed values of 3JA,B for the acids/esters depicted in Scheme 1 fall in the range typical for trans-4,5-disubstituted 2-pyrrolidinones.
 |
| Scheme 1 Annulation of 1,3-azadienes with succinic anhydride (2). | |
Understandably, ortho-substituted styrenes (see 3c vs. 3e) and electron-deficient styrenes (see 3e vs. 3f) react with a slight decrease in efficiency. N-alkyl-, allyl-, benzyl-, and phenethyl-azadienes are well tolerated (see 3b–3z3). As previously stated, N-aryl imines are typically reluctant substrates for the anhydride–imine reaction.14 Nevertheless, 2 reacts with some N-aryl-1,3-azadienes with varying degrees of success (3z4 and 3z5). In these cases, it was imperative to maintain an anhydrous medium. The use of freshly distilled TMO and molecular sieves was paramount. There is no compromise in the E/Z stereoselectivity of the alkene even when trisubstituted alkenes are employed (3j–z). The synthesis of metathesis-suitable bis-allylic 2-oxopyrrolidines such as 3n/w sets the stage for the construction of N-fused bicyclic amines. Halogenated precursors are tolerated (see 3s, 3u, 3y, 3z, and 3z3), which bodes well for late-stage diversification since the halogen group may be utilized as a functional handle for cross-coupling purposes.9b The annulation of 2 with externally substituted 1,3-azadienes derived from β-aryl cinnamaldehydes is possible (see 3z5). Diarylethenes such as 3z5 are prevalent in photoresponsive molecular switches.20
A potentially beneficial aspect of this methodology is the scalable nature of the reactions given that products such as 3b′ have been prepared in over 10 mmol scale, with little to no compromise in efficiency and diastereoselectivity. This has set the stage for the post-diversification studies described henceforth. It is now fully appreciated that both molecular complexity (as measured by Fsp3, where Fsp3 refers to the ratio of sp3 hybridized carbons to the total number of carbons) and the presence of carbon stereocenters correlate with success as compounds transition from discovery, through clinical testing, to drugs.21 Among the many methods for the construction of common-ring lactones, halogen-initiated cyclization of unsaturated carboxylic acids is an attractive and direct approach for the stereoselective synthesis of halogenated lactones.22 Fittingly, our studies have revealed that catalytic bromo- and iodo-lactonization of the allylic lactam acids of type 3′ proceeds regioselectively as well as diastereoselectively (Scheme 2, 5a–j). These bicycles bear four contiguous stereocenters. The stereocontrolled synthesis of tertiary organic halides such as 5f–h is noteworthy given the scarcity of reliable methods for their preparation. Notably, when bis-homoallylic lactam acid 3n′ is employed, iodolactonization is accompanied by carbo-iodination of the N-allyl moiety, leading to the formation of tricycle 6.
 |
| Scheme 2 Regioselective and stereocontrolled halolactonization of lactam-tethered alkenoic acid 3. | |
One of the most succinct approaches to C(sp3)–C(sp3) bond construction is α-enolate alkylation of carbonyl compounds.23 It is well established that due to resonance stabilization, amides have comparably lower α-CH acidities (pKa = 35.0) than other carbonyl-containing compounds such as esters (pKa = 29.5), which renders α-functionalization of amides extremely challenging. Nevertheless, we have explored the amenability of unprotected tertiary γ-lactam alkenols, which are obtainable from 3 by chemoselective nucleophilic addition of Grignard reagents to the ester motif, to diastereoselctive enolate alkylation (Scheme 3). In the event, we find that enolization and concomitant trapping with several carbon-based electrophiles proceeds smoothly to afford the highly customized 5-alkenylated lactams depicted in Scheme 3. Allylic alkylation of the enolates with prenyl-, geranyl-, or allyl bromide furnishes the allylated lactams in satisfactory yields and diastereoselectivities (8a–i). Benzyl electrophiles react prudently in this transformation (8j–p). Methylation with methyl iodide proceeds uneventfully when the enolate is trapped at a colder temperature to prevent bis-methylation and O-methylation of the alcohol (see 8q/r).
 |
| Scheme 3 Scope of diastereoselective α-functionalization of alkenol-tethered γ-lactams. | |
Trapping of the lactam enolate with other potentially problematic alkyl electrophiles such as phenethyl bromide, 11-bromoundecene, and 1-bromononane furnished the desired products in moderate yields and high diastereoselectivities (see 8s–u). Acylation with allyl chloroformate proceeds satisfactorily to afford β-lactam ester 8v, a potentially useful substrate for enantioselective decarboxylative allylation. Propargylation of the transient enolate with propargyl bromide leads to the installation of the terminal alkyne resident in lactam 8w. Allylic lactams harboring a cyclopentenol motif have also been successfully interrogated in this diastereoselective α-functionalization protocol (see 8x–z2). The superiority of tert-butyllithium over conventional lithium amide bases such as LDA and LiHMDS, in lactam enolate alkylations, has previously been noted by Beak and coworkers.24 These α,β,γ-substituted lactams are potential precursors to 2,3,4,5-substituted pyrrolidines given the increasing popularity of methods for the reductive alkylation of lactams.25
Conclusions
In summary, a formal cycloaddition between 1,3-azadienes and succinic anhydride, performed in a sustainable reaction medium, has been developed to facilitate the construction of allylic 2-pyrrolidinones bearing contiguous stereocenters. The annulation reaction shows a relatively broad substrate scope and provides vicinally functionalized allylic γ-lactams in moderate to good yields. The nature of the nitrogen substituent on the 1,3-azadiene component appears to have a profound effect on the reactivity and selectivity given that N-aryl azadienes are problematic. The protocol is chemoselective, diastereoselective, and scalable, thus, providing an opportunity for post-modification by incorporating motifs with either known pharmaceutical value or that permit subsequent conversion to medicinally relevant entities (e.g., halogens, carboxylic acids, esters, alkenes, alkynes, and alcohols). Highly customized bicyclic γ-lactams have been assembled through a DMAP-catalyzed halolactonization protocol. The amenability of these functionalized 2-pyrrolidinones to C–C bond forming transformations, such as enolate alkylation of unprotected alkenol-tethered γ-lactams, bodes well for future late-stage assembly of complex bioactive pyrrolidines and γ-lactams. With the developed method, we anticipate that scientists would be able to use the 1,3-azadiene-succinic anhydride annulation reaction to rapidly generate libraries of new vicinally functionalized 2-oxopyrrolidines in a sustainable manner, while controlling the 3D architecture of the compounds.
Author contributions
T. K. B. – conceptualization, project administration, supervision, investigation, data curation, methodology, writing – original draft, internal and external funding acquisition. J. K. – investigation, data curation, methodology; I. S. A. – investigation, data curation, methodology; B. R. – investigation, data curation, methodology; S. N. – investigation, data curation, methodology.
Conflicts of interest
There are no conflicts of interest to declare.
Acknowledgements
We are grateful to Central Washington University for financial support through startup funds to T. K. B. and for the continuous maintenance of major research instrumentation. The School of Graduate Studies and Research is thanked for partial support of this work through a Faculty Research Award to T. K. B. and for a graduate summer fellowship to I. S. A. The Office of the Provost is acknowledged for research fellowships to J. K. and I. S. A. We thank the Office of University Research (OUR) at CWU for partially funding this project through funding awarded to B. R., J. K., and S. N. We are grateful to Mr Daniel Hall and Miss Lisa Stowe for timely ordering and delivery of chemicals and supplies. We thank Organic Syntheses, Inc. for partial support of this project.
Notes and references
- For Selective Reviews, see:
(a) M. D. McReynolds, J. M. Dougherty and P. R. Hanson, Chem. Rev., 2004, 104, 2239–2258 CrossRef CAS PubMed;
(b) F. Alonso, I. P. Beletskaya and M. Yus, Chem. Rev., 2004, 104, 3079–3159 CrossRef CAS PubMed;
(c) Z. Jakopin and M. S. Dolenc, Curr. Med. Chem., 2010, 17, 651–671 CrossRef CAS PubMed.
-
(a) R. H. Feling, G. O. Buchanan, T. J. Mincer, C. A. Kauffman, P. R. Jensen and W. Fenical, Angew. Chem., Int. Ed., 2003, 42, 355–357 CrossRef CAS PubMed;
(b) L. R. Reddy, P. Saravanan and E. J. Corey, J. Am. Chem. Soc., 2004, 126, 6230–6231 CrossRef CAS PubMed;
(c) R. De Vreese and M. D'Hooghe, Beilstein J. Org. Chem., 2012, 8, 398–402 CrossRef CAS PubMed;
(d) X. Zheng, X.-J. Dai, H.-Q. Yuan, C.-X. Ye, J. Ma and P.-Q. Huang, Angew. Chem., Int. Ed., 2013, 52, 3494 CrossRef CAS PubMed;
(e) K.-Z. Hu, J. Ma, S. Qiu, X. Zheng and P.-Q. Huang, J. Org. Chem., 2013, 78, 1790 CrossRef CAS PubMed;
(f) S. P. Lathrop and T. Rovis, Chem. Sci., 2013, 4, 1668 RSC;
(g) N. Armanino and E. M. Carreira, J. Am. Chem. Soc., 2013, 135, 6814 CrossRef CAS PubMed;
(h) K. L. Kimmel, J. D. Weaver, M. Lee and J. A. Ellman, J. Am. Chem. Soc., 2012, 134, 9058 CrossRef CAS PubMed;
(i) N. Satoh, S. Yokoshima and T. Fukuyama, Org. Lett., 2011, 13, 3028 CrossRef CAS PubMed;
(j) R. A. Shenvi and E. J. Corey, J. Am. Chem. Soc., 2009, 131, 5746 CrossRef CAS PubMed;
(k) T. B. Poulsen, G. Dickmeiss, J. Overgaard and K. A. Jørgensen, Angew. Chem., Int. Ed., 2008, 47, 4687 CrossRef CAS PubMed;
(l) G. Ma, H. Nguyen and D. Romo, Org. Lett., 2007, 9, 2143 CrossRef CAS PubMed;
(m) D. Chauhan, L. Catley, G. Li, K. Podar, T. Hideshima, M. Velankar, C. Mitsiades, N. Mitsiades, H. Yasui, A. Letai, H. Ovaa, C. Berkers, B. Nicholson, T. H. Chao, S. T. Neuteboom, P. Richardson, M. A. Palladino and K. C. Anderson, Cancer Cell, 2005, 8, 407 CrossRef CAS PubMed;
(n) S. Fustero, M. García de la Torre, J. F. Sanz Cervera, C. Ramírez de Arellano, J. Piera and A. Simón, Org. Lett., 2002, 4, 3651 CrossRef CAS PubMed;
(o) A. G. M. Barrett, J. Head, M. L. Smith, N. S. Stock, A. J. P. White and D. J. Williams, J. Org. Chem., 1999, 64, 6005 CrossRef CAS;
(p) E. J. Corey and W.-D. Z. Li, Chem. Pharm. Bull., 1999, 47, 1 CrossRef CAS PubMed;
(q) C. W. G. Fishwick, R. J. Foster and R. E. Carr, Tetrahedron Lett., 1996, 37, 3915 CrossRef CAS.
-
(a) C. Gomez, M. Gicquel, J.-C. Carry, L. Schio, P. Retailleau, A. Voituriez and A. Marinetti, J. Org. Chem., 2013, 78, 1488 CrossRef CAS PubMed;
(b) K.-J. Xiao, A.-E. Wang and P.-Q. Huang, Angew. Chem., Int. Ed., 2012, 51, 8314 CrossRef CAS PubMed;
(c) C. Shao, H.-J. Yu, N.-Y. Wu, P. Tian, R. Wang, C.-G. Feng and G.-Q. Lin, Org. Lett., 2011, 13, 788 CrossRef CAS PubMed;
(d) K.-J. Xiao, J.-M. Luo, K.-Y. Ye, Y. Wang and P.-Q. Huang, Angew. Chem., Int. Ed., 2010, 49, 3037 CrossRef CAS PubMed;
(e) G. Chouhan and H. Alper, Org. Lett., 2008, 10, 4987 CrossRef CAS PubMed;
(f) A. Agosti, S. Britto and P. Renaud, Org. Lett., 2008, 10, 1417 CrossRef CAS PubMed;
(g) A. Gheorghe, M. Schulte and O. Reiser, J. Org. Chem., 2006, 71, 2173 CrossRef CAS PubMed;
(h) T. Okino, Y. Hoashi, T. Furukawa, X. Xu and Y. Takemoto, J. Am. Chem. Soc., 2005, 127, 119 CrossRef CAS PubMed;
(i) S. Madan, P. Milano, D. B. Eddings and R. E. Gawley, J. Org. Chem., 2005, 70, 3066 CrossRef CAS PubMed.
- L. I. Kas'yan, V. A. Pal'chikov and Y. S. Bondarenko, Russ. J. Org. Chem., 2011, 47, 1609–1652 CrossRef.
- S. A. Shuler, G. Yin, S. B. Krause, C. M. Vesper and D. A. Watson, J. Am. Chem. Soc., 2016, 138, 13830–13833 CrossRef CAS PubMed.
-
(a) N. T. Burdzhiev and E. R. Stanoeva, Z. Naturforsch., B: Chem. Sci., 2008, 63, 313–320 CrossRef CAS;
(b) N. Castagnoli Jr, J. Org. Chem., 1969, 34, 3187–3189 CrossRef CAS PubMed.
-
(a) N. Kanbayashi, K. Takenaka, T. Okamura and K. Onitsuka, Angew. Chem., Int. Ed., 2013, 52, 4897 CrossRef CAS PubMed;
(b) M. Hatano and T. Nishimura, Angew. Chem., Int. Ed., 2015, 54, 10949 CrossRef CAS PubMed;
(c) J. L. Panger and S. E. Denmark, Org. Lett., 2020, 22, 2501 CrossRef CAS PubMed;
(d) K. Ashida, Y. Hoshimoto, N. Tohnai, D. E. Scott, M. Ohashi, H. Imaizumi, Y. Tsuchiya and S. Ogoshi, J. Am. Chem. Soc., 2020, 142, 1594 CrossRef CAS PubMed;
(e) H. Qian, S. Sun, W. Zhao and J. Sun, Chem. Sci., 2020, 56, 11295 CAS;
(f) P. V. Ramachandran and T. E. Burghardt, Chem.–Eur. J., 2005, 11, 4387 CrossRef CAS PubMed.
- X. Zhao, D. A. DiRocco and T. Rovis, J. Am. Chem. Soc., 2011, 133, 12466 CrossRef CAS PubMed.
-
(a) H. Braunstein, S. Langevin, M. Khim, J. Adamson, K. Hovenkotter, L. Kotlarz, B. Mansker and T. K. Beng, Org. Biomol. Chem., 2016, 14, 8864–8872 RSC;
(b) T. K. Beng and A. Moreno, New J. Chem., 2020, 44, 4257–4261 RSC;
(c) T. K. Beng, M. Bauder, M. J. Rodriguez and A. Moreno, New J. Chem., 2018, 42, 16451–16455 RSC;
(d) K. Hovenkotter, H. Braunstein, S. Langevin and T. K. Beng, Org. Biomol. Chem., 2017, 15, 1217–1221 RSC;
(e) T. K. Beng and A. Moreno, RSC Adv., 2020, 10, 8805–8809 RSC;
(f) J. Garcia, J. Eichwald, J. Zesiger and T. K. Beng, RSC Adv., 2022, 12, 309–318 RSC;
(g) T. K. Beng, J. Garcia, J. Eichwald and C. Borg, RSC Adv., 2023, 13, 14355–14360 RSC;
(h) T. K. Beng, J. Eichwald, J. Fessenden, K. Quigley, S. Sharaf, N. Jeon and M. Do, RSC Adv., 2023, 13, 21250–21258 RSC;
(i) T. K. Beng, M. Rodriguez and C. Borg, RSC Adv., 2022, 12, 17617–17620 RSC;
(j) T. K. Beng, C. Borg and M. Rodriguez, RSC Adv., 2022, 12, 28685–28691 RSC.
-
(a) T. K. Beng, S. Langevin, H. Braunstein and M. Khim, Org. Biomol. Chem., 2016, 14, 830–834 RSC;
(b) D. P. Bassler, L. Spence, A. Alwali, O. Beale and T. K. Beng, Org. Biomol. Chem., 2015, 13, 2285–2292 RSC;
(c) T. K. Beng, A. W. V. Silaire, A. Alwali and D. P. Bassler, Org. Biomol. Chem., 2015, 13, 7915–7919 RSC;
(d) T. K. Beng, H. TakeuchVi, M. Weber and R. Sarpong, Chem. Commun., 2015, 51, 7653–7656 RSC;
(e) T. K. Beng, N. Fox, D. P. Bassler, A. Alwali, K. Sincavage and A. W. V. Silaire, Org. Biomol. Chem., 2015, 13, 8647–8651 RSC;
(f) T. K. Beng and D. P. Bassler, Tetrahedron Lett., 2014, 55, 6662–6664 CrossRef CAS.
-
(a) N. Guranova, P. Golubev, O. Bakulina, D. Dar'in, G. Kantin and M. Krasavin, Org. Biomol. Chem., 2021, 19, 3829–3833 RSC;
(b) A. Georgieva, E. Stanoeva, S. Spassov, M. Haimova, N. De Kimpe, M. Boelens, M. Keppens, A. Kemme and A. Mishnev, Tetrahedron, 1995, 51, 6099 CrossRef CAS;
(c) A. Georgieva, S. Spassov, E. Stanoeva, I. Topalova and C. J. Tchanev, J. Chem. Res. Synop., 1997, 148 RSC.
-
(a) M. Krasavin and D. Dar'in, Tetrahedron Lett., 2016, 57, 1635–1640 CrossRef CAS;
(b) M. Gonzalez-Lopez and J. T. Shaw, Chem. Rev., 2009, 109, 164–189 CrossRef CAS PubMed;
(c) S. W. Laws, S. Y. Howard, R. Mato, S. Meng, J. C. Fettinger and J. T. Shaw, Org. Lett., 2019, 13, 5073–5077 CrossRef PubMed;
(d) N. Castagnoli and M. Cushman, J. Org. Chem., 1971, 36, 3404–3406 CrossRef CAS PubMed;
(e) J. Wei and J. T. Shaw, Org. Lett., 2007, 9, 4077–4080 CrossRef CAS PubMed;
(f) O. Pattawong, D. Q. Tan, J. C. Fettinger, J. T. Shaw and P. H.-Y. Cheong, Org. Lett., 2013, 15, 5130–5133 CrossRef CAS PubMed.
- J. Robert and A. Boucherle, Ann. Pharm. Fr., 1981, 39, 337–346 CAS.
- D. Dar'in, O. Bakulina, S. Nikolskaya, I. Gluzdikov and M. Krasavin, RSC Adv., 2016, 6, 49411–49415 RSC.
- R. C. Cioc, E. Ruijter and R. V. A. Orru, Green Chem., 2014, 16, 2958 RSC.
- C. M. Alder, J. D. Hayler, R. K. Henderson, A. M. Redman, L. Shukla, L. E. Shuster and H. F. Sneddon, Green Chem., 2016, 18, 3879–3890 RSC.
- F. Byrne, B. Forier, G. Bossaert, C. Hoebers, T. J. Farmer, J. H. Clark and A. J. Hunt, Green Chem., 2017, 19, 3671–3678 RSC.
- A. Pellis, F. P. Byrne, J. Sherwood, M. Vastano, J. W. Comerford and T. J. Farmer, Green Chem., 2019, 21, 1686–1694 RSC.
-
(a) N. Castagnoli, J. Org. Chem., 1969, 34, 3187–3189 CrossRef CAS PubMed;
(b) D. Alonso, A. Costa, B. Mancheno and C. Najera, Tetrahedron, 1997, 53, 4791–4814 CrossRef CAS;
(c) M. Novikov, A. Khlebnikov, E. Sidorina, A. Masalev, J. Kopf, R. Kostikov and Zh. Org, Khim, 2002, 38, 710–719 Search PubMed.
- A. Faulkner, T. van Leeuwen, B. L. Feringa and S. J. Wezenberg, J. Am. Chem. Soc., 2016, 138, 13597–13603 CrossRef CAS PubMed.
- F. Lovering, J. Bikker and C. Humblet, J. Med. Chem., 2009, 52, 6752–6756 CrossRef CAS PubMed.
-
(a) M. Okada, K. Kaneko, M. Yamanaka and S. Shirakawa, Org. Biomol. Chem., 2019, 17, 3747–3751 RSC;
(b) R. Kristianslund, J. E. Tungen and T. V. Hansen, Org. Biomol. Chem., 2019, 17, 3079–3092 RSC;
(c) T. Chen, T. J. Y. Foo and Y.-Y. Yeung, ACS Catal., 2015, 5, 4751–4755 CrossRef CAS;
(d) J. Wong and Y.-Y. Yeung, RSC Adv., 2021, 11, 13564–13570 RSC;
(e) K. Moriyama, M. Kuramochi, S. Tsuzuki, K. Fujii and T. Morita, Org. Lett., 2021, 23, 268–273 CrossRef CAS PubMed;
(f) J. E. Tungen, R. Kristianslund, A. Vik and T. V. Hansen, J. Org. Chem., 2019, 84, 11373–11381 CrossRef CAS PubMed;
(g) Y. A. Cheng, T. Chen, C. K. Tan, J. J. Heng and Y.-Y. Yeung, J. Am. Chem. Soc., 2012, 134, 16492–16495 CrossRef CAS PubMed.
- B. M. Stoltz, N. B. Bennett, D. C. Duquette, A. F. G. Goldberg, Y. Liu, M. M. Loewinger and C. M. Reeves, Compr. Org. Synth., 2014, 3, 1–55 CAS.
- T. A. Johnson, D. O. Jang, B. W. Slafer, M. D. Curtis and P. Beak, J. Am. Chem. Soc., 2002, 124, 11689–11698 CrossRef CAS PubMed.
- P. J. Czerwinski and B. Furman, Front. Chem., 2021, 9, 655849 CrossRef CAS PubMed.
|
This journal is © The Royal Society of Chemistry 2024 |
Click here to see how this site uses Cookies. View our privacy policy here.