DOI:
10.1039/D4RA03170A
(Paper)
RSC Adv., 2024,
14, 20441-20453
Unveiling the influence of end-capped acceptors modification on photovoltaic properties of non-fullerene fused ring compounds: a DFT/TD-DFT study†
Received
29th April 2024
, Accepted 11th June 2024
First published on 27th June 2024
Abstract
Herein, unique A–D–A configuration-based molecules (NBD1–NBD7) were designed from the reference compound (NBR) by utilizing the end-capped acceptor modification approach. Various electron-withdrawing units –F, –Cl, –CN, –NO2, –CF3, –HSO3, and –COOCH3, were incorporated into terminals of reference compound to designed NBD1–NBD7, respectively. A theoretical investigation employing the density functional theory (DFT) and time-dependent DFT (TD-DFT) was performed at B3LYP/6-311G(d,p) level. To reveal diverse opto-electronic and photovoltaic properties, the frontier molecular orbitals (FMOs), absorption maxima (λmax), density of states (DOS), exciton binding energy (Eb), open-circuit voltage (Voc) and transition density matrix (TDM) analyses were executed at the same functional. Moreover, the global reactivity parameters (GRPs) were calculated using the HOMO–LUMO energy gaps from the FMOs. Significant results were obtained for the designed molecules (NBD1–NBD7) as compared to NBR. They showed lesser energy band gaps (2.024–2.157 eV) as compared to the NBR reference (2.147 eV). The tailored molecules also demonstrated bathochromic shifts in the chloroform (671.087–717.164 nm) and gas phases (623.251–653.404 nm) as compared to NBR compound (674.189 and 626.178 nm, respectively). From the photovoltaic perspectives, they showed promising results (2.024–2.157 V). Furthermore, the existence of intramolecular charge transfer (ICT) in the designed compounds was depicted via their DOS and TDM graphical plots. Among all the investigated molecules, NBD4 was disclosed as the excellent candidate for solar cell applications owing to its favorable properties such as the least band gap (2.024 eV), red-shifted λmax in the chloroform (717.164 nm) and gas (653.404 nm) phases as well as the minimal Eb (0.126 eV). This is due to the presence of highly electronegative –NO2 unit at the terminal of electron withdrawing acceptor moiety, which leads to increased conjugation and enhanced the intramolecular charge transfer (ICT) rate. The obtained insights suggested that the designed molecules could be considered as promising materials for potential applications in the realm of OSCs.
Introduction
An efficient approach to address the current energy problem is to utilize renewable and environment friendly energy sources such as solar energy to replace polluting fossil fuels.1 The OSCs have attracted significant attention over inorganic and conventional silicon-based solar cells, owing to their advantages such as mechanical flexibility, low-cost and light-weight.2–5 Some OSCs used fullerene-based acceptors which exhibit considerable advantages with almost 10% power conversion efficiency (PCE).6 Despite the remarkable success attained in fullerene-based OSCs, they encountered various challenges, such as expensive purification, inadequate stability and limited absorption in the visible wavelength range.7,8 In order to overcome these challenges, significant research efforts have been focused on non-fullerene acceptors, particularly non-fullerene small-molecule acceptors (NF-SMAs).8 They offer distinct benefits over fullerene derivatives, such as cost-effectiveness, tunable energy levels and effective absorption of visible light.9
Recent reports have demonstrated the NF-SMAs as robust alternatives to the fullerene acceptors, achieving comparable power conversion efficiency (PCE).10 OSCs utilizing non-fullerene materials made significant advancements, with achieving PCE of more than 13%.11–14 NFAs-based OSCs possess unique properties over the fullerene-based acceptors.15 They can be used to accurately modify properties like optical absorption, energy levels and crystallization ability up to 13%.11–14,16 The heterojunction formed by donor and acceptor moieties serves as the operative basis for organic solar cells (OSCs).17 Hence, PCE of solar cells primarily relies on the characteristics of the acceptor and donor materials.18 As the donor materials have undergone significant development, the current emphasis is on enhancing the efficiency of acceptor moieties to achieve highly efficient OSCs.19 In recent years, significant development is observed in the fused ring NFAs (FR-NFAs), which feature acceptor–donor–acceptor (A–D–A) architecture integrating a ladder-form fused donor core.20,21 Employing this molecular design approach, OSCs harvesting over 18% PCE are developed.5,22
Therefore, in the proposed study a non-fullerene small molecule acceptor i.e., NTIC is utilized having A–D–A configuration with a central hexacyclic naphthalene-(cyclopentadithiophene) donor core and terminal acceptor group i.e., 2-(2,3-dihydro-3-oxo-1-H-inden-1-ylidene) propanedinitrile (INCN).23 In A–D–A type non-fullerene molecules, employing the planar π-extended donor core, such as naphthalene (cyclopentadithiophene), has the potential to enhance photon absorption, leading towards improved short-circuit current density (Jsc), hence making it a promising choice for the proposed research.24 Donor core shows a planar structure which is beneficial for the π-electron delocalization and intramolecular charge transport (ICT).25 This helps to avoid aggregation in the solid form, making it suitable for efficient OSC devices.23
Owing to these facts, NTIC is taken as a parent compound in this research paper. The structural modeling of NTIC into the reference compound (NBR) is accomplished by replacing the sulphur (S) with selenium (Se) atom and 1-methyl-4-hexylbenzene with methyl group to streamline the structure and avoid the computational cost. Consequently, various derivatives, denoted as NBD1–NBD7, are formulated by introducing different acceptor groups at the terminals of the NBR as shown in the Scheme 1. The aim of this research is to examine the influence of various acceptor moieties on the photovoltaic characteristics of the naphthalene-based compound.
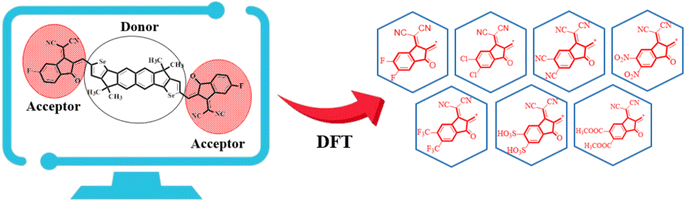 |
| Scheme 1 Schematic illustration of the studied compounds (NBR and NBD1–NBD7). | |
The DFT-based calculations are carried out for NBR and NBD1–NBD7 to investigate their FMOs, UV-Vis spectra, DOS, TDM, Eb, Voc and fill factor (FF). Literature survey has revealed that the modification of the terminal acceptor moieties used to construct different compounds that shows improved photovoltaic and charge transfer properties. The designed molecules are expected to exhibit remarkable photovoltaic features, including minimum band gap, higher light absorption coefficient and elevated charge mobility.
Computational procedure
The present theoretical study was conducted utilizing the Gaussian 09 program26 and visualization of the outcomes were accomplished via the Gauss View 6.0. software.27 In order to select a suitable functional for current study, benchmark study was performed between reported experimental and DFT λmax values at various functionals. For this purpose, the reference compound (NBR) was first optimized at four different DFT functionals such as B3LYP,28 CAM-B3LYP,28 MPW1PW91 (ref. 29) and M06 (ref. 30) combining with 6-311G(d,p) basis set. After the successful optimization of NBR, the UV-visible analysis was conducted at the afore-mentioned functionals. The simulated λmax values at above-mentioned functionals: B3LYP (674.189 nm), CAM-B3LYP (525.799 nm), MPW1PW91 (637.971 nm) and M06 (635.258 nm) were compared with reported experimental (657 nm)23 results to choose an appropriate DFT functional for further investigation. This comparison indicated that B3LYP/6-311G(d,p) level exhibited close harmony with the experimental findings as shown in the Fig. S1† therefore, this functional was selected as the most suitable level for further analyses.
To investigate the photovoltaic and optoelectronic properties of the studied chromophores, their frontier molecular orbitals (FMOs), absorption maxima (λmax), density of states (DOS), exciton binding energy (Eb), open-circuit voltage (Voc), transition density matrix (TDM) and fill factor (FF) analyses were performed at B3LYP/6-311G(d,p) level. The global reactivity parameters (GRPs) were calculated using the energies of the FMOs findings (HOMO–LUMO energies). The following software tools were utilized for interpret the data from outputs: PyMOlyze,31 Origin 8.0 program,32 GaussSum,33 Multiwfn 3.7,34 Chemcraft35 and Avogadro.36
Results and discussion
In the present study, a donor molecule (NTIC) consisting of A–D–A configuration was employed to develop a reference compound (NBR). The NBR undergoes modification by substituting the sulfur atom (S) with selenium atom (Se) in the fused cyclopentadithiophene ring (donor) of NTIC and replacing its larger bulky alkyl groups (–C6H13) with smaller methyl unit (–CH3) to diminish the steric hindrance and to alleviate computational costs (Fig. 1). The reference chromophores (NBR) consist of two parts: central core (hexacyclic naphthalene-(cyclopentadithiophene)) as donor connected with two terminal acceptor moieties (2-(5,6-flouro-3-oxo-2,3-dihydro-1-H-inden-1-y-yildene)propanedinitrile). Various electron withdrawing units –F, –Cl, –CN, –NO2, –CF3, –HSO3, –COOCH3 were incorporated into terminals of reference compound to explore the photovoltaic properties. End-capped acceptors play a pivotal role in the design of high-performance OSCs, particularly in donor–acceptor (D–A) conjugated systems. These electron-deficient moieties, attached to the ends of conjugated donor backbones, extend the π-conjugation and facilitate better charge delocalization. This structural modification allows for precise tuning of the HOMO and LUMO energy levels, optimizing the energy gap and enhancing light absorption. In OSCs, end-capped acceptors enable broader absorption spectra, increasing the generation of photogenerated excitons. They also improve exciton dissociation at the donor–acceptor interface, leading to efficient charge separation and transport. Consequently, the incorporation of end-capped acceptors into the molecular architecture significantly boosts the efficiency and durability of organic photovoltaic devices. By the structure tailoring of NBR, seven new derivatives (NBD1–NBD7) were designed with same configuration as that of the parent and reference compounds (A–D–A). The (A–D–A) configuration, representing acceptor–donor–acceptor, is crucial in photovoltaic devices, such as organic solar cells, for several reasons. It enhances light absorption by broadening the absorption spectrum through molecular tuning of acceptor and donor materials. This structure also creates a strong internal electric field that efficiently separates photo generated electron–hole pairs, reducing recombination losses. Additionally, it provides clear pathways for charge carriers, improving charge mobility and minimizing energy losses. These factors collectively boost key solar cell parameters such as power conversion efficiency (PCE), open-circuit voltage (Voc), short-circuit current density (Jsc), and fill factor (FF), making the A–D–A configuration essential for high-performance solar cells.27
 |
| Fig. 1 Conversion of NTIC into NBR by (I) replacement of thiophene with selenophene and (II); replacement of hexyl group with methyl group. | |
All the designed compounds contain hexacyclic naphthalene-(cyclopentadithiophene) core paired with seven distinct acceptor moieties; 2-(5,6-diflouro-3-oxo-2,3-dihydro-1-H-inden-1-y-yildene)propanedinitrile (NBD1), 2-(5,6-dichloro-3-oxo-2,3-dihydro-1-H-inden-1-y-yildene)propanedinitrile (NBD2), 2-(5,6-dicyano-3-oxo-2,3-dihydro-1-H-inden-1-y-yildene)propanedinitrile (NBD3), 2-(5,6-dinitro-3-oxo-2,3-dihydro-1-H-inden-1-y-yildene)propanedinitrile (NBD4), 2-(5,6-bis(trifluoromethyl)-3-oxo-2,3-dihydro-1-H-inden-1-y-yildene)propanedinitrile (NBD5), 2-(5,6-disulfo-3-oxo-2,3-dihydro-1-H-inden-1-y-yildene)propanedinitrile (NBD6) and 2-(5,6- bis(methoxycarbonyl)-3-oxo-2,3-dihydro-1-H-inden-1-y-yildene)propanedinitrile (NBD7) attached to it. The Fig. 2 displays their chemical structures, while the optimized geometries obtained via the DFT analysis are represented in the Fig. S2.† However, their Cartesian coordinates are provided in the Tables S1–S8 (ESI).†
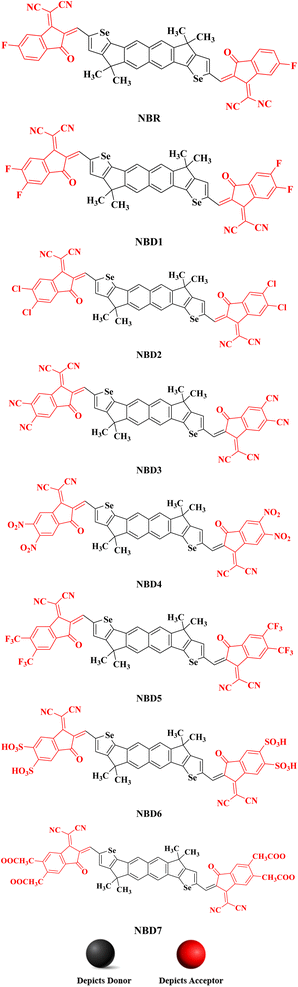 |
| Fig. 2 The chemical structures of NBR and NBD1–NBD7 compounds. | |
Frontier molecular orbitals (FMOs)
Frontier molecular orbitals (FMOs) study is an efficient approach to characterize the photovoltaic properties of the studied molecules. The FMOs diagrams facilitate the understanding of electronic density and the charge distribution pattern of HOMO and LUMO in a molecule.37 The HOMO (valence band) serves as an electron donor, while the LUMO (conduction band) acts as an electron acceptor.38,39 Effective charge transmission within a molecule requires the transfer of electron density from HOMO to LUMO.40 FMOs diagrams of reference (NBR) and tailored compounds (NBD1–NBD7) at B3LYP/6-311G(d,p) level are presented in the Fig. 3. The energy difference between HOMO and LUMO represents the band gap (ΔE = EHOMO − ELUMO).41 The energy gap (ΔE) is crucial in evaluating stability, strength, hardness, softness and chemical reactivity of a molecule.42 Moreover, it has a significant impact on the working efficiency of an OSC. The efficiency of OSCs increases with a smaller band gap and conversely decreases with a larger band gap.43 The charge carrier mobility in designed molecules (NBD1–NBD7) is enhanced by introducing electron withdrawing acceptor moieties. The energy data of HOMO/LUMO orbitals obtained from the FMOs analysis of derivatives are given in the Table 1. Whereas, the HOMO+1/LUMO−1 and HOMO+2/LUMO−2 values along with their visual representation are recorded in the Table S9 and Fig. S3.†
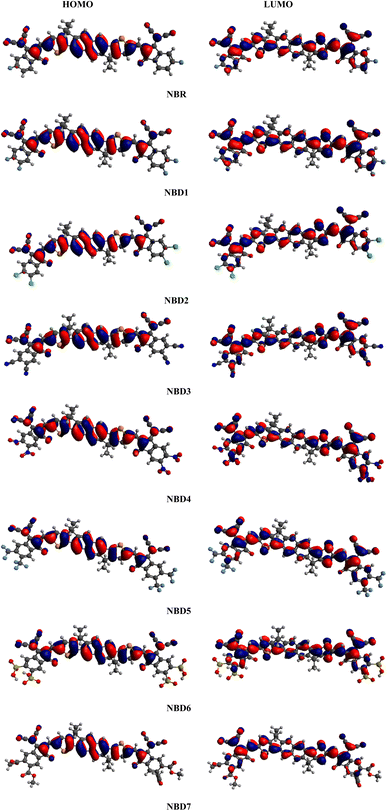 |
| Fig. 3 Frontier molecular orbital contour plots for the reference (NBR) and designed molecules (NBD1–NBD7). | |
Table 1 Energies of the frontier molecular orbitals of the studied compounds in eV
Compounds |
EHOMO |
ELUMO |
ΔE |
NBR |
−5.839 |
−3.692 |
2.147 |
NBD1 |
−5.873 |
−3.716 |
2.157 |
NBD2 |
−5.906 |
−3.776 |
2.130 |
NBD3 |
−6.059 |
−4.014 |
2.045 |
NBD4 |
−6.077 |
−4.053 |
2.024 |
NBD5 |
−5.987 |
−3.887 |
2.100 |
NBD6 |
−6.078 |
−4.034 |
2.044 |
NBD7 |
−5.908 |
−3.785 |
2.123 |
For NBR, the HOMO/LUMO energy values are obtained as −5.839 eV and −3.692 eV, accordingly, resulting in a band gap of 2.147 eV, slightly larger than that observed in its derivatives except NBD1. This band gap showed harmony with reported experimental value (1.82 eV)23 indicating the suitable selection of functional. Calculated HOMO energies of (NBD1–NBD7) are obtained as −5.873, −5.906, −6.059, −6.077, −5.987, −6.078 and −5.908 eV, respectively while LUMO energies of these molecules are −3.716, −3.776, −4.014, −4.053, −3.887, −4.034, and −3.785 eV, correspondingly. Consequently, the band gap ΔE values for the studied derivative molecules (NBD1–NBD7) are found to be 2.157, 2.13, 2.045, 2.024, 2.100, 2.044, and 2.123 and 4.21 eV. Literature reveals that the naphthalene based compounds with A–D–A configuration demonstrates the excellent results of band gap as compared to the A–D–A configured indacenodithiophene-based acceptor chromophores ranging from (2.245–2.070 eV), indicating more effective structural modifications.44 Among all, NBD4 exhibits the least ΔE value as compared to the reference and other designed molecules. This might be owing to the presence of electron withdrawing nitro group (–NO2) at the end of acceptor moiety (2-(5,6-dinitro-3-oxo-2,3-dihydro-1-H-inden-1-y-yildene)propanedinitrile). Furthermore, this decrease in ΔE can also be attributed to the phenomenon of negative inductive effect (−I) and prolonged conjugation within molecule. The prolonged conjugation in aromatic rings results in a significant charge transference from donor to acceptor moieties. Additionally, the energies and HOMO/LUMO band gap of NBD4 that showed least band gap among all designed derivatives at B3LYP functional was also investigated at PBE1PBE/6-311 G (d,p) functional. The comparative study between PBE1PBE/6-311 G (d,p) and B3LYP functionals showed that the ΔE value of NBD4 determined at PBE1PBE functional is 2.292 eV, higher than the ΔE value of 2.024 eV obtained at B3LYP functional.
Moreover, ΔE value of NBD1 is reported as 2.157 and this might be due to the incorporation of fluorine (–F) atoms at the terminal acceptors (2-(5,6-diflouro-3-oxo-2,3-dihydro-1-H-inden-1-y-yildene)propanedinitrile), which showed positive inductive effect (+I) and causes steric hindrance. Accordingly, second narrow band gap is seen in the case of NBD3 and NBD6, probably attributed to the existence of efficient electron withdrawing substituents such as: –CN and –SO3H group at the terminal end of acceptor units (2-(5,6-dicyano-3-oxo-2,3-dihydro-1-H-inden-1-y-yildene)propanedinitrile) and (2-(5,6-disulfo-3-oxo-2,3-dihydro-1-H-inden-1-y-yildene)propanedinitrile) respectively. The –CN and –SO3H groups attracted more electrons towards itself, causes an extended conjugation and increased the charge carrier mobility. Similarly, NBD2, NBD5, and NBD7 also exhibits smaller band gap than NBR, but slightly larger than other derivatives, owing to the lesser resonance effect in the molecule. The decreasing order of ΔE value for the reference and all derivatives are: NBD1 > NBR > NBD2 > NBD7 > NBD5 > NBD3 > NBD6 > NBD4. From above discussion, it is found that our designed molecules showing remarkable performance in comparison to the reference molecule.45
Chemical reactivity parameters (CRPs)
The HOMO–LUMO band gap is utilized to compute the global reactivity parameters (GRPs) such as: ionization potential (IP),46 chemical potential (μ),47 electron affinity (EA), electronegativity (X),48 global electrophilicity index (ω),49 global hardness (η),50 global softness (σ)51 and charge transfer index (ΔNmax).52 Koopmans' theorem53 is widely used for the calculation of these parameters by employing the eqn (S1)–(S8),† and the outcomes of these parameters are presented in the Table 2.
Table 2 Calculated GRPs for the studied compounds (NBR and NBD1–NBD7)
Compounds |
IP |
EA |
X |
η |
μ |
ω |
σ |
ΔNmax |
NBR |
5.839 |
3.692 |
4.766 |
1.074 |
−4.766 |
10.57 |
0.466 |
4.4376 |
NBD1 |
5.873 |
3.716 |
4.795 |
1.079 |
−4.795 |
10.66 |
0.464 |
4.4439 |
NBD2 |
5.906 |
3.776 |
4.841 |
1.065 |
−4.841 |
11.01 |
0.469 |
4.5455 |
NBD3 |
6.059 |
4.014 |
5.037 |
1.023 |
−5.037 |
12.41 |
0.489 |
4.9237 |
NBD4 |
6.078 |
4.053 |
5.065 |
1.012 |
−5.065 |
12.66 |
0.494 |
5.0049 |
NBD5 |
5.987 |
3.887 |
4.937 |
1.050 |
−4.937 |
11.61 |
0.476 |
4.701 |
NBD6 |
6.077 |
4.034 |
5.056 |
1.022 |
−5.056 |
12.51 |
0.489 |
4.9471 |
NBD7 |
5.908 |
3.785 |
4.847 |
1.062 |
−4.847 |
11.06 |
0.471 |
4.5640 |
The IP and EA represents the electron donating and electron accepting properties of molecules, respectively.54 The higher values of IP (6.078–5.873 eV) and EA (4.053–3.716 eV) of designed derivatives (NBD1–NBD7) as compared to NBR (5.839, 3.692 eV, respectively), showed the greater charge transference between donor and acceptor moieties. The decreasing order for both IP and EA values are: NBD4 > NBD6 > NBD3 > NBD5 > NBD7 > NBD2 > NBD1 > NBR.
There exists a close relationship between the GRPs and energy gaps.55 Higher kinetic stability of a molecule correlates with a larger energy band gap between HOMO/LUMO.56 Chemical potential, global electrophilicity, softness and hardness play a pivotal role in influencing the reactivity, stability and polarizability rate of molecules. Molecules with a narrow band gap may be regarded as soft, chemically reactive, less stable and vice versa. All the designed molecules elucidate greater softness (σ) and lower hardness (η) values than reference molecule which indicates higher reactivity with high polarizing power. The increasing order of σ for the titled compounds is as follows: NBD1 (0.464) < NBR (0.466) < NBD2 (0.469) < NBD7 (0.471) < NBD5 (0.476) < NBD3 (0.489) < NBD6 (0.489) < NBD4 (0.494) in eV−1. For η, the following decreasing order is observed: NBD1 (1.079) > NBR (1.074) > NBD2 (1.065) > NBD7 (1.062) > NBD5 (1.050) > NBD3 (1.023) > NBD6 (1.022) > NBD4 (1.012) in eV. Moreover, the molecules with more negative of chemical potential (μ) and high global electrophilicity (ω) value are less stable and more reactive. It is concluded from the above discussion that the compound (NBD4) is anticipated to be the most favorable molecule due to its highest softness value (0.494 eV−1) and lowest hardness (1.012 eV), which aligns with its lowest energy gap (2.024 eV). The comparative study of global hardness and softness at above mentioned both functionals showed that NBD4 at PBE1PBE level exhibits a lower global softness value (0.436 eV−1) and a higher global hardness value (1.146 eV) compared to the results from the B3LYP functional (softness = 0.494 eV−1 and lower hardness 1.012 eV).
Density of states (DOS)
The examination of DOS provides further insight into the distribution of electron density. It describes distribution pattern around HOMO and LUMO which is affected by the nature of electron-withdrawing acceptor moieties. Each structure is divided into two segments for DOS interpretation: the acceptor and the donor.57 The results of DOS analysis are studied by PyMolyze software. Here, the acceptor contributes: 30.0, 29.7, 30.4, 31.5, 31.5, 30.8, 31.5 and 30.5% to HOMO and 61.1, 60.0, 60.5, 65.0, 69.6, 61.7, 65.7 and 61.1% to LUMO for NBR and NBD1–NBD7, respectively. Similarly, the donor contributes 70.0, 70.3, 69.6, 68.5, 68.5, 69.2, 68.5 and 69.5% to HOMO, while 38.9, 40.0, 39.5, 35.0, 30.4, 38.3, 34.3 and 38.9% to LUMO for NBR and NBD1–NBD7, accordingly as shown in the Table S12.†
The DOS graphs represent each part of the molecule in a distinct color i.e., relative intensity of donor is represented in green, while the relative intensity of acceptors is denoted in red color as shown in the Fig. 4. In DOS graphs, the positive values signify LUMO, while, the negative values depict HOMO along the x-axis. In the reference molecule (NBR), the HOMO exhibits electron density distributed across the entire molecule, with a slightly higher concentration on the donor groups. Conversely, the LUMO primarily features electron density concentrated on the acceptor moiety, although there is also a portion of electron density associated with the donating groups. In contrast, in the designed compounds (NBD1–NBD7), the HOMO exhibits higher electron density on the cyclopentadithiophene (donor group) and lower density on the acceptor portion. Conversely, the LUMO displays greater electron density on the acceptor group and lesser density on the donor part. These findings demonstrate the end-capped acceptor moieties are satisfactorily proficient in terms of withdrawing effect.
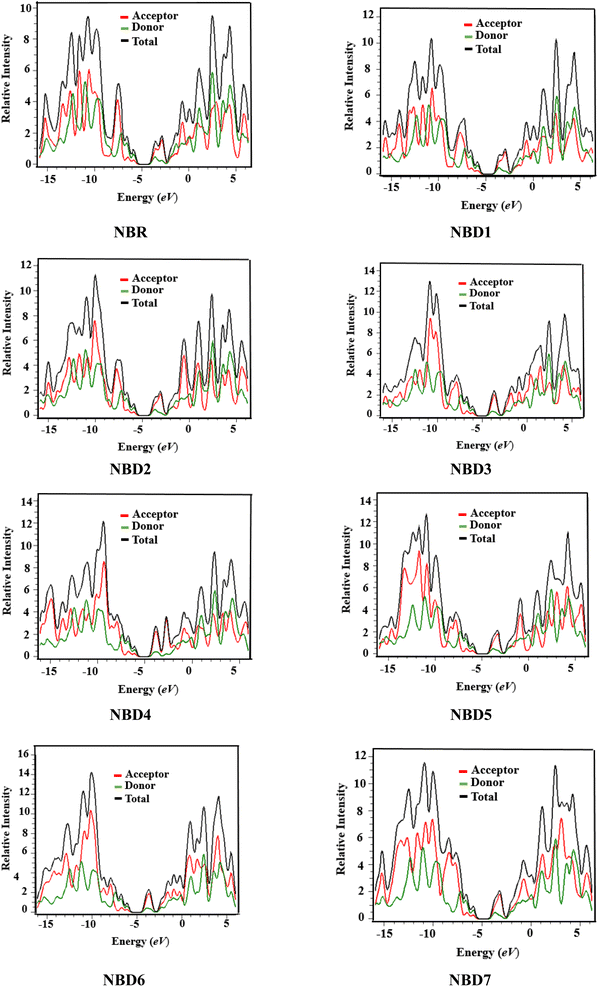 |
| Fig. 4 Graphical representation of density of states (DOS) of NBR and NBD1–NBD7. | |
Absorption properties
UV-Vis analysis is used for determining the nature of transitions and charge-transfer characteristics of a molecule.58 The photovoltaic properties have correlation with the excitation energy (E), oscillation strength (fos), dipole moment and absorption maxima (λmax).59,60 The value of λmax describes the exact energy of photon required for the excitation of an electron from the HOMO towards LUMO, fos represents the possibility of transition, while the E refers to the energy necessary for a transition to occur.61 Therefore, broader absorption at a higher λmax, high fos and low excitation energy are anticipated to yield efficient intramolecular charge transfer (ICT).62 The absorption spectra of reference molecule (NBR) and designed compounds (NBD1–NBD7) are calculated in both the gaseous and chloroform solvent phases. The representative values of λmax along with their corresponding absorption parameters such as transition energy (E), oscillator strength (fos) and major molecular orbitals contributions are shown in the Table 3. While, the remaining values are recorded in the supplementary part (Tables S10 and S11†). The visual representation of the UV-Vis absorption spectra in both media is shown in the Fig. 5.
Table 3 Wavelength (λmax), excitation energy (E), oscillator strength (fos) and major molecular orbital assessments of the titled compounds (D1–D7) in the chloroform solvent and gaseous phases
|
Compounds |
DFT λmax (nm) |
E (eV) |
fos |
Major MO assessment (%) |
Chloroform solvent. Gas phase. |
aPhase |
NBR |
674.189 |
1.839 |
2.734 |
H → L (98%) |
NBD1 |
671.087 |
1.848 |
2.768 |
H → L (98%) |
NBD2 |
681.827 |
1.818 |
2.871 |
H → L (98%) |
NBD3 |
709.937 |
1.746 |
2.677 |
H → L (98%) |
NBD4 |
717.164 |
1.729 |
2.357 |
H → L (98%) |
NBD5 |
689.562 |
1.798 |
2.735 |
H → L (99%) |
NBD6 |
710.099 |
1.746 |
2.598 |
H → L (98%) |
NBD7 |
683.480 |
1.814 |
2.848 |
H → L (98%) |
bPhase |
NBR |
626.178 |
1.980 |
2.476 |
H → L (99%) |
NBD1 |
623.251 |
1.989 |
2.517 |
H → L (99%) |
NBD2 |
632.149 |
1.961 |
2.646 |
H → L (99%) |
NBD3 |
652.132 |
1.901 |
2.545 |
H → L (99%) |
NBD4 |
653.404 |
1.898 |
2.394 |
H → L (99%) |
NBD5 |
637.053 |
1.946 |
2.531 |
H → L (99%) |
NBD6 |
652.372 |
1.901 |
2.471 |
H → L (99%) |
NBD7 |
632.149 |
1.961 |
2.663 |
H → L (99%) |
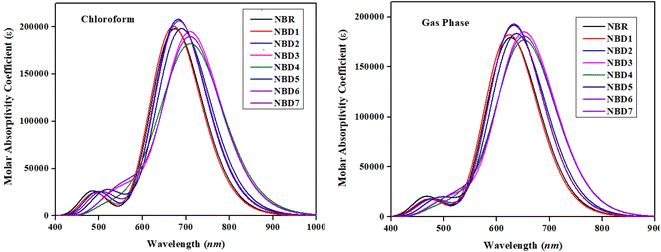 |
| Fig. 5 UV-visible spectra of NBR and NBD1–NBD7 in chloroform and gaseous phase. | |
All the designed derivatives (NBD1–NBD7) exhibited higher maximum absorption values (λmax) as compared to the reference (NBR) which might be attributed to the mutual effect of auxochromes and chromophores incorporated in the NBD1–NBD7 compounds. Moreover, the absorption shift towards longer wavelengths is more prominent in case of the chloroform solvent which indicated that polarity induces the enhanced charge generation capacity. The values of λmax of NBD1–NBD7 compounds in the solvent chloroform and gas phase are noted in the range of 671.087–717.164 nm and 623.25–653.404 nm, accordingly. Whereas, the λmax for reference (NBR) compound in solvent and gas phases are 674.189 and 626.178 nm, respectively. The absorption maxima (λmax) of NBD1–NBD7 in solvent phase are found in the following decreasing order: NBD4 (717.164) > NBD6 (710.099) > NBD3 (709.937) > NBD5 (689.562) > NBD7 (683.480) > NBD2 (681.827) > NBR (674.189) > NBD1 (671.087) in nm. Similarly, the following absorption maxima trend is seen in gas phase: NBD4 (653.404) > NBD6 (652.372) > NBD3 (652.132) > NBD5 (637.053) > NBD7 (632.149) > NBD2 (632.149) > NBR (626.178) > NBD1 (623.251) in nm. Moreover, the investigated molecules (NBR and NBD1–NBD7) exhibit lower corresponding excitation energies (E) as 1.839, 1.848, 1.818, 1.746, 1.729, 1.798, 1.746 and 1.814 eV, respectively, in the chloroform and 1.980, 1.989, 1.961, 1.901, 1.898, 1.946, 1.901 and 1.961 eV in the gas phase, respectively.
Among all the designed molecules, NBD4 exhibits the highest λmax in both media (717.164 nm in the chloroform and 653.404 nm in the gas) with lower excitation energy values of 1.729 eV (in chloroform) and 1.898 eV (in gas phase). This might be due to the presence of –NO2 group at the terminal acceptor moiety which has strong resonating electron withdrawing effect.63 Furthermore, its lower excitation energy leads to an increased charge transference from the HOMO to LUMO. Whereas, NBD1 exhibits the lowest λmax of 671.087 nm (in solvent chloroform) and 623.251 nm (in gas phase), with the highest excitation energy (E) as 1.848 eV (in solvent) and 1.989 eV (in gas phase). From literature, Asif et al. investigated the optical properties of naphtho-dithiophene based non-fullerene acceptor molecule. The results demonstrates that the absorption maximum λmax for designed acceptor molecules (NDT1–NDT4) is 430.2 nm, 449.8 nm, 473.9 nm, and 444.9 nm, respectively. While the current research features broader and longer wavelength absorption ranging from (671.087–717.164 nm) demonstrate excellent optical properties. Therefore, it is anticipated that all the designed compounds exhibit significant optical properties, with high efficiency at low excitation energies in the absorption spectrum.
Transition density matrix (TDM) and exciton binding energy (Eb)
The TDM is employed to estimate and analyze the electronic charge transfer in the excited state. It helps to understand the nature of transitions, hole–electron localization and de-localization as well as the interaction of donor and acceptor units.64–66 It offers a comprehensive insights into electronic transitions taking place within a photovoltaic material.67 TDM plots for transitions in the first excited state (S1) are represented in the Fig. 6.
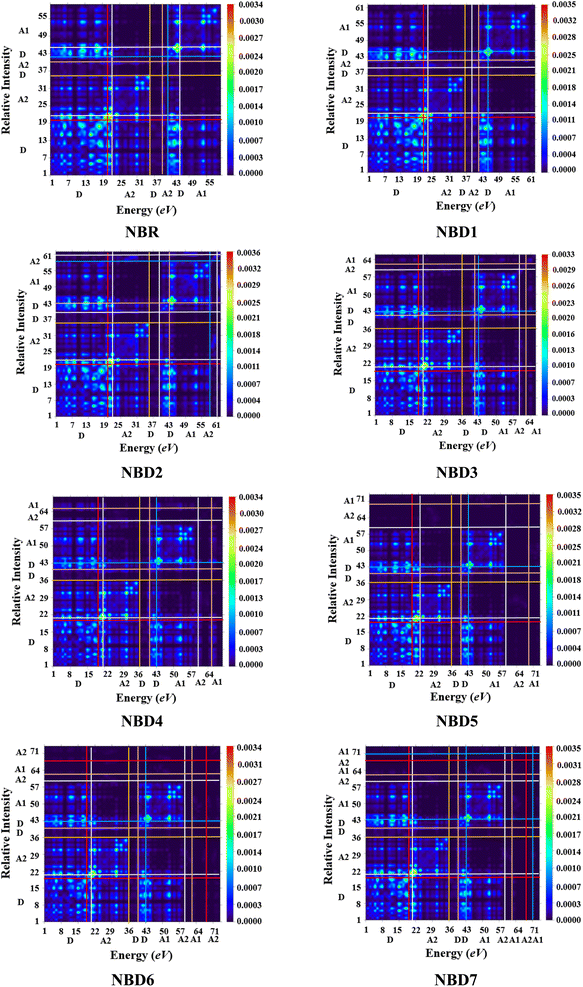 |
| Fig. 6 Transition density matrix plots of NBR and NBD1–NBD7 at S1 state. | |
The hydrogen atoms are mainly neglected by default, due to their minimal contribution to transitions. The investigated molecules are divided into two parts: acceptor (A) and donor (D) whose electron coherence regions are separated by the horizontal and vertical lines. The plots illustrated the accumulation of holes and electrons of the exciton in donor and acceptor part. The electron density in the reference molecule (NBR) is mostly present on the diagonal of the donor part. Whereas, in all the designed compounds (NBD1–NBD7), the distribution of electronic density is mainly concentrated on both the acceptor and donor moieties (mostly on the acceptor part) along diagonal and off-diagonal portions, facilitating the efficient charge transference from donor to acceptor moiety. This efficient charge transfer phenomenon is owed to the prolonged conjugation of utilized acceptor moieties.
Binding energy (Eb) significantly influences the photovoltaic properties, excited separation rate and efficiency of the OSCs.67 The Eb represents the energy needed to dissociate excitons into free charge carriers.68 It is performed to calculate the coulombic force of interaction between electrons and holes in a molecule. Also, it is directly proportional to the band gap (EH–L) and coulombic interaction between electrons and holes and inversely proportional to the exciton dissociation rate.69 The lower the binding energy (Eb) and coulombic interactions, the higher will be the dissociation rate in excited state and vice versa. The values for Eb are calculated by using the eqn (1).70
where,
EH–L demonstrates the HOMO/LUMO energy gap and
Eopt is minimum energy needed for transition from the ground state (S
0) to excited state (S
1). The
Eb values for reference and all the designed chromophores are presented in the
Table 4.
NBD4 exhibits the lowest
Eb (0.126 eV) as compared to
NBR reference and all other designed compounds, whereas
NBD2 displays the highest binding energy (0.169 eV). Therefore,
NBD4 shows the maximum dissociation potential due to weaker coulombic interactions between electron and hole. The decreasing order of binding energy in eV is:
NBD2 (0.169) >
NBD1 (0.168) >
NBR (0.167) >
NBD7 (0.162) >
NBD5 (0.154) >
NBD3 (0.144) >
NBD6 (0.143) >
NBD4 (0.126). Based on the above discussion, it is inferred that all the designed derivatives (excluding
NBD1 and
NBD2) demonstrate lesser binding exciton energies and greater charge separation.
Table 4 Calculated binding energy (Eb) of titled compoundsa
Compounds |
EH–L |
Eopt |
Eb |
Units in eV. |
NBR |
2.147 |
1.980 |
0.167 |
NBD1 |
2.157 |
1.989 |
0.168 |
NBD2 |
2.13 |
1.961 |
0.169 |
NBD3 |
2.045 |
1.901 |
0.144 |
NBD4 |
2.024 |
1.898 |
0.126 |
NBD5 |
2.1 |
1.946 |
0.154 |
NBD6 |
2.044 |
1.901 |
0.143 |
NBD7 |
2.123 |
1.961 |
0.162 |
Open circuit voltage (Voc) and fill factor (FF)
Open circuit voltage (Voc) is one of the important parameters to estimate the efficiency of OSCs.47 It refers to the maximum voltage produced by the photovoltaic devices to the external circuit when operating at zero current. In OSCs, electricity is produced at the donor of HOMO, transferring electrons to the acceptor of LUMO.71 Hence, the Voc value primarily depends on the energy levels of the LUMO and HOMO of acceptor and donor, respectively.72 The higher value of HOMO and the lower value of LUMO should be required to attain high device performance.20 Moreover, the Voc is in direct relation with HOMO–LUMO band gap between the designed molecules and the polymer. The higher the band gap between HOMO and LUMO, higher will be the Voc value. In organic photovoltaic (OPV) devices, the open circuit voltage (Voc) is influenced by the HOMO–LUMO band gap of the donor and acceptor materials. As the band gap decreases, the energy difference between the donor's HOMO and the acceptor's LUMO increases, potentially raising the Voc. This reduction in band gap also allows absorption of a broader spectrum of sunlight, potentially increasing the short circuit current density (Jsc) by generating more charge carriers.73
Furthermore, the HOMO/LUMO energy gap of the acceptor and donor units directly increases the PCE values. In this study, the PC71BM acceptor polymer is chosen for the calculation of Voc of the donor-type designed compounds owing to its confirmed effective charge transference from the donor to acceptors. The Voc of the reference compound (NBR) and designed derivatives (NBD1–NBD7) is computed by using the Scharber's Equation.18 According to this eqn (2), difference between the HOMO of donor (NBD1–NBD7) and the LUMO of acceptor (PC71BM) denotes the Voc20 subtracting 0.3 (an empirical factor).The Voc values of all the titled molecules are presented in the Table 5, while their visual representation is displayed in the Fig. 7.
|
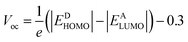 | (2) |
Table 5 Open-circuit voltage (Voc) of the entitled compoundsa
Compounds |
Voc (V) |
ΔE |
ΔE = EALUMO − EDHOMO. |
NBR |
2.109 |
1.959 |
NBD1 |
2.143 |
2.443 |
NBD2 |
2.176 |
2.476 |
NBD3 |
2.329 |
2.629 |
NBD4 |
2.347 |
2.647 |
NBD5 |
2.257 |
2.557 |
NBD6 |
2.348 |
2.648 |
NBD7 |
2.178 |
2.478 |
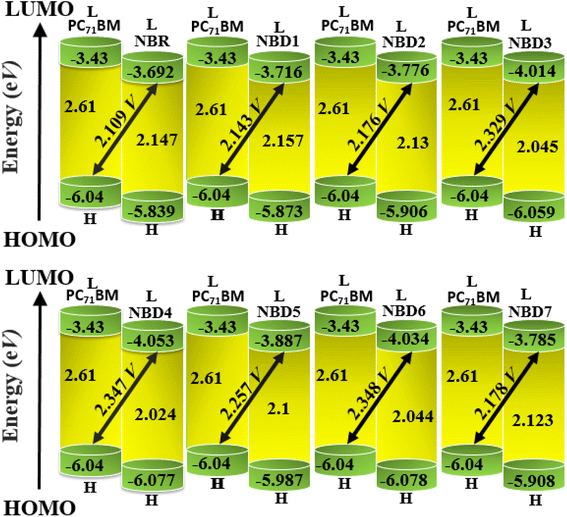 |
| Fig. 7 Graphical representation of Voc for designed molecules with PC71BM. | |
The results demonstrate that all the tailored compounds (NBD1–NBD7) exhibit greater Voc values as compared to NBR reference, owing to their higher HOMO energy values. Notably, NBD6 and NBD4 exhibit the highest Voc values of 2.348 and 2.347 V, respectively due to efficient terminal acceptor moieties with planar geometries which allow the ultimate charge transference from donor to acceptor and enhance the conjugation. Therefore, NBD4 emerges as the optimal choice for solar cell applications. Its electron-pulling acceptor group, enhanced by the highly electronegative –NO2 attachment, results in excellent photophysical, electronic, and photovoltaic properties compared to all other derivatives. A decreasing order of Voc values for all the studied compounds in V is as follows: NBD6 (2.348) > NBD4 (2.347) > NBD3 (2.329) > NBD5 (2.257) > NBD7 (2.178) > NBD2 (2.176) > NBD1 (2.143) > NBR (2.109).
Fill factor (FF) significantly influences PCE of organic photovoltaic (PV) devices.74 It primarily relies on the open-circuit voltage value.75 Higher Voc values enhance the fill factor, significantly contributing to the system's efficiency.76 It can be computed by using the following eqn (3).77
|
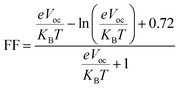 | (3) |
where
KB,
T, and
e represent the Boltzmann's constant (8.61733034 × 10
5), temperature (298 K), and the elementary charge (fixed at 1), respectively. The computed values listed in Table S13
† reveal high FF indicating promising PCE.
Conclusion
In conclusion, a quantum chemical study is performed on the newly designed naphthalene-based molecules (NBD1–NBD7) to explore their optoelectronic, photo-physical and photovoltaic properties. It is noteworthy to discuss that the molecular engineering is performed by incorporating the efficient electron-withdrawing units in NBR which significantly improves the photovoltaic characteristics of the designed derivatives (NBD1–NBD7). They showed reduced energy gap (2.024–2.157 eV) and broadened optical absorption in chloroform (671.087–717.164 nm) and gas phase (623.251–653.404 nm). Further, all derivatives showed higher Voc values compared to NBR which are calculated via the HOMOdonor and polymer LUMOPC71BM. Exploring the photovoltaic properties more particularly, it is reported that NBD4 is potent among all the designed molecules as it exhibits the maximum absorbance at 717.164 nm (chloroform solvent) and 653.404 nm (gas phase) with lowest binding energy value of 0.126 eV. Moreover, the efficient charge transfer from the HOMO of naphthalene-based donor to the LUMO of the end-capped acceptor units is demonstrated via the results of FMOs, DOS and TDM analyses. The results indicate that the designed NFAs-based compounds (NBD1–NBD7) are potential candidates for the next-generation photovoltaic devices.
Data availability
All data generated or analyzed during this study are included in this published article and its ESI files.†
Conflicts of interest
There are no conflicts to declare.
Acknowledgements
Dr Muhammad Khalid gratefully acknowledges the financial support of HEC Pakistan (project no. 20-14703/NRPU/R&D/HEC/2021). A. A. C. B. acknowledges the financial support of the São Paulo Research Foundation (FAPESP) (Grant 2015/01491-3), the Conselho Nacional de Desenvolvimento Científico e Tecnologico (CNPq) of Brazil for academic support (Grant 313720/2023-1), and Coordenação de Aperfeiçoamento de Pessoal de Nivel Superior – Brasil (CAPES) that partially supported this work (Finance code 001). The authors thank the Researchers Supporting project number (RSP2024R6), King Saud University, Riyadh, Saudi Arabia.
References
- F. Abbas, U. Ali, H. M. R. Ahmad, A. Tallat, A. Shehzad, Z. Zeb, I. Hussain and A. Saeed, Opt. Quantum Electron., 2022, 54, 1–15 CrossRef.
- S. Günes, H. Neugebauer and N. S. Sariciftci, Chem. Rev., 2007, 107, 1324–1338 CrossRef PubMed.
- G. Li, R. Zhu and Y. Yang, Nat. Photonics, 2012, 6, 153–161 CrossRef CAS.
- H. J. Son, B. Carsten, I. H. Jung and L. Yu, Energy Environ. Sci., 2012, 5, 8158–8170 RSC.
- Q. Liu, Y. Jiang, K. Jin, J. Qin, J. Xu, W. Li, J. Xiong, J. Liu, Z. Xiao, K. Sun, S. Yang, X. Zhang and L. Ding, Sci. Bull., 2020, 65, 272–275 CrossRef CAS PubMed.
- J. Zhao, Y. Li, H. Lin, Y. Liu, K. Jiang, C. Mu, T. Ma, J. Y. L. Lai, H. Hu, D. Yu and H. Yan, Energy Environ. Sci., 2015, 8, 520–525 RSC.
- L. Nian, Y. Kan, K. Gao, M. Zhang, N. Li, G. Zhou, S. B. Jo, X. Shi, F. Lin, Q. Rong, F. Liu, G. Zhou and A. K.-Y. Jen, Joule, 2020, 4, 2223–2236 CrossRef CAS.
- J. Zhang, H. S. Tan, X. Guo, A. Facchetti and H. Yan, Nat. Energy, 2018, 3, 720–731 CrossRef CAS.
- D. Luo, W. Jang, D. D. Babu, M. S. Kim, D. H. Wang and A. K. K. Kyaw, J. Mater. Chem. A, 2022, 10, 3255–3295 RSC.
- I. Shafiq, U. Shoukat, M. A. Asghar, M. Aslam, T. Ahamad, S. Ahmed, S. Bullo and S. C. Ojha, J. Saudi Chem. Soc., 2024, 28, 101799 CrossRef CAS.
- J. Yuan, Y. Zhang, L. Zhou, G. Zhang, H.-L. Yip, T.-K. Lau, X. Lu, C. Zhu, H. Peng, P. A. Johnson, M. Leclerc, Y. Cao, J. Ulanski, Y. Li and Y. Zou, Joule, 2019, 3, 1140–1151 CrossRef CAS.
- Y. Cui, H. Yao, L. Hong, T. Zhang, Y. Xu, K. Xian, B. Gao, J. Qin, J. Zhang, Z. Wei and J. Hou, Adv. Mater., 2019, 31, 1808356 CrossRef PubMed.
- K. Gao, S. B. Jo, X. Shi, L. Nian, M. Zhang, Y. Kan, F. Lin, B. Kan, B. Xu, Q. Rong, L. Shui, F. Liu, X. Peng, G. Zhou, Y. Cao and A. K.-Y. Jen, Adv. Mater., 2019, 31, 1807842 CrossRef PubMed.
- Z. Zhou, S. Xu, J. Song, Y. Jin, Q. Yue, Y. Qian, F. Liu, F. Zhang and X. Zhu, Nat. Energy, 2018, 3, 952–959 CrossRef CAS.
- I. Shafiq, A. Mustafa, R. Zahid, R. Baby, S. Ahmed, M. A. Asghar, T. Ahamad, M. Alam, A. A. Braga and S. C. Ojha, ACS Omega, 2023, 8, 39288–39302 CrossRef CAS PubMed.
- M.-H. Lee, Org. Electron., 2020, 76, 105465 CrossRef CAS.
- B. Kippelen and J.-L. Brédas, Energy Environ. Sci., 2009, 2, 251–261 RSC.
- M. Rezvani, M. D. Ganji, S. Jameh-Bozorghi and A. Niazi, Spectrochim. Acta, Part A, 2018, 194, 57–66 CrossRef CAS PubMed.
- P. W. Blom, V. D. Mihailetchi, L. J. A. Koster and D. E. Markov, Adv. Mater., 2007, 19, 1551–1566 CrossRef CAS.
- Z. Luo, T. Liu, Y. Wang, G. Zhang, R. Sun, Z. Chen, C. Zhong, J. Wu, Y. Chen, M. Zhang, Y. Zou, W. Ma, H. Yan, J. Min, Y. Li and C. Yang, Adv. Energy Mater., 2019, 9, 1900041 CrossRef.
- C. Yao, J. Zhao, Y. Zhu, B. Liu, C. Yan, D. F. Perepichka and H. Meng, ACS Appl. Mater. Interfaces, 2020, 12, 11543–11550 CrossRef CAS PubMed.
- Y. Cui, H. Yao, J. Zhang, K. Xian, T. Zhang, L. Hong, Y. Wang, Y. Xu, K. Ma, C. An, C. He, Z. Wei, F. Gao and J. Hou, Adv. Mater., 2020, 32, 1908205 CrossRef CAS PubMed.
- Y.-Q.-Q. Yi, H. Feng, M. Chang, H. Zhang, X. Wan, C. Li and Y. Chen, J. Mater. Chem. A, 2017, 5, 17204–17210 RSC.
- L. Bhattacharya, A. Brown, S. Sharma and S. Sahu, J. Phys. Chem. A, 2022, 126, 7110–7126 CrossRef CAS PubMed.
- Y. Lin, J. Wang, Z. G. Zhang, H. Bai, Y. Li, D. Zhu and X. Zhan, Adv. Mater., 2015, 27, 1170–1174 CrossRef CAS PubMed.
- M. J. Frisch, G. W. Trucks, H. B. Schlegel, G. E. Scuseria, M. A. Robb, J. R. Cheeseman, G. Scalmani, V. Barone, B. Mennucci, G. A. Petersson, H. Nakatsuji, M. Caricato, X. Li, H. P. Hratchian, A. F. Izmaylov, J. Bloino, G. Zheng, J. L. Sonnenberg, M. Hada, M. Ehara, K. Toyota, R. Fukuda, J. Hasegawa, M. Ishida, T. Nakajima, Y. Honda, O. Kitao, H. Nakai, T. Vreven, J. A. Montgomery Jr, J. E. Peralta, F. Ogliaro, M. Bearpark, J. J. Heyd, E. Brothers, K. N. Kudin, V. N. Staroverov, R. Kobayashi, J. Normand, K. Raghavachari, A. Rendell, J. C. Burant, S. S. Iyengar, J. Tomasi, M. Cossi, N. Rega, J. M. Millam, M. Klene, J. E. Knox, J. B. Cross, V. Bakken, C. Adamo, J. Jaramillo, R. Gomperts, R. E. Stratmann, O. Yazyev, A. J. Austin, R. Cammi, C. Pomelli, J. W. Ochterski, R. L. Martin, K. Morokuma, V. G. Zakrzewski, G. A. Voth, P. Salvador, J. J. Dannenberg, S. Dapprich, A. D. Daniels, O. Farkas, J. B. Foresman, J. V. Ortiz, J. Cioslowski and D. J Fox, Gaussian 09, Revision B.01, Gaussian Inc., Wallingford, 2010 Search PubMed.
- I. Shafiq, R. Zahid, K. Mahmood, M. A. Asgha, T. Ahamad, S. Ahmed and S. C. Ojha, J. Saudi Chem. Soc., 2023, 27, 101767 CrossRef CAS.
- B. Civalleri, C. M. Zicovich-Wilson, L. Valenzano and P. Ugliengo, CrystEngComm, 2008, 10, 405–410 RSC.
- C. Adamo and V. Barone, J. Chem. Phys., 1998, 108, 664–675 CrossRef CAS.
- V. S. Bryantsev, M. S. Diallo, A. C. Van Duin and W. A. Goddard III, J. Chem. Theory Comput., 2009, 5, 1016–1026 CrossRef CAS PubMed.
- M. Khalid, I. Shafiq, S. C. Ojha, A. A. Braga, T. Ahamad and M. Arshad, J. Photochem. Photobiol., A, 2023, 445, 115091 CrossRef CAS.
- K. J. Stevenson, J. Am. Chem. Soc., 2011, 133, 5621 CrossRef CAS.
- N. M. O'boyle, A. L. Tenderholt and K. M. Langner, J. Comput. Chem., 2008, 29, 839–845 CrossRef PubMed.
- T. Lu and F. Chen, J. Comput. Chem., 2012, 33, 580–592 CrossRef CAS PubMed.
- G. A. Zhurko and D. A. Zhurko, Chem Craft, version 1.6, 2009 Search PubMed.
- M. D. Hanwell, D. E. Curtis, D. C. Lonie, T. Vandermeersch, E. Zurek and G. R. Hutchison, J. Cheminf., 2012, 4, 1–17 Search PubMed.
- S. Ahmed, R. Dutta and D. J. Kalita, Chem. Phys. Lett., 2019, 730, 14–25 CrossRef CAS.
- R. Sikandar, A. Farhat, R. A. Khera, S. Jabeen, A. R. Ayub, P. Langer and J. Iqbal, J. Mol. Graphics Modell., 2021, 106, 107918 CrossRef CAS PubMed.
- I. Zubair, R. A. Kher, S. J. Akram, Y. A. El-Badry, M. U. Saeed and J. Iqbal, Chem. Phys. Lett., 2022, 793, 139459 CrossRef CAS.
- J.-i. Fujisawa, Chem. Phys. Lett., 2014, 608, 355–359 CrossRef CAS.
- J. Yang, Q.-S. Li and Z.-S. Li, Phys. Chem. Chem. Phys., 2021, 23, 12321–12328 RSC.
- S. A. Siddique, M. B. A. Siddique, R. Hussain, X. Liu, M. Y. Mehboob, Z. Irshad and M. Adnan, Comput. Theor. Chem., 2020, 1191, 113045 CrossRef CAS.
- M. B. A. Siddique, R. Hussain, S. A. Siddique, M. Y. Mehboob, Z. Irshad, J. Iqbal and M. Adnan, ChemistrySelect, 2020, 5, 7358–7369 CrossRef.
- M. N. Arshad, I. Shafiq, M. Khalid and A. M. Asiri, ACS Omega, 2022, 7, 11606–11617 CrossRef CAS PubMed.
- M. U. Khan, R. Hussain, M. Y. Mehboob, M. Khalid, Z. Shafiq, M. Aslam, A. A. Al-Saadi, S. Jamil and M. R. S. A. Janjua, ACS Omega, 2020, 5, 24125–24137 CrossRef CAS PubMed.
- S. Saravanan and V. Balachandran, Spectrochim. Acta, Part A, 2015, 138, 406–423 CrossRef CAS PubMed.
- M. Y. Mehboob, M. U. Khan, R. Hussain, K. Ayub, A. Sattar, M. K. Ahmad, Z. Irshad and M. Adnan, Spectrochim. Acta, Part A, 2021, 244, 118873 CrossRef CAS PubMed.
- R. G. Parr, R. A. Donnelly, M. Levy and W. E. Palke, J. Chem. Phys., 1978, 68, 3801–3807 CrossRef CAS.
- P. K. Chattaraj and D. R. Roy, Chem. Rev., 2007, 107, PR46–PR74 CrossRef CAS.
- R. G. Pearson, Proc. Natl. Acad. Sci. U. S. A., 1986, 83, 8440–8441 CrossRef CAS PubMed.
- J. Poater, I. Teixidor, J. Swart, M. Swart, M. Solà and I. Puig, J. Mex. Chem. Soc., 1983, 56, 311–315 Search PubMed.
- J. Padmanabhan, R. Parthasarathi, V. Subramanian and P. Chattaraj, J. Phys. Chem. A, 2007, 111, 1358–1361 CrossRef CAS PubMed.
- T. Koopmans, Physica, 1934, 1, 104–113 CrossRef.
- R. G. Parr, R. A. Donnelly, M. Levy and W. E. Palke, J. Chem. Phys., 1978, 68, 3801–3807 CrossRef CAS.
- M. Mohammadzaheri, S. Jamehbozorgi, M. D. Ganji, M. Rezvani and Z. Javanshir, Phys. Chem. Chem. Phys., 2023, 25, 21492–21508 RSC.
- M. N. Tahir, M. Khalid, A. Islam, S. M. A. Mashhadi and A. A. Braga, J. Mol. Struct., 2017, 1127, 766–776 CrossRef CAS.
- K. Q. Kayani, U. Yaqoob, S. Jabeen, S. Iqbal, M. Yaseen, M. Khalid, M. S. Akhter and J. Iqbal, Comput. Theor. Chem., 2021, 1202, 113305 CrossRef.
- Z. Zhao, C. Xu, Y. Ma, K. Yang, M. Liu, X. Zhu, Z. Zhou, L. Shen, G. Yuan and F. Zhang, Adv. Funct. Mater., 2022, 32, 2203606 CrossRef CAS.
- M. Adnan and J. K. Lee, RSC Adv., 2020, 10, 5454–5461 RSC.
- M. Adnan, Z. Irshad and J. K. Lee, RSC Adv., 2020, 10, 29010–29017 RSC.
- D. Liu, B. Kan, X. Ke, N. Zheng, Z. Xie, D. Lu and Y. Liu, Adv. Energy Mater., 2018, 8, 1801618 CrossRef.
- R. Saleem, A. Farhat, R. A. Khera, P. Langer and J. Iqbal, Comput. Theor. Chem., 2021, 1197, 113154 CrossRef CAS.
- J. Xiao, T. Yan, T. Lei, Y. Li, Y. Han, W. Song, S. Tan and Z. Ge, Org. Electron., 2020, 81, 105662 CrossRef.
- R. Hussain, M. Y. Mehboob, M. U. Khan, M. Khalid, Z. Irshad, R. Fatima, A. Anwar, S. Nawab and M. Adnan, J. Mater. Sci., 2021, 56, 5113–5131 CrossRef CAS.
- M. Adnan, M. Y. Mehboob, R. Hussain and Z. Irshad, Optik, 2021, 241, 166839 CrossRef CAS.
- M. Y. Mehboob, R. Hussain, Z. Irshad and M. Adnan, Bull. Korean Chem. Soc., 2021, 42, 597–610 CrossRef CAS.
- N. Kanwal, R. Hussain, A. Sattar, M. A. Assiri, M. Imran, A. Hussain, M. A. Yawer, R. Hussain, M. Y. Mehboob and M. Khalid, Opt. Quantum Electron., 2022, 54, 546 CrossRef CAS.
- U. Azeem, R. A. Khera, A. Naveed, M. Imran, M. A. Assiri, M. Khalid and J. Iqbal, ACS Omega, 2021, 6, 28923–28935 CrossRef CAS PubMed.
- M. M. A. Iqbal, M. Y. Mehboob, R. Hussain, M. Adnan and Z. Irshad, Comput. Theor. Chem., 2021, 1202, 113335 CrossRef.
- J.-P. Correa-Baena, L. Nienhaus, R. C. Kurchin, S. S. Shin, S. Wieghold, N. T. P. Hartono, M. Layurova, N. D. Klein, J. R. Poindexter and A. Polizzotti, Chem. Mater., 2018, 30, 3734–3742 CrossRef CAS.
- Z. Xiao, X. Jia, D. Li, S. Wang, X. Geng, F. Liu, J. Chen, S. Yang, T. P. Russell and L. Ding, Sci. Bull., 2017, 62, 1494–1496 CrossRef CAS PubMed.
- X. Ma, W. Gao, J. Yu, Q. An, M. Zhang, Z. Hu, J. Wang, W. Tang, C. Yang and F. Zhang, Energy Environ. Sci., 2018, 11, 2134–2141 RSC.
- M. T. Moghim, S. Jamehbozorgi, M. Rezvani and M. Ramezani, Spectrochim. Acta, Part A, 2022, 280, 121488 CrossRef PubMed.
- K. Wang, H. Wei, Z. Li and S. Lu, Dyes Pigm., 2021, 196, 109801 CrossRef CAS.
- U. Yaqoob, A. R. Ayub, S. Rafiq, M. Khalid, Y. A. El-Badry, Z. M. El-Bahy and J. Iqbal, J. Mol. Liq., 2021, 341, 117428 CrossRef CAS.
- N. Wu, Y. Wu, D. Walter, H. Shen, T. Duong, D. Grant, C. Barugkin, X. Fu, J. Peng and T. White, Energy Technol., 2017, 5, 1827–1835 CrossRef CAS.
- L. Zhang, W. Shen, R. He, X. Liu, X. Tang, Y. Yang and M. Li, Org. Electron., 2016, 32, 134–144 CrossRef CAS.
|
This journal is © The Royal Society of Chemistry 2024 |
Click here to see how this site uses Cookies. View our privacy policy here.