DOI:
10.1039/D4RA03730H
(Paper)
RSC Adv., 2024,
14, 22560-22568
Zn3V2O7(OH)2·2H2O/MXene cathode with fast ion diffusion for highly durable zinc ion batteries†
Received
21st May 2024
, Accepted 3rd July 2024
First published on 17th July 2024
Abstract
V-based materials are considered promising candidates as cathode materials for zinc ion batteries (ZIBs). However, the inherent low conductivity of V-based compounds leads to the sluggish diffusion kinetics of Zn2+ and serious cycling capacity degradation of ZIBs. Herein, 1D Zn3V2O7(OH)2·2H2O (ZVO) nanowires were grown on monodisperse 2D Ti3C2Tx MXene nanosheets via a facile microwave-assisted method. The introduction of Ti3C2Tx MXenes effectively improved the conductivity and hydrophilicity of ZVO. Furthermore, the Zn2+ diffusion coefficient of ZVO/Ti3C2Tx composites was enhanced to 10−7–10−8 cm2 s−1, which was superior to that of pure ZVO nanowires (10−8–10−10 cm2 s−1) and other previously reported typical V-based cathodes. The ZIBs based on the ZVO/Ti3C2Tx cathode possessed an excellent discharge specific capacity of 215.2 mAh g−1 at 0.1 A g−1 and cycling stability (84% retention over 14
000 cycles at 10 A g−1). Moreover, the flexible Zn//ZVO/Ti3C2Tx ZIBs using a gel electrolyte still exhibited good cycling stability and rate performance.
Introduction
Recently, cost-effective, environmentally friendly energy storage materials with high capacity and long life have attracted increasing attention.1,2 Li-ion batteries face intrinsic safety issues caused by hazardous and flammable organic electrolytes and have high production cost.3,4 Extensive research has been conducted on metal-ion batteries (Mg2+, Zn2+, Na+, etc.) with eco-friendly aqueous electrolytes, which are abundant in resources and inexpensive.5–8 Particularly, aqueous zinc ion batteries (ZIBs) have attracted more attention owing to the chemical and physical properties of the zinc anode, including low redox potential (−0.763 V vs. standard hydrogen electrode), high theoretical specific capacity (820 mAh g−1 and 5855 mAh cm−3), environmental friendliness and high safety characteristics.9,10 In view of the most significant component, cathodes, such as manganese-based compounds, V-based compounds, organic substances and Prussian blue analogs, have been extensively developed.11–13 V-based materials are promising candidates for ZIB cathode materials owing to their low cost, multi-valence states (V3+, V4+, V5+), and large interlayer/tunnel spacing.14–16
Typically, Zn3V2O7(OH)2·2H2O (ZVO) with a layered octahedron and columnar hexahedron structure has become a powerful competitor with improved electron migration and high Zn2+ storage capacity for ZIBs.17 ZVO nanowires were first synthesized using a microwave-assisted method and used as cathodes for ZIBs.18 Zn//ZVO batteries showed about a 68% retention ratio of original discharge capacity after 300 cycles at 0.2 A g−1. The diffusion coefficient of Zn2+ ions was approximately 10−9–10−10 cm2 s−1. ZVO nanowires with exposed (001) plane lattice exhibited a specific discharge capacity of 108 mAh g−1 (about 77% retention) after 700 cycles at 2 A g−1 and a diffusion coefficient of 10−11–10−10 cm2 s−1 for the Zn2+ ion.19 Few modification methods have been proposed to improve the diffusion kinetics of Zn2+ and cycling capacity of ZVO-based ZIBs.20,21 Conductive and flexible current collectors such as 1D carbon nanotubes and carbon cloth were used to improve the electrochemical reaction kinetics of ZVO, which facilitated Zn2+ diffusion and accelerated electron transport.22–24 An ultrathin HfO2 film was coated on ZVO as an artificial solid electrolyte interphase to decrease the by-product and suppress the dissolution of the ZVO cathode. The obtained HfO2-coated ZVO cathodes delivered 90% retention over 100 cycles at 0.1 A g−1 and 84% retention over 1000 cycles at 10 A g−1.25 In addition, the diffusion coefficient of Zn2+ was improved to 5 × 10−8–6.9 × 10−8 cm2 s−1 by constructing the bridge oxygen vacancies for refined 1D Zn2+ ion transport channels in ZVO.26 Gradient concentration of N was refilled into partial oxygen vacancies to further enhance the stability of oxygen vacancies and reduce the energy barrier for Zn2+ diffusion.27 Nonetheless, some issues, such as low inherent conductivity and poor structural stability, still need to be further improved.
Herein, ZVO/Ti3C2Tx composites were synthesized by growing 1D ZVO nanowires on the monodisperse 2D MXene by a facile microwave-assisted method. The MXene as the conductive and hydrophilic substrate additive not only provided abundant reactive sites but also enhanced the hydrophilicity and structural stability of ZVO/Ti3C2Tx composites. Moreover, the diffusion coefficient of Zn2+ and electrolyte-philicity of ZVO/Ti3C2Tx composites were significantly improved. The ZVO/Ti3C2Tx composites exhibited high discharge capacities of about 215.2 mAh g−1 at a current density of 0.1 A g−1. Impressively, the as-fabricated ZVO/Ti3C2Tx cathodes remained at about 84% and 88% capacity retention after 14
000 cycles at 10 A g−1 and 1200 cycles at 2 A g−1, respectively. Furthermore, the cycle stability and rate performance of ZVO/Ti3C2Tx cathode ZIBs constructed by gel electrolyte were demonstrated.
Experimental
Synthesis of Ti3C2Tx
Ti3C2Tx was achieved through selective etching of the Al layer from Ti3AlC2 feedstock. First, 3.2 g LiF was dissolved in 40 mL 9 mol L−1 HCl to obtain a homogeneous solution, followed by gradually adding Ti3AlC2. Next, the above mixture was stirred for 24 hours at 35 °C. Then, the obtained solution was washed with deionized water by centrifugation until the pH of the supernatant reached approximately 6, which was followed by sonication for 1 hour in an argon environment. Finally, the resulting solution was subsequently centrifuged at 3500 rpm for 20 minutes to obtain a specific concentration of Ti3C2Tx dispersion.
Synthesis of Zn3V2O7(OH)2·2H2O/Ti3C2Tx composites
Firstly, 1 mmol ammonium metavanadate (NH4VO3) was dissolved in 10 mL deionized water with stirring at 80 °C. Then, 1.5 mmol zinc nitrate hexahydrate (Zn(NO3)2·6H2O) dissolved in 10 mL deionized water and Ti3C2Tx were orderly poured into the NH4VO3 solution. Finally, the mixed solution was transferred into a microwave reaction tube and reacted at 160 °C for 6 h. The resulting ZVO/Ti3C2Tx composites were washed with deionized water, followed by freeze-drying for later application. In contrast, ZVO nanowires were synthesized without the addition of Ti3C2Tx.
The assembling of the zinc-ion batteries
The ZVO/Ti3C2Tx cathode was prepared by mixing the active material and conductive carbon black (Super P) and polyvinylidene fluoride (PVDF) binder in a ratio of 7
:
2
:
1. The mixture was vigorously stirred for 12 h, and then the resulting slurry was uniformly coated on a titanium foil substrate. The coated substrate was vacuum-dried at 80 °C for 12 h, followed by cutting into circular pieces. The average loading of the active material on the prepared cathode was 1.0–1.5 mg cm−2. The ZIB was built in a CR2032 coin cell with ZVO/Ti3C2Tx cathode, zinc foil anode, along with 21 mol L−1 lithium bis(trifluoromethanesulfonyl)imide (LiTFSI) and 1 mol L−1 zinc trifluoromethanesulfonate (Zn(CF3SO3)2) solution and a glass fiber filter membrane, which were respectively used as the electrolyte and the septum.
The assembling of the flexible zinc-ion batteries
First, 3.02 g LiTFSI and 0.18 g Zn(CF3SO3)2 were completely dissolved in 0.5 mL deionized water. Then, 160 mg acrylamide (AM), 3 mg ammonium persulfate (APS), and 0.15 mg N,N′-methylenebisacrylamide (MBA) were added to the above solution under stirring. Finally, the mixtures were injected into a mold, followed by heating at 70 °C for 1.5 h to obtain the polyacrylamide (PAM) hydrogel. The flexible zinc-ion batteries were assembled using the sandwich-type structure, where the ZVO/Ti3C2Tx electrodes and zinc electrodes were directly attached to the sides of the PAM hydrogel.
Characterization
Field emission scanning electron microscopy (SEM, HITACHI, S–4800) and transmission electron microscopy (TEM, JEOL JEM–2100) were performed to observe the morphology and microstructure of the samples. X-ray powder diffraction (XRD) and X-ray photoelectron spectroscopy (XPS) were respectively observed using Rigaku D/Max B diffractometer with Cu Kα radiation and Thermo Scientific Nexsa system. The Electrochemical characterizations were carried out using the LAND 2001A cell test system and electrochemical workstation (CHI 760E).
Results and discussion
Synthesis and characterization of Zn3V2O7(OH)2·2H2O/Ti3C2Tx composites
The ZVO/Ti3C2Tx composites were constructed by growing ZVO on the single-layer Ti3C2Tx nanosheets, as shown in Fig. 1a. Firstly, the single-layer dispersed Ti3C2Tx MXenes were obtained by etching the Al layer of Ti3AlC2 MAX using LiF and HCl, followed by an ultrasonic process. Before etching, Ti3AlC2 MAX appeared as a tightly packed and multilayered block (Fig. S1†). The XRD spectrum showed that the (002) characteristic peak of Ti3AlC2 MAX shifted from 9.6° to 6.7° after etching, indicating the successful preparation of Ti3C2Tx MXene with increased interlayer spacing (Fig. S2†). Subsequently, multiple layers of Ti3C2Tx were subjected to ultrasonic treatment, resulting in uniform single-layer MXene dispersion (Fig. S3†) with a thickness of 1.5 nm (Fig. S4†). The ZVO nanowires of ZVO/Ti3C2Tx composites were synthesized by a microwave-assisted method of Zn(NO3)2·6H2O and NH4VO3 in Ti3C2Tx (Fig. S5†). The capacity and cycling stability of ZVO/Ti3C2Tx composites were systematically adjusted by changing the chemical concentrations, reaction temperature, reaction time and MXene weight (Fig. S6–S9†). According to the XRD spectrum, the distinct characteristic diffraction peaks observed at 12.5°, 17.1°, 21.2°, 30.3°, 32.2°, 34.4° and 36.7° correspond to the (001), (010), (011), (012), (111), (020), and (021) crystal planes of ZVO (Fig. 1b). All peaks were consistent with the standard card PDF#50-0570 of hexagonal ZVO, indicating the successful synthesis of ZVO/Ti3C2Tx composites.
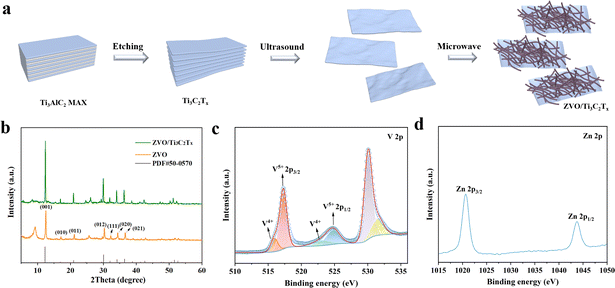 |
| Fig. 1 (a) Schematic synthesis of ZVO/Ti3C2Tx composites. (b) XRD patterns of ZVO and ZVO/Ti3C2Tx composites. The high-resolution XPS spectrum of (c) V 2p and (d) Zn 2p in the ZVO/Ti3C2Tx composites.† | |
XPS spectra were conducted for the chemical composition and valence state of ZVO/Ti3C2Tx MXene composites. Obviously, the elements C, Ti, V, O, and Zn were confirmed in the ZVO/Ti3C2Tx composites (Fig. S10†). As shown in the high-resolution XPS spectrum (Fig. 1c), the strong peaks at 517.3 eV and 524.9 eV were attributed to V5+ 2p3/2 and V5+ 2p1/2, respectively. Moreover, the characteristic peak of V4+ appeared as broad peaks at 515.9 eV and 523.18 eV. V in the hierarchical structure of ZVO/Ti3C2Tx MXene composite was dominant in the oxidization of V5+ and minimal in V4+. In the high-resolution Zn 2p spectrum (Fig. 1d), two distinct characteristic peaks at binding energies of 1020.7 eV and 1043.8 eV, mainly ascribed to the 2p3/2 and 2p1/2 of Zn in the ZVO/Ti3C2Tx composite, respectively. The characteristic absorption peak of the M−O bond appeared at 530.1 eV, mainly reflecting the bond between the metal atoms (Zn or V) and oxygen atoms, as shown in Fig. S10b.† Additionally, the absorption peak at binding energies of 531.4 eV was ascribed to the characteristic peak of the C–O bond.
The morphology and structure of ZVO and ZVO/Ti3C2Tx composites were investigated through SEM, EDX, and TEM analyses. As shown in Fig. S11,† the prepared ZVO exhibited interweaved and stacked nanowire morphology. After the introduction of Ti3C2Tx, ZVO nanowires were tightly wrapped around the two-dimensional Ti3C2Tx layer, as shown in Fig. 2a and S12†. Moreover, the self-aggregation of ZVO nanowires was prevented, attributing to the two-dimensional Ti3C2Tx, resulting in better electrochemical properties. Similar to the SEM images, the fabricated ZVO nanowires manufactured on the ZVO/Ti3C2Tx composite were elucidated with an interlayer spacing of 0.72 nm (Fig. 2b, c and S13†), which is consistent with the XRD results (Fig. 1b). Furthermore, V, O, Zn, and Ti elements were uniformly distributed on the ZVO/Ti3C2Tx composites, as shown in Fig. 2d.
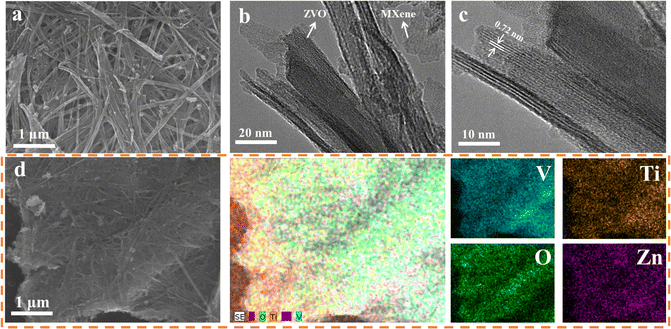 |
| Fig. 2 (a) SEM image and (b and c) TEM images and (d) element mapping of ZVO/Ti3C2Tx composites. | |
Electrochemical performance of zinc-ion batteries
To determine the electrochemical performance of the ZVO/Ti3C2Tx composite, Zn//ZVO/Ti3C2Tx ZIBs were fabricated by utilizing a 21 mol L−1 LiTFSI + 1 mol L−1 Zn(CF3SO3)2 electrolyte, zinc foil anode, and ZVO/Ti3C2Tx cathode (Fig. 3a). Galvanostatic charge/discharge (GCD) curves and rate capability of Zn//ZVO/Ti3C2Tx batteries were examined between 0 V and 1.8 V under various current densities, as shown in Fig. 3b and c. The Zn//ZVO/Ti3C2Tx battery exhibited a discharge capacity of 215.2 mAh g−1 at a current density of 0.1 A g−1 (Fig. 3b). Upon increasing current densities from 0.2, 0.5, and 1.0, to 2.0 A g−1, the discharge capacities were 185.8, 155.5, 129.1 and 95.2 mAh g−1, respectively, which were comparable to those of other reported vanadium-based ZIBs (Fig. S14†).18,25,28–35 In addition, when the current density returned to 0.1 A g−1, the discharge capacity of Zn//ZVO/Ti3C2Tx batteries reached 209.9 mAh g−1, retaining about 98% of the original discharge capacity (Fig. 3c). This indicated that the prepared Zn//ZVO/Ti3C2Tx batteries exhibited good rate performance and specific discharge capacities, better than that of pure ZVO (Fig. S15†). The results showed that the introduction of 2D Ti3C2Tx nanosheets with a high specific surface area can effectively increase the specific surface area of the ZVO/Ti3C2Tx composites, resulting in the increased number of active sites for the reaction between the Zn2+ and ZVO/Ti3C2Tx cathode (Fig. S16†). The reaction kinetics of Zn2+ and conductivity of ZVO/Ti3C2Tx cathode were respectively speeded up and improved due to the high conductivity of Ti3C2Tx (Fig. S17†), resulting in smaller charge transfer resistance (Rct, Fig. 3d). Furthermore, the introduction of Ti3C2Tx containing abundant hydrophilic groups has been found to effectively improve the hydrophilicity of the ZVO/Ti3C2Tx cathode with electrolyte (Fig. S18†). As a result, the internal ion migration resistance of the electrode was reduced, enabling a complete reaction between Zn2+ in the electrolyte and the electrode material. Furthermore, this increase in hydrophilicity accelerated the diffusion rate of ions with lower ion diffusion resistance (Zw, Fig. 3d). The addition of highly conductive MXene effectively decreased the charge transfer resistance (Rct) of the ZVO/Ti3C2Tx cathode, indicating the promotion of electronic conductivity than that of ZVO. As shown in Fig. 3d, the impedance data were analyzed and fitted to a typical equivalent electrical circuit consisting of the series resistance (Rs), interface resistance (Ri), constant-phase element (CPE), Rct and Warburg diffusion behavior (W). In addition, Zn//ZVO/Ti3C2Tx ZIBs exhibited a power density of 1220 W kg−1 and an energy density of 141.6 W h kg−1.
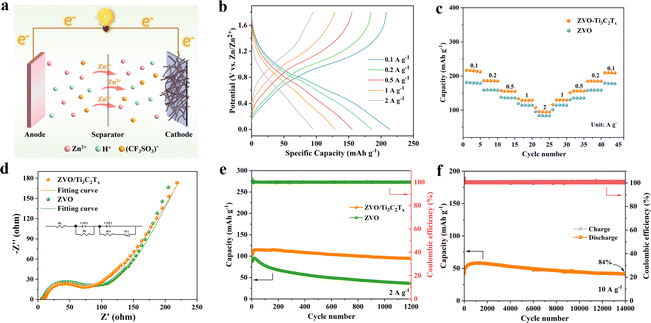 |
| Fig. 3 Electrochemical performances of Zn//ZVO/Ti3C2Tx batteries in 21 mol L−1 LiTFSI + 1 mol L−1 Zn(CF3SO3)2 aqueous electrolyte. (a) Schematic of the mechanism of the Zn//ZVO/Ti3C2Tx battery. (b) The galvanotactic charge/discharge curves and (c) rate capability of Zn//ZVO/Ti3C2Tx batteries under different current densities. (d) Electrochemical impedance spectroscopy (EIS) spectra of Zn//ZVO/Ti3C2Tx and Zn//ZVO batteries. Inset: the partial enlargement of the EIS spectra at the high-frequency region. Long cycling performance of Zn//ZVO/Ti3C2Tx batteries at (e) 2 A g−1 and (f) 10 A g−1. | |
To further investigate the effect of the introduction of 2D Ti3C2Tx, the cycling performance of the battery was tested at 2 A g−1. The discharge specific capacity of Zn//ZVO/Ti3C2Tx batteries could maintain 88% of initial capacity after 1200 charge/discharge cycles (Fig. 3e). The local damage of the ZVO/Ti3C2Tx structure led to a weakened intensity of the (001) characteristic peak (Fig. S19a†) and increased charge transfer resistance (Fig. S19b†) after cycling, resulting in slightly decreased discharge specific capacities. In contrast, the capacity of Zn//ZVO batteries decreased rapidly, retaining only 38% of the initial capacity. Especially, Zn//ZVO/Ti3C2Tx batteries could still retain 84% of initial capacity after 14
000 cycles at a large current density of 10.0 A g−1 (Fig. 3f), even maintaining 95% after 60 cycles at a low current density of 0.1 A g−1 (Fig. S20†). The cycling performance of the Zn//ZVO/Ti3C2Tx battery was superior to most other previously reported vanadium-based cathodes (Table S1†).18–23,25,36–39 That was because the introduction of Ti3C2Tx effectively inhibited the self-stacking of ZVO nanowires and mitigated the structural damage during Zn2+ intercalation/deintercalation. To explore the practical application value of ZIBs, two ZIBs connected in series can be used to power a clock (Fig. S21†). This demonstrated that the prepared ZIBs can be utilized as electrochemical storage devices for other electronic products.
Kinetics of zinc-ion batteries
Cyclic voltammogram (CV) measurements were performed further to confirm the kinetics performance of Zn//ZVO/Ti3C2Tx batteries. It can be observed that the CV curves almost overlapped, indicating the stable redox potential and highly reversible electrochemical transformation of the ZVO/Ti3C2Tx cathode (Fig. S22†). In addition, the area of the CV curve gradually increased with the increase in scanning rate from 0.2 mV s−1 to 1.0 mV s−1, as well as a lower cathodic and a higher anodic peak shift simultaneously (Fig. 4a). Two pairs of redox peaks were located at 0.49 V/0.76 V and 0.75 V/1.10 V at 0.2 mV s−1, consistent with the redox discharge and charge platforms in the previously tested GCD curve (Fig. 3b). The mentioned cathodic and anode peaks corresponded to the insertion/desertion of zinc ions, respectively.
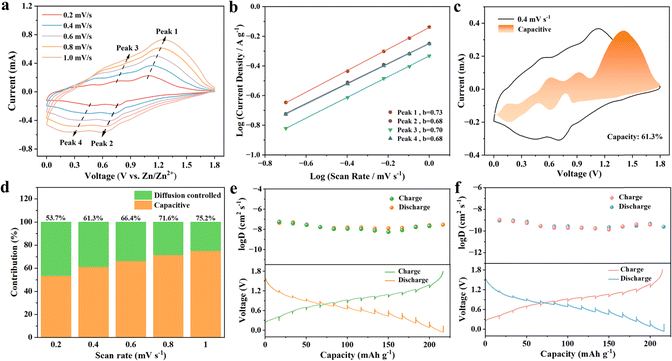 |
| Fig. 4 (a) Cyclic voltammogram profiles of Zn//ZVO/Ti3C2Tx batteries at different scan rates. (b) The fitting plots between log (i) and log (v) at different peak currents. (c) Quantification of the capacitive (orange area) and diffusion charge storage in the ZVO/Ti3C2Tx electrode at a scan rate of 0.4 mV s−1 and (d) the ratio of the capacitive contribution (orange) to the diffusion contribution (green) as a function of scan rate. GITT curves and corresponding Zn2+ diffusion coefficients for (e) Zn//ZVO/Ti3C2Tx batteries and (f) Zn//ZVO batteries at 0.5 A g−1. | |
To better understand the afore-mentioned superior performance, the power-law relationship between the peak current (i) and scan rate (v) can be determined by the following equation:
a and
b were variable parameters in the formula. In general, the coefficient
b varied in the range of 0.5 to 1.0, reflecting the rate-limiting step and different charge storage states. The discharge/charge process was completely dominated by the ionic diffusion-controlled process (
b = 0.5) while attributed to the capacitive-limited behavior through a fast surface reaction (
b = 1.0). According to the fitting curve in
Fig. 4b, the
b values fitted to the four peaks of Zn//ZVO/Ti
3C
2T
x batteries were respectively calculated as 0.73, 0.68, 0.70 and 0.68, indicating the co-dominated discharge/charge process by capacitive behavior and ionic diffusion-controlled process. The following deformed equation was further used to accurately determine the contribution ratios of capacitive-limited behavior and diffusion-controlled process:
where
k1 and
k2 were varied parameters with the peak current (
i). Generally,
k1ν and
k2ν1/2 represented the capacitive-limited and ionic diffusion-controlled contribution of the total capacity, respectively. At the scanning speed of 0.4 mV s
−1, the capacitive contribution rate was about 61.3% of the overall capacity (
Fig. 4c).
Fig. 4d demonstrates the ratio of capacitive-limited contribution (orange) and ionic diffusion-controlled contribution (green) as a function of scan rate. The capacitive-limited contribution gradually increased from 53.7% to 75.2% with the increase in the scanning rate from 0.2 to 1.0 mV s
−1. The capacitance contribution rates of Zn//ZVO/Ti
3C
2T
x batteries were larger than those of Zn//ZVO batteries at the same scanning rate (Fig. S23
†). This was because of the increasing active sites of the Zn
2+ reaction with a larger specific surface area and more electrolyte-philic cathode due to the introduction of Ti
3C
2T
x and a small capacitance of Ti
3C
2T
x.
The galvanostatic intermittent titration technique (GITT) was further demonstrated to investigate the Zn2+ kinetics diffusion behavior in the lattices of ZVO/Ti3C2Tx composite cathodes. The diffusion coefficient of Zn2+ (DZn2+) was determined using the following formula:
τ, M, m, Vm, and A represent the relaxation time, molar mass of active materials, mass of active materials, molar volume, and effective contact area between the electrode and electrolyte, respectively. Especially, ΔEs and ΔEτ were the voltage change of pulse and voltage change of constant-current charge/discharge, respectively. GITT curves and the corresponding DZn2+ of Zn//ZVO/Ti3C2Tx batteries and Zn//ZVO batteries at 0.5 A g−1 are shown in Fig. 4e and f. The Zn2+ diffusion coefficient of ZVO/Ti3C2Tx composites as the cathode was calculated in the range of 10−7 to 10−8 cm2 s−1, which was superior to pure ZVO nanowires (10−8–10−10 cm2 s−1) and other previously reported typical vanadium-based cathodes.20,36,40–44 The introduction of Ti3C2Tx with high electrical conductivity and good hydrophilic properties inhibited the self-stacking of ZVO nanowires and increased available active areas, resulting in fast solid-state diffusion kinetics of Zn2+. This also accounted for the superior cycling performances and rate properties of the Zn//ZVO/Ti3C2Tx battery.
Mechanism of zinc-ion batteries
In order to further study the zinc storage mechanism of ZVO/Ti3C2Tx electrodes, ex situ XRD and XPS characterization were conducted. Various charge/discharge states (points A–G) were subjected to ex situ XRD analysis at 0.5 A g−1, as shown in Fig. 5a and b. When discharging from 1.8 V to 0 V, the 2θ angle shifted from 12.1° at point A to 11.8° at point D. This shift indicated that Zn2+ ions were inserted into the ZVO/Ti3C2Tx layers during discharge, causing an expansion of the interlayer spacing. Upon charging back to 1.8 V, the (001) peak position of ZVO/Ti3C2Tx almost returned to its starting position at point A, ascribing to the desertion of Zn2+ from ZVO/Ti3C2Tx. The well-designed ZVO/Ti3C2Tx represented high reversibility of Zn2+ intercalation/deintercalation.
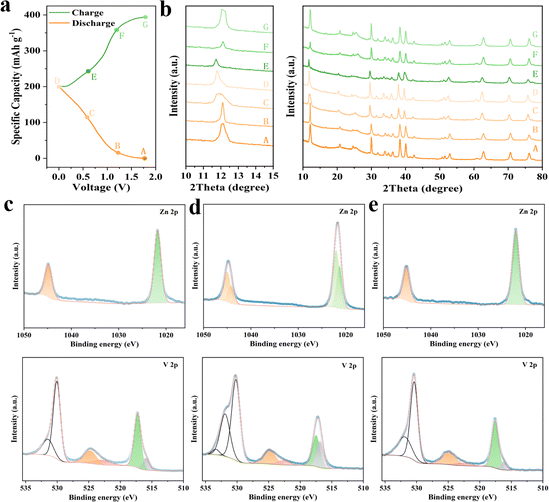 |
| Fig. 5 Zn-storage mechanism of ZVO/Ti3C2Tx composites. (a) Charge/discharge profiles of the Zn//ZVO/Ti3C2Tx battery at a current density of 0.5 A g−1. (b) Ex situ XRD patterns collected at different charge/discharge depths. Ex situ high-resolution XPS spectroscopy of Zn 2p and V 2p at (b) point A, (c) point D and (e) point G in (a). | |
The reversible insertion/desertion mechanism of Zn2+ was further verified using ex situ XPS, as shown in Fig. 5c–e. In the fully charged states A (Fig. 5c), two remarkable diffraction peaks that corresponded to Zn 2p1/2 and Zn 2p3/2 were observed at 1022.1 eV and 1045.1 eV, which were detected in the Zn 2p fine XPS spectra of the original ZVO/Ti3C2Tx composites (Fig. 1d). When discharged to 0 V (at point D, Fig. 5d), new peaks from the Zn 2p3/2 and Zn 2p1/2 orbitals appeared at 1021.3 eV and 1044.1 eV, respectively, indicating the successful insertion of Zn2+ from the electrolyte into the ZVO/Ti3C2Tx electrode. Upon charging back to G (1.8 V, Fig. 5e), new peaks from Zn 2p3/2 and Zn 2p1/2 disappeared, while XPS spectroscopy of Zn 2p returned to its starting position at point A. The effect of Zn2+ on the valence changes of V elements can be evidenced in the ex situ XPS patterns (Fig. 5c–e). When discharging to 0 V, the intensity of V5+ weakened and another V 2p3/2 feature appeared, indicating a partial reduction of V5+ to Vδ+ (4 < δ < 5). At the fully charged state of A or G, the Vδ+ contribution almost diminished. These results confirmed the zinc storage mechanism of the ZVO/Ti3C2Tx electrode consistent with the ex situ XRD results (Fig. 5b), indicating good reversibility and stability of the ZVO/Ti3C2Tx electrode.
It can be calculated that 1.93 Zn2+ can be inserted into per molecule of ZVO at the current density of 0.1 A g−1, corresponding to an ≈3.86 electron redox process. Consequently, the electrochemical reaction of the Zn//ZVO/Ti3C2Tx battery can be illustrated and formulated as follows:
Cathode: 1.93Zn2+ + 3.86e− + Zn3V2O7(OH)2·2H2O ↔ Zn4.93V2O7(OH)2·2H2O |
During the discharge process, 1.93 Zn2+ ions were inserted into ZVO to establish the phase of Zn4.93V2O7(OH)2·2H2O at the cathode. Meanwhile, the Zn anode was reduced to Zn2+, losing the electron. Upon reverse charging, Zn2+ ions were gradually deserted from Zn4.93V2O7(OH)2·2H2O to re-construct the ZVO phase, while the Zn2+ regained the electron to deposit on the anode.
Electrochemical performance of flexible zinc-ion batteries
Engineering flexible batteries is significant for wearable electronics. To exemplify the practicability of ZVO/Ti3C2Tx composites, flexible ZIBs were constructed with PAM hydrogel electrolyte containing Zn2+ and Li+ ions, a cathode of ZVO nanowires in situ grown on 2D Ti3C2Tx and Zn anode, as displayed in Fig. S24.† Typical absorption bands at 1660 and 3196 cm−1 ascribed to C
O vibration and N–H stretching vibration, respectively, confirming the successful preparation of PAM hydrogel (Fig. S25†). Especially, PAM hydrogel possessed a 3D porous profile (Fig. S26†), which was conducive to Zn2+ transportation and beneficial for higher impoundment capacity. The resultant PAM hydrogel provided well-stretchable properties (a tensile strain of 1445.5% and tensile strength of 255 kPa, Fig. S27 and 28†), showing considerable deformability to the gel electrolyte of flexible ZIBs.
The CV and GCD measurements of the flexible battery at different scanning rates explored the electrochemical performance of the flexible ZIBs, as shown in Fig. 6a and b. Furthermore, the locations of the redox peak were consistent with the voltage range of the button battery (Fig. 4a). The flexible ZIBs delivered excellent rate capacities of 201.8 mAh g−1 at 0.1 A g−1 with 63.5 mAh g−1 at 2.0 A g−1. Returning to 0.1 A g−1, the discharge capacities of ZIBs retained about 88% of the initial capacity at 0.1 A g−1 (Fig. 6c). After 1400 cycles at 2.0 A g−1, ZIBs maintained about 80% of their initial capacity with a coulomb efficiency of about 100%, indicating outstanding cycling stability (Fig. 6d). This decrease in capacity was because of the increased Rct caused by the gradually generated by-products during the discharge/charge cycles, as presented in Fig. S29.† In addition, the charge/discharge curves of flexible Zn//ZVO/Ti3C2Tx ZIBs under different bending times are shown in Fig. 6e. They exhibited about 95% capacity retention after 200 bending cycles. Moreover, after 1000 cycles of bending, ZIB-based ZVO/Ti3C2Tx composites retained approximately 86% of their initial capacity (Fig. S30†), indicating good flexibility and showing the prospective possibility to be energy storage devices for wearable devices. Furthermore, two flexible ZIBs in series can successfully light the clock (Fig. 6f, Video S1†), proving great potential for practical applications.
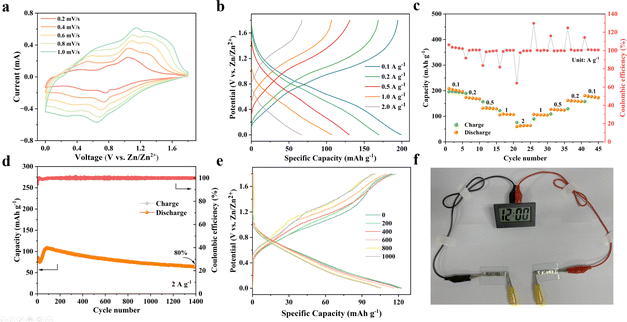 |
| Fig. 6 (a) Cyclic voltammogram profiles of flexible Zn//ZVO/Ti3C2Tx ZIBs at different scan rates. (b) Galvanostatic charge/discharge profiles of flexible ZIBs at a current density from 0.1 to 2.0 A g−1. (c) Rate capability of flexible Zn//ZVO/Ti3C2Tx ZIBs under different current densities. (d) Long cycling performance of flexible Zn//ZVO/Ti3C2Tx ZIBs batteries at 2 A g−1. (e) Galvanostatic charge/discharge curves of flexible Zn//ZVO/Ti3C2Tx ZIBs during bending cycles. (f) Digital photographs of flexible Zn//ZVO/Ti3C2Tx ZIBs in series to power a clock. | |
Conclusions
In summary, MoS2/Ti3C2Tx composites were constructed through the 1D ZVO nanowires on 2D Ti3C2Tx MXene by a microwave-assisted method. MXene prevented the coated ZVO from agglomerating during the intercalation/deintercalation process of Zn2+, resulting in the enhanced structural stability of ZVO/Ti3C2Tx composites. Furthermore, the diffusion coefficient of Zn2+ and electrolyte-philicity of ZVO/Ti3C2Tx composites were significantly improved. The ZVO/Ti3C2Tx electrode exhibited ultrahigh specific discharge performance (215.2 mAh g−1 specific capacity at 0.1 A g−1) and long cycling stability (84% capacity retention over 14
000 cycles at 10 A g−1, 88% capacity retention over 1200 cycles at 2 A g−1). Furthermore, the flexible Zn//ZVO/Ti3C2Tx ZIBs using PAM gel electrolyte still delivered excellent capacities of 201.8 mAh g−1 at 0.1 A g−1 and kept 80% retention of initial capacity after 1400 cycles at 2.0 A g−1. This study is expected to inspire the design of more rational cathode systems to obtain ZIBs with high capacities.
Data availability
The data supporting this article have been included as part of ESI.†
Author contributions
W. Su contributed to most experimental work. M. Lang completed the required experiments in the revised manuscript. W. Li assisted in the characterization of some materials. H. Li conceived this idea and wrote the manuscript. All the authors assisted in experiments and provided constructive comments on the text.
Conflicts of interest
There are no conflicts to declare.
Acknowledgements
We are grateful for the financial support from the National Natural Science Foundation of China (52203269 and 22076122) and Shanghai Government (22ZR1480200).
References
- B. Dunn, H. Kamath and J.-M. Tarascon, Science, 2011, 334, 928–935 CrossRef CAS.
- M. Armand and J. M. Tarascon, Nature, 2008, 451, 652–657 CrossRef CAS.
- M. Song, H. Tan, D. Chao and H. J. Fan, Adv. Funct. Mater., 2018, 28, 1802564 CrossRef.
- J. B. Goodenough and K.-S. Park, J. Am. Chem. Soc., 2013, 135, 1167–1176 CrossRef CAS.
- Y. Li, Z. Wang, Y. Cai, M. E. Pam, Y. Yang, D. Zhang, Y. Wang and S. Huang, Energy Environ. Mater., 2022, 5, 823–851 CrossRef CAS.
- J. Ming, J. Guo, C. Xia, W. Wang and H. N. Alshareef, Mater. Sci. Eng. R Rep., 2019, 135, 58–84 CrossRef.
- S. W. D. Gourley, R. Brown, B. D. Adams and D. Higgins, Joule, 2023, 7, 1415–1436 CrossRef CAS.
- X. Fan, C. Zhong, J. Liu, J. Ding, Y. Deng, X. Han, L. Zhang, W. Hu, D. P. Wilkinson and J. Zhang, Chem. Rev., 2022, 122, 17155–17239 CrossRef CAS.
- G. Li, L. Sun, S. Zhang, C. Zhang, H. Jin, K. Davey, G. Liang, S. Liu, J. Mao and Z. Guo, Adv. Funct. Mater., 2024, 34, 2301291 CrossRef CAS.
- Y. Bai, Y. Qin, J. Hao, H. Zhang and C. M. Li, Adv. Funct. Mater., 2024, 34, 2310393 CrossRef CAS.
- H. Li, Y. Liu, Z. Chen, Y. Yang, T. Lv and T. Chen, J. Colloid Interface Sci., 2023, 639, 408–415 CrossRef CAS PubMed.
- X. Wang, Z. Zhang, B. Xi, W. Chen, Y. Jia, J. Feng and S. Xiong, ACS Nano, 2021, 15, 9244–9272 CrossRef CAS PubMed.
- Y. Zeng, D. Luan and X. W. Lou, Chem, 2023, 9, 1118–1146 CAS.
- C. Guo, S. Yi, R. Si, B. Xi, X. An, J. Liu, J. Li and S. Xiong, Adv. Energy Mater., 2022, 12, 2202039 CrossRef CAS.
- Z. Xing, G. Xu, J. Han, G. Chen, B. Lu, S. Liang and J. Zhou, Trends Chem., 2023, 5, 380–392 CrossRef CAS.
- T. Lv, Y. Peng, G. Zhang, S. Jiang, Z. Yang, S. Yang and H. Pang, Adv. Sci., 2023, 10, 2206907 CrossRef CAS.
- L. Li, T. Jia, X. Pei, S. Hou, B. Liu, Q. Yang and Z. Zhu, Ionics, 2022, 28, 283–293 CrossRef CAS.
- C. Xia, J. Guo, Y. Lei, H. Liang, C. Zhao and H. N. Alshareef, Adv. Mater., 2018, 30, 1705580 CrossRef.
- H. Cao, C. Peng, Z. Zheng, Z. Lan, Q. Pan, U. G. Nielsen, P. Norby, X. Xiao and S. Mossin, Electrochim. Acta, 2021, 388, 138646 CrossRef CAS.
- X. Li, X. Zhu, Z. Cao, Z. Xu, J. Shen and M. Ye, Small, 2022, 18, 2105325 CrossRef CAS.
- L. Ren, G. Yu, H. Xu, W. Wang, Y. Jiang, M. Ji and S. Li, ACS Sustain. Chem. Eng., 2021, 9, 12223–12232 CrossRef CAS.
- Z. Pan, J. Yang, J. Yang, Q. Zhang, H. Zhang, X. Li, Z. Kou, Y. Zhang, H. Chen, C. Yan and J. Wang, ACS Nano, 2020, 14, 842–853 CrossRef CAS PubMed.
- Y. Cui, Y. Ding, L. Guo, C. Guo, Y. Liu, Y. Bai, G. Li and K. Wang, Energy Mater., 2023, 3, 300023 CAS.
- Y. Zhu, X. Lin, X. Jin, T. Han, H. Zhang and J. Liu, Chem. Commun., 2022, 58, 8117–8120 RSC.
- J. Guo, J. Ming, Y. Lei, W. Zhang, C. Xia, Y. Cui and H. N. Alshareef, ACS Energy Lett., 2019, 4, 2776–2781 CrossRef CAS.
- M. Wang, G. Zhao, X. Yu, X. Bai, A. Chen, C. Zhao, P. Lyu and N. Zhang, Nano Energy, 2023, 110, 108336 CrossRef CAS.
- M. Wang, G. Zhao, X. Bai, W. Yu, C. Zhao, Z. Gao, P. Lyu, Z. Chen and N. Zhang, Adv. Energy Mater., 2023, 13, 2301730 CrossRef CAS.
- P. He, M. Yan, G. Zhang, R. Sun, L. Chen, Q. An and L. Mai, Adv. Energy Mater., 2017, 7, 1601920 CrossRef.
- T. Wei, Q. Li, G. Yang and C. Wang, Electrochim. Acta, 2018, 287, 60–67 CrossRef CAS.
- Z. Wu, Y. Wang, L. Zhang, L. Jiang, W. Tian, C. Cai, J. Price, Q. Gu and L. Hu, ACS Appl. Energy Mater., 2020, 3, 3919–3927 CrossRef CAS.
- L. Chen, Z. Yang, J. Wu, H. Chen and J. Meng, Electrochim. Acta, 2020, 330, 135347 CrossRef CAS.
- B. Lan, Z. Peng, L. Chen, C. Tang, S. Dong, C. Chen, M. Zhou, C. Chen, Q. An and P. Luo, J. Alloys Compd., 2019, 787, 9–16 CrossRef CAS.
- L. Deng, H. Chen, J. Wu, Z. Yang, Y. Rong and Z. Fu, Ionics, 2021, 27, 3393–3402 CrossRef CAS.
- X. Pu, T. Song, L. Tang, Y. Tao, T. Cao, Q. Xu, H. Liu, Y. Wang and Y. Xia, J. Power Sources, 2019, 437, 226917 CrossRef CAS.
- Z. Wu, C. Lu, Y. Wang, L. Zhang, L. Jiang, W. Tian, C. Cai, Q. Gu, Z. Sun and L. Hu, Small, 2020, 16, 2000698 CrossRef CAS PubMed.
- H. Liu, J.-G. Wang, H. Sun, Y. Li, J. Yang, C. Wei and F. Kang, J. Colloid Interface Sci., 2020, 560, 659–666 CrossRef CAS PubMed.
- Y. Ma, Y. Qi, Y. Niu, Y. Liu, S. Bao and M. Xu, Inorg. Chem. Front., 2022, 9, 2706–2713 RSC.
- L. Rao, Z. Zhou, H. Liu, W. Peng, Y. Li, F. Zhang and X. Fan, J. Colloid Interface Sci., 2023, 629, 473–481 CrossRef CAS.
- Y. Li, H. Ba, Z. Wang, S. Wu, Y. Shang, S. Huang and H. Y. Yang, Mater. Today Energy, 2024, 39, 101460 CrossRef CAS.
- M. Liao, J. W. Wang, L. Ye, H. Sun, Y. Z. Wen, C. Wang, X. M. Sun, B. J. Wang and H. S. Peng, Angew. Chem. Int. Ed., 2020, 59, 2273–2278 CrossRef CAS PubMed.
- J. Ding, Z. Du, B. Li, L. Wang, S. Wang, Y. Gong and S. Yang, Adv. Mater., 2019, 31, 1904369 CrossRef CAS PubMed.
- N. Liu, X. Wu, L. Fan, S. Gong, Z. Guo, A. Chen, C. Zhao, Y. Mao, N. Zhang and K. Sun, Adv. Mater., 2020, 32, 1908420 CrossRef CAS.
- S. Liu, H. Zhu, B. Zhang, G. Li, H. Zhu, Y. Ren, H. Geng, Y. Yang, Q. Liu and C. C. Li, Adv. Mater., 2020, 32, 2001113 CrossRef CAS PubMed.
- W. Liang, D. Rao, T. Chen, R. Tang, J. Li and H. Jin, Angew. Chem. Int. Ed., 2022, 61, e202207779 CrossRef CAS PubMed.
|
This journal is © The Royal Society of Chemistry 2024 |
Click here to see how this site uses Cookies. View our privacy policy here.