DOI:
10.1039/D4RA03897E
(Paper)
RSC Adv., 2024,
14, 22642-22655
Optimizing white light emission in Dy(III) complexes: impact of energy transfer from mono and bidentate ligands on luminescence†
Received
27th May 2024
, Accepted 11th July 2024
First published on 18th July 2024
Abstract
Complexes of dysprosium(III) ions with 1,1,1,5,5,5-hexafluoro-2,4-pentanedione featuring various mono and bi-dentate neutral ligands have been prepared and thoroughly investigated. The synthesized complexes exhibit an octa-coordinated environment, achieved by stoichiometrically combining organic ligands and Dy(III) ions. This octa-coordination environment of Dy(III) ion was confirmed by FT-IR spectroscopy, thermogravimetry and elemental analysis. Near-white light (NWL) is emitted when complexes were exposed to UV radiation, indicating a significant flow of energy from the sensitizing moieties towards the Dy(III) ion. This NWL emission might have resulted due to a balance between the intensities corresponding to emission peaks at 480 nm (blue) and 575 nm (yellow) in Dy1–Dy3. Emission spectra recorded at different excitation wavelength were utilized to study the tunability of CIE color coordinates. In addition to their high thermal stability, the complexes display bipolar paramagnetic shifts in their NMR spectra. The 4F9/2 → 6H13/2 transition, contributing ∼62% of the total emission, stands out as a promising candidate for laser amplification due to its dominance in the emission spectra. Additionally, NWL emission observed in a solid Dy(III) complex opens intriguing possibilities for its application in next-generation white-light emitting devices.
1 Introduction
There has been a strong push towards the development and wide spread use of energy efficient SSL (solid state lighting) technologies and smart devices in response to increased global demand for energy. Solid-state white-light-emitting materials offer remarkable benefits, like energy conservation and extended lifespan, leading to their widespread adoption across various applications, ranging from large-panel displays to lasers.1–4 It is important to note that SSL sources can be classified based on the material used in their fabrication. For instance, LEDs employ inorganic phosphors, while OLEDs make use of organic semiconductors. Furthermore, SSLs can be categorized based on the method by which they emit light, with LEDs utilizing UV excitation and OLEDs relying on electrical excitation.5,6 Considerable interest surrounds the realm of white light emission (WLE) which can be obtained using two different approaches: dual color emitters [blue either with red (B/R) or with yellow (B/Y)] or simply employing three-colour emitters that mix primary colors (RGB).7–9 To generate white light, a variety of methods are employed, including the incorporation of different dopants or multiple phase matrices. These matrices can be composed of various materials like nanomaterials, organic compounds, hybrid organic–inorganic substances, metal complexes or inorganic phosphors. These materials have unique properties for achieving white light emission and sometimes combining these different materials is necessary to reach the desired outcome.
Additionally, WLE from single molecules is also attainable, covering entire visible spectrum.10–12 Such systems comprises of a single-phase-single-emitter which eases the manufacturing process, resulting in materials which are light weight and thin, making them particularly well-suited for use in optical devices.13 WLE from single molecules can be generated by utilizing trivalent lanthanide (Ln) complexes. The fascinating aspect of lanthanide luminescence is that it relies on indirect excitation of lanthanide ions, unlike other conventional photo-excitation methods. This is on account of parity restricted behavior of their f–f transitions which results in low absorptivity (0.5–3 dm3 mol1 cm1).14,15 To overcome this limitation, researchers have turned to suitable sensitizers, which possess the unique ability to indirectly transfer energy to lanthanide ions and function as antennas.16,17 They absorb incident light and sensitize metal ion by efficiently transferring this energy to their excited states. Subsequently, excited Ln(III) ion engages in radiative relaxations to the lower electronic states. Narrow peaks are obtained in the emission spectra of these complexes due to the well-shielded 4f electrons of the lanthanide ions, which result in sharp and well-defined electronic transitions that are relatively unaffected by the ligand field and surrounding environment.
However, it is essential to highlight that selection of chromophoric sensitizer should be done carefully so that it functions as antenna along with producing its own emission in blue region which is basic necessity to produce white light.18–20 This dual functionality is crucial for the production of white light when it is coordinated with the yellow light-emitting Dy3+ ion. Frequently employed antenna ligands include β-diketones and aromatic carboxylic acids, among others.21–23 Along with these ligands, various neutral ligands like triphenylphosphine oxide, indazole, bipyridine, pyrazine etc. are also utilized in ternary complexes. These ligands apart from sensitizing the central metal ion, forms a protective coordination sphere to keep the high vibration solvent molecules away from the complex.24,25 In this article, one [Dy(hfpd)3(M2)] and three [Dy(hfpd)3(L)] (hfpd: 1,1,1,5,5,5-hexafluoro-pentane-2,4-dione), M: tri-n-octylphosphineoxide (TOPO), L: 1,10-phenanthroline (phen), neocuproine (neoc) and bathocuproine (bathoc) type of complexes were synthesized. The ligands used in the synthesis have high probability to coordinate with Ln3+ ions due to the strong affinity of oxygen and nitrogen atoms towards lanthanide ions and their π–π* transitions result in strong UV absorption and efficiently sensitize Ln3+ ions. The [Dy(hfaa)3phen] complex26 has been previously documented in the literature. The potential applications of these complexes span a broad spectrum, covering diverse fields such as solid-state lighting, chemical and biological sensing, temperature monitoring and telecommunication.27–29
2 Experimental section
2.1 Materials
DyCl3·6H2O, hfpd, TOPO, phen, neoc and bathoc of high purity were sourced from Sigma Aldrich. AR or spectroscopic grade hexane and ethanol along with reagent 25% ammonia solution were sourced from Sigma-Aldrich. All the procured chemicals were employed as received and the reactions were performed under ambient conditions.
2.2 Method
Quantification of carbon, hydrogen and nitrogen contents was achieved by employing Series II PerkinElmer Elemental Analyser (2400). While, FT-IR spectra (4000–400 cm−1) were collected employing a Nicolet iS-50 FTIR spectrometer. The KBr pellets were formed by incorporating a small quantity of the complex into KBr and then thoroughly grounding it. 1H NMR spectra were recorded using a FT-NMR spectrometer in CDCl3. Shimadzu 2450 UV-visible spectrophotometer was employed to observe electronic spectra ranging 200–800 nm. Photoluminescent (PL) properties were analyzed through Horiba Jobin YVON Fluorolog Model FL-3-11 spectrofluorometer equipped with xenon lamp as light source and 0.5 mm excitation and emission slit width. A Fluorescence Spectrophotometer was utilized to investigate the lifetimes of the prepared samples. The current–voltage relationship was characterized at a scan rate of 0.1 V s−1 using the PARSTAT 4000 instrument. The complexes were subjected to thermal analyses under a N2 at temperature rise of 10 °C per minute, utilizing Hitachi STA thermal analyzer 7300 and the findings are presented in the form of a thermogram.
2.3 Synthesis of ternary Dy3+ complexes
Synthesis of dysprosium(III) complexes was carried out using an in situ approach (Fig. 1).30,31 25% NH4OH solution was added to 5 mL ethanolic solution of 6.48 mmol hfpd. The contents were kept in a 50 mL container and sealed until the complete dissolution of ammonia vapours which led to the formation of ammonium salt of hexafluoroacetylacetone, [NH4(hfpd)]. Subsequently, this mixture was combined with 5 mL solution of 2.16 mmol DyCl3·6H2O solution in ethanol and further ethanolic solution of neutral ligand was added. The stoichiometric relationship between DyCl3·6H2O, hfpd and neutral ligands was 1
:
3
:
1 in Dy2–Dy4 complex and 1
:
3
:
2 in Dy1. This mixture was kept within a pH range of 6–7 and underwent stirring for 12 h at room temperature. Completion of synthetic reaction was marked by formation of precipitates of Dy1, Dy2, Dy3 and Dy4 with TOPO, phen, neoc and bathoc, respectively. The mixtures were left for slow evaporation at room temperature and the formed precipitates underwent repeated washing with both ethanol and hexane.
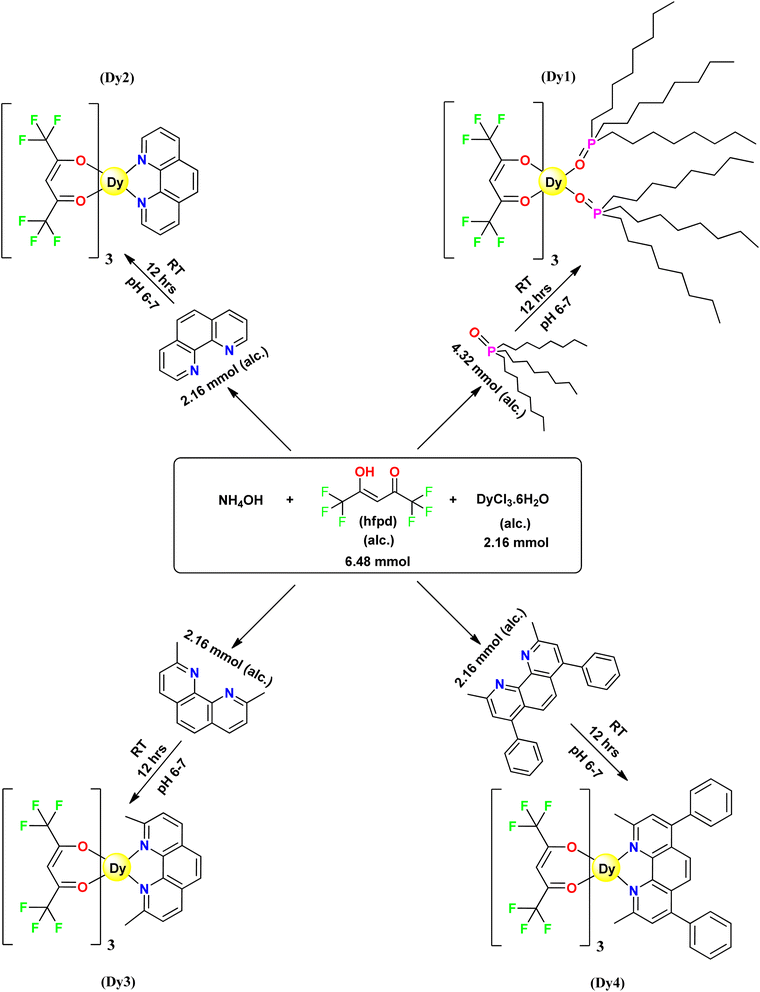 |
| Fig. 1 Schematic pathway for preparation of Dy1–Dy4. | |
3 Findings and interpretation
3.1 Preliminary assessment
CHN analysis was conducted for precise establishment of elemental composition of synthesized trivalent dysprosium compounds. The elemental analysis findings showed close approximation with calculated elemental content, indicating that the complexes were prepared in the correct stoichiometry. Moreover, all the compounds displayed solubility in dichloromethane, chloroform and dimethyl sulphoxide under standard laboratory conditions. Table 1 presents the color and elemental composition for Dy1 to Dy4.
Table 1 Primary findings of C, H and N component in % of Dy1–Dy4
Complex |
Colour shown |
C calcd. (Expt.) |
H calcd. (Expt.) |
N calcd. (Expt.) |
Dy1 |
White |
48.60 (48.65) |
6.80 (6.78) |
— |
Dy2 |
White |
33.65 (33.61) |
1.15 (1.18) |
2.91 (2.93) |
Dy3 |
Cream |
35.12 (35.17) |
1.52 (1.50) |
2.82 (2.85) |
Dy4 |
Light yellow |
43.04 (43.09) |
2.03 (2.01) |
2.45 (2.49) |
3.2 IR spectroscopy study
The application of IR spectroscopy enables the determination of bonding of ligand with emissive ion. hfpd chelates with Dy3+ ion were formed through a covalent bond with enolic oxygen(
C–O) and a coordinate bond with carbonyl oxygen (–C
O), resulting in stable complexes. Table 2 provides information of the peaks associated with the synthesized complexes. The unbound ligand (hfpd) exhibits a band ∼3400 cm−1, in relation to
C–OH stretch. Absence of this stretching vibration band in complexes indicates the chelation of Dy(III) via deprotonated ligand in its enol form (C
C–O). Additionally, peaks around 1690 and 1636 cm−1 relative to carbonyl vibration of enol-isomer and C
C stretching, respectively in free hfpd32 are displaced to 1660 cm−1 and 1434 cm−1 in Dy1–Dy4, highlighting the potential participation of C
O in coordination processes. Complexes exhibit multiple peaks corresponding to CF3 groups in the range 1144–1154 cm−1, which are observed in the range 1368–1090 cm−1 in free hfpd ligand.33
Table 2 Key findings from FT-IR spectroscopic analysis of Dy1–Dy4 (cm−1)
Complex |
Dy–O |
Dy–N |
C–H |
C–F |
P O |
C–N |
C C |
C N |
C O |
CH3 |
C–H |
Dy1 |
425 |
— |
844 |
1146 |
1204 |
— |
1427 |
— |
1663 |
2858 |
3105 |
Dy2 |
428 |
554 |
842 |
1144 |
— |
1349 |
1428 |
1556 |
1653 |
— |
3058 |
Dy3 |
426 |
546 |
851 |
1146 |
— |
1355 |
1434 |
1553 |
1651 |
2834 |
3062 |
Dy4 |
426 |
541 |
854 |
1154 |
— |
1357 |
1441 |
1542 |
1662 |
2841 |
3055 |
The free phen ligand displays the C–H bending vibration at 857 cm−1 as well as C
C and C
N ring vibrations in 1650–1400 cm−1 range.34 Discussed peaks undergo significant downward shifts in wavenumber to 842 cm−1 in mononuclear [Dy(hfpd)3phen] complex as a result of perturbation brought on by coordination of ligand to metal ion. Similarly, free TOPO exhibits a peak at 1210 cm−1 ascribed to P
O that is shifted towards 1204 cm−1 in Dy1.35 Moreover, the complexes exhibit low-intensity peaks around 425–428 cm−1, associated with Dy–O modes and at ∼546 cm−1, attributed to Dy–N coordination in Dy2–Dy4 complexes.36 Such changes in spectrum suggest that Dy(III) ion not only coordinates with carbonyl oxygen of hfpd but also directly interacts with two nitrogen atoms of phen and its derivatives in Dy2–Dy4 and two oxygen atoms of TOPO in Dy1 complex. The lack of vibrational band between 3600 and 3200 cm−1 indicates that water molecules are absent in Dy1–Dy4.37
3.3 1H NMR analysis
Proton NMR spectra of the synthesized complexes (Fig. S1–S4†) were recorded in CDCl3 at a bandwidth of 500 MHz and the obtained chemical shifts are outlined in Table 3. Salient features of the spectra are as follows: (i) in comparison to uncoordinated ligands, the complexes exhibit significant upfield and downfield shifts38 (ii) instead of the anticipated 4 peaks, complex Dy2 displayed only 3 peaks corresponding to phen. Notably, one proton signal was absent within the range of +200 to −200 ppm, owing to the higher magnetic moment of dysprosium ion and resulting in paramagnetically enhanced relaxation of this nucleus (iii) opposite shift directions are observed for methine protons (hfpd) and ancillary ligand protons in Dy1–Dy4 (iv) the absence of a signal relative to enolic OH of hfpd in the spectral profiles of complex implies the binding of the Dy3+ ion to the oxygen atom of hfpd.26,39
Table 3 Proton δ (ppm) data of Dy1–Dy4
Complex |
Peaks relative to hfpd |
Peaks relative to ancillary moiety |
hfpd |
10.85 (-OH), 6.48 (-C-H) |
— |
Dy1 |
101.47 (3H) |
−1.48 (18H), −3.42 & −3.81 (60H), −5.89 (12H), −7.72 (12H) |
Dy2 |
117.36 (3H) |
−28.72 (2H), −42.64 (2H), −60.90 (2H) |
Dy3 |
134.10 (3H) |
−1.34 (6H), −32.85 (2H), −48.11 (2H), −62.86 (2H) |
Dy4 |
128.08 (3H) |
−2.19 (6H), −33.22 (2H), −48.18 (2H), −61.39 (10H) |
3.4 UV-vis absorption spectroscopy
Fig. 2 illustrates room temperature electronic spectra of free hfpd and Dy1–Dy4, captured in 1 × 10−5 mol L−1 dichloromethane solution. The uncoordinated hfpd displays a prominent absorption band at 279 nm, predominantly associated with spin-allowed π → π* transitions. In contrast, the UV-vis absorption spectra of Dy1–Dy4, besides the UV absorption band at 243–277 nm, reveal a broad maxima at 287–314 nm owing to corresponding transitions of π → π* and n → π*. The initial band in each spectrum (shorter-wavelength band), corresponds to the ancillary ligand.40 Complexation leads to a red-shift in the absorption bands of β-diketone ligands, revealing stabilization of their orbitals (nephelauxetic effect).41 Table 4 comprises of absorption maxima corresponding to Dy1–Dy4.
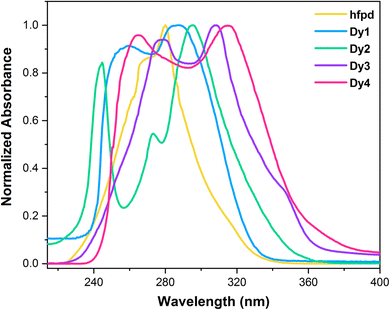 |
| Fig. 2 Absorption spectra of free hfpd and Dy1–Dy4 complexes. | |
Table 4 Optical parameters for Dy1–Dy4
Complex |
aλmaxabs |
aEg |
aλex |
aλem |
λ and E in nm and eV, respectively. |
Dy1 |
286 |
3.87 |
279, 371 |
485, 575, 661 |
Dy2 |
295 |
3.79 |
278, 370 |
486, 574, 662 |
Dy3 |
308 |
3.70 |
279, 372 |
486, 574, 660 |
Dy4 |
314 |
3.55 |
302, 374 |
574, 662 |
For Dy1–Dy4 complexes, the determined molar absorption coefficients (ε) are 1.4 × 104, 2.91 × 104, 6.24 × 104, and 1.07 × 105, respectively (Fig. S5†). Compared to the ε of free hfpd, which is 6.7 × 103,42 these values are approximately threefold higher. This result shows that inclusion of ancillary ligands not only meets the coordination needs of the Dy(III) ion but also enhances the absorption intensity. The lowest ε value observed for complex Dy1 can be attributed to the lack of an extended π-system, resulting in less efficient light absorption. In contrast, Dy4 exhibits the highest ε value due to its more extended π-system, which significantly enhances its ability to absorb light. Band gap (Eg) of aforementioned compounds is also measured utilizing eqn (1) i.e. Tauc's equation, on data obtained from electronic spectra:43
Here,
α denotes absorptivity,
hυ denotes energy of photon,
A is used to signify the band edge parameter, while
Eg symbolizes the optical band gap. The parameter
n is a transition-dependent factor with a value of 1/2 for indirect and 2 for direct excitation.
44 Eg values of
Dy1–Dy4 were measured by the help of Tauc plot between energy and (
αhυ)
2 as shown in
Fig. 3. This was accomplished by extrapolation of straight line to (
αhυ)
2 = 0.
Eg of
Dy1–Dy4 complexes in eV are detected as 3.87, 3.79, 3.70 and 3.55, respectively. This decreasing order in the band gap is attributed to the increasing conjugation in synthesized complexes. The effectiveness of the considered complexes in display devices is closely tied to
Eg values falling in semiconductor range, contributing to enhanced performance in electronic technologies.
45
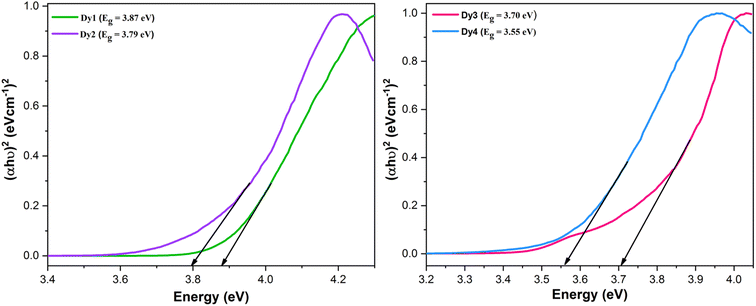 |
| Fig. 3 Tauc's plots and band gap values of Dy1–Dy4 complexes. | |
3.5 Photoluminescence spectroscopy investigations
3.5.1 Excitation spectra. To analyze photoluminescent behaviour exhibited by Dy1–Dy4, solid samples were excited using emission wavelengths (λem) of 480 nm (4F9/2 → 6H15/2) and 575 nm (4F9/2 → 6H13/2). However, the spectra recorded at 575 nm was more intense in comparison to the one recorded at 480 nm. Normalized excitation spectrum (λem = 575 nm) (Fig. 4) of each complex revealed ligand based broad bands ∼280 and ∼370 nm (Table 4) relative to energy required for an electron to jump from π to π*.46,47 Since, no metal centered peaks were observed in the excitation spectra and the spectral profiles are dominated by ligand's absorption, it suggests that sensitization in Dy1–Dy4 happened via indirect energy transfer [ligand → Dy(III)]. The excitation spectra of Dy1–Dy4 exhibit different intensities with Dy3 being most intense and Dy4 being least intense.
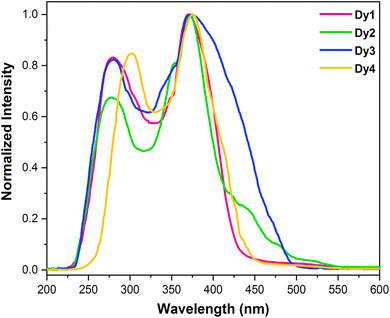 |
| Fig. 4 Normalized excitation spectra of solid Dy1–Dy4 at room temperature at λem = 575 nm. | |
3.5.2 Emission spectra. Fig. 5 contains the normalized emission profiles of solid Dy1–Dy4 complexes. The spectral profiles exhibit emission peaks (Table 4) associated with 4F9/2 → 6Hx/2 (x = 15, 13, 11) and π–π* transitions of organic moieties, upon excitation at ∼370 nm. These peaks correspond to three different electronic transitions: a blue, magnetic-dipole (MD) transition (4F9/2 → 6H15/2) at ∼486 nm; a yellow, electric-dipole (ED) transition (4F9/2 → 6H13/2) at ∼574 nm and a least intense peak at ∼661 nm.48,49 The transition at ∼574 nm is most intense and sensitive to its environment. The greater intensity of transition centered at 574 nm indicates that complexes are situated in low molecular symmetry and lack inversion centre. This makes the yellow transition a valuable reference for assessing symmetry in such systems. While magnetically allowed blue emission (4F9/2 → 6H15/2) remains unaffected by the local environment surrounding Dy(III) ion.50 A broad band at lower wavelength is exhibited by all the complexes due to transition from ligand's excited state to ground state indicating that the ligands do not transfer the absorbed energy effectively to the emissive state of metal ion which is discussed in detail in Section 3.5.7. Moreover, the emission spectrum of Dy4 exhibits only 2 metal based peaks (at 574 nm and 662 nm) along with a broad ligand based band which merged with the third peak (485 nm).
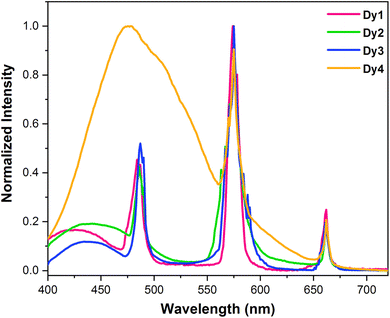 |
| Fig. 5 Normalized emission spectra of solid Dy1–Dy4 obtained at room temperature with λex = ∼370 nm. | |
3.5.3 Branching ratio. Calculating the branching ratio (β) involves the comparison between radiative transition probability for each peak and combined radiative transition probability of all peaks as expressed in eqn (2).51 |
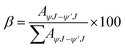 | (2) |
Here, AψJ–ψ′J denotes the total area under the observed peak in photoluminescence emission (PLE) spectrum of Dy1–Dy4 compounds. The importance of β in laser design lies in its role in determining the chances of stimulated emission from particular emission transitions. A greater β value indicates a higher suitability for achieving stimulated emission cross-section.52 Table 5 presents the calculated branching ratios for Dy1–Dy4. Analysis of β ratios reveals a predominance of ΔJ = 2 transition, accounting for ∼60% of the total emission in Dy1–Dy3. This suggests its suitability for laser amplification.53 The yellow emission originating from ΔJ = 2 transition dominates the luminescence spectra, followed by blue light of ΔJ = 3 transition and lastly, red emission from ΔJ = 1 transition. Intensity ratio of yellow [Y] (ΔJ = 2) to blue [B] (ΔJ = 3) emission lines reflects neighbouring environment experienced by Dy(III) ion in Dy1–Dy4.54 In this study, the Y/B ratio is pointing towards a highly asymmetric surrounding in the solid phase.
Table 5 Branching ratio of characteristic peaks for dysprosium and intensity ratio, lifetime and quantum yield across Dy1–Dy4
Complex |
Branching ratio |
Intensity ratio |
Lifetime (ms) |
Quantum yield (%) |
Ligand based band |
ΔJ = 3 |
ΔJ = 2 |
ΔJ = 1 |
Dy1 |
11.894 |
23.441 |
54.992 |
9.671 |
2.34 |
0.46 |
1.9 |
Dy2 |
15.703 |
11.605 |
68.556 |
4.134 |
5.90 |
0.65 |
2.7 |
Dy3 |
15.069 |
16.997 |
61.525 |
6.407 |
3.61 |
0.76 |
3.4 |
Dy4 |
90.616 |
7.987 |
1.395 |
— |
0.37 |
1.2 |
3.5.4 Decay analysis and quantum yield. Fig. 6 depicts emission lifetime curves associated with Dy1–Dy4 acquired by examining emission at 574 nm with excitation at about 370 nm. These curves were properly fitted by a single exponential function (eqn (3)),55 yielding lifetime of τ (in ms) = 0.46, 0.65, 0.76 and 0.37 for Dy1–Dy4, respectively. This result strongly supports the existence of a single emissive center within the complexes.56
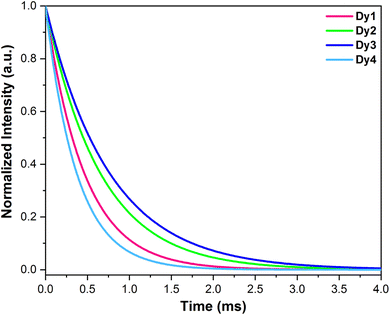 |
| Fig. 6 Luminescence decay profiles of Dy1–Dy4 complexes. | |
The above relation expresses exponential decay of intensity I over time t, characterized by the decay time constant τ and initial intensity I0. It is noteworthy to emphasize that upon coordinating three hfpd units (possessing low-energy C–F oscillators) alongside auxiliary ligands enables the emissive ions in these complexes to attain coordinative saturation. Additionally, these ligands shield the metal ion from external disturbances. Table 5 presents the data that was obtained through this analysis.
Photoluminescence quantum yield quantifies the proportion of absorbed photons that are re-emitted, typically in the visible spectrum, through the process of photoluminescence. To understand the effect of ancillary ligands on the photoluminescent characteristics, the luminescence quantum yields of the synthesized complexes were calculated using eqn (4) and are tabulated in Table 5. These calculations were carried out in DCM against quinine bisulphate in 1 M H2SO4 (Φr = 0.546).57,58
|
 | (4) |
Here,
Φ,
A,
I,
n,
r and
s are quantum yield, absorbance at excitation wavelength, integrated emission intensity, refractive index, reference and sample, respectively.
59 Complex
Dy4 exhibited lowest quantum yield which could be attributed to back energy transfer whereas highest quantum yield amongst the synthesized samples was exhibited by
Dy3 suggesting better sensitizing nature of neoc unit.
3.5.5 Color parameters. Evaluation of emitted color and its quality from the solid Dy3+ complexes involved the use of CIE color coordinates and Correlated Color Temperature (CCT) values, as detailed in Table 6 and Fig. 7. The emission spectrum was employed to calculate x and y (CIE color coordinates), providing information on the color emitted by these ternary complexes. The conversion from x, y coordinates to 1976 CIE uniform chromaticity coordinates (u′, v′) (Fig. 8) was accomplished by employing eqn (5), utilizing the x, y values determined previously.60,61 |
 | (5) |
Table 6 Color Characteristics corresponding to Dy1 to Dy4
Complex |
(x, y) |
(u′, v′) |
CCT |
Dy1 |
(0.3191, 0.3143) |
(0.2081, 0.4612) |
6240.99 |
Dy2 |
(0.3330, 0.3305) |
(0.2114, 0.4722) |
5467.00 |
Dy3 |
(0.3545, 0.3714) |
(0.2101, 0.4954) |
4733.92 |
Dy4 |
(0.2206, 0.3086) |
(0.1409, 0.4435) |
>10 000 |
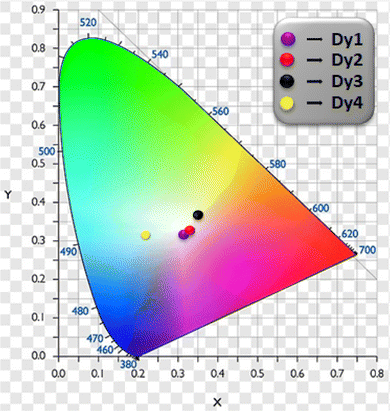 |
| Fig. 7 CIE 1931 color coordinates of Dy1–Dy4 complexes. | |
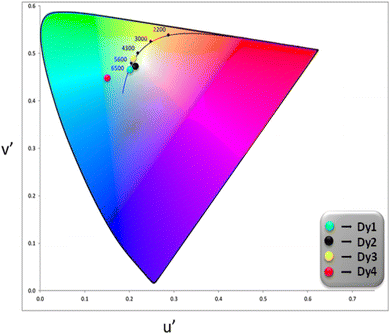 |
| Fig. 8 CIE 1976 color coordinates for Dy1–Dy4 complexes. | |
CCT serves as a standard for assessing the properties of light imparted by a light source, playing a critical role in alleviating mental and visual stress. The careful selection of an appropriate CCT is crucial for ensuring effective lighting. Generally, a CCT value below 3200 K suggests warm white light, ideal for residential use, while a value exceeding 4000 K implies cold white light, often preferred for commercial lighting.62,63 Calculation of these values was performed using McCamy's equation (eqn (6)):64,65
|
CCT = −437n3 + 3601n2 − 6861n + 5514.31
| (6) |
The variable n in the provided equation corresponds to the inverse slope of line and its calculation can be executed according to eqn (7).66,67
|
 | (7) |
Here,
xe (0.332) and
ye (0.186) denote color epicentre. Complexes with ligands, TOPO, phen and neoc, are emitting in the region of cold white light at
λex = 370 nm. The CCT values recorded for these complexes are between 4733 to 6240 K, indicating the complexes' potential for applications in cold white light, particularly in commercial lighting. CIE data shows
Dy2 emits white light (CIE
x,
y ≈ 0.333), with CCT around 5467.004 K.
Dy1 leans towards cold-white (bluish), while
Dy3 exhibits cold-white (yellowish) emission. However, subtle differences emerge for
Dy4 due to presence of a broad ligand based emission band in blue region. This color tunability is attained by modifying the coordination environment with various ancillary ligands.
3.5.6 Dependence of color coordinates on excitation wavelength. In an attempt to study the potential modulation of color in CIE graphs, the photoluminescence spectra of Dy3 complex was recorded at various excitation wavelengths (250 nm to 380 nm). The resulting emission data was used to generate the emission map (Fig. 9). It was observed that the intensity of the emission spectra varies with the excitation wavelength, indicating that the emissive color coordinates are also influenced by the excitation wavelength. The emission data was analyzed to determine the x and y color coordinates (Table S1†) which were subsequently plotted on a CIE 1931 diagram for color visualization (Fig. 10). Utilizing eqn (4), the u′, v′ chromaticity coordinates were calculated and plotted on CIE 1976 color triangle (Fig. S6†). The dependence of color coordinates on excitation wavelength is shown in Fig. 10 & S6.† This comprehensive analysis provides insights into the tunability of emission properties and the potential for modulating the color output paving the way for future applications in color-tunable photonic devices.
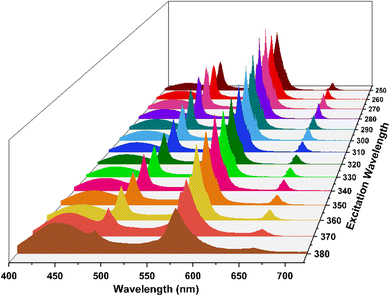 |
| Fig. 9 Emission Map of Dy3 at different excitation wavelengths. | |
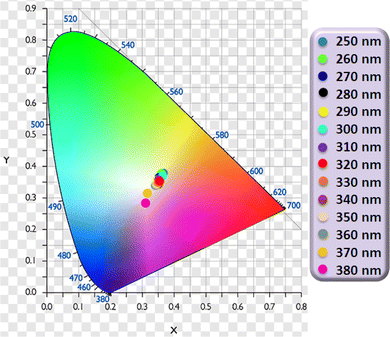 |
| Fig. 10 CIE 1931 color coordinates for Dy3 complex at different λex. | |
3.5.7 Energy transfer mechanism. Understanding the photoluminescent behaviour of Dy(III) complexes requires careful examination of energy transfer pathways between dysprosium ion and coordinated ligands. Intramolecular energy transfer (IET) mechanism described by Dexter and Sato et al. states that two processes are mainly responsible for the IET efficiency.68,69 (i) Through resonant exchange interaction, energy migrates from T1 of organic ligand to emissive level of Ln3+ ion. (ii) Through a process known as thermal deactivation, energy is migrated inversely from Ln ion back to organic moiety. Back energy transfer (BET) predominates when the energy difference (ΔE) is too small for coordinated ligands and metal ion. ΔE plays a very significant role in energy transfer via above mentioned processes. However inefficient ligand-to-metal sensitization due to BET can be overcome by vibrationally-assisted energy transfer.70 This holds particular significance in Dy3+ coordination compounds, having main and long-lived emitting level, 4F9/2, positioned at approximately 20
833 cm−1 which is relatively higher when compared to the T1 of the majority of aromatic organic ligands. However, Dy(III) complexes lacks a reported optimal energy gap as suggested by Latva that would support efficient IET.71The energy levels of the ligands (employed in complexation in Dy1–Dy4) in their triplet states are 21
978 cm−1 (TOPO),72 22
100 cm−1 (phen),73 22
624 cm−1 (neoc),74 20
163 cm−1 (bathoc)75 and 21
930 cm−1 (hfpd).76 The ΔE between emissive level of Dy(III) ion and T1 of ligands is 1145 cm−1 (TOPO), 1267 cm−1 (phen), 1791 cm−1 (neoc), −670 cm−1 (bathoc) and 1097 cm−1 (hfpd). Energy transfer process in considered complexes is shown in Fig. 11. The relatively small ΔE is the contributing factor to BET from Dy3+ to organic ligands which is observed as a ligand based band in the emission spectrum of all the complexes. The most favourable ΔE has been observed for Dy3 complex which results in least BET and most intense emission (Fig. S7 and S8†). While, least favourable ΔE is found for Dy4 where the T1 state of the ancillary ligand is positioned lower than the emissive state of the dysprosium ion, hindering efficient IET and promoting BET, resulting in a broad ligand based emission. Between Dy1 and Dy2 complex, better luminescence enhancement of Dy(III) ion is observed in Dy2 owing to higher energy of T1 state of phen in comparison to T1 state of TOPO. Above that, the overall intensites of these complexes is low in comparison to the other reported compounds with europium, terbium and samarium metal ion, owing to less ΔE between emissive state of Dy and T1 state of hfpd.26
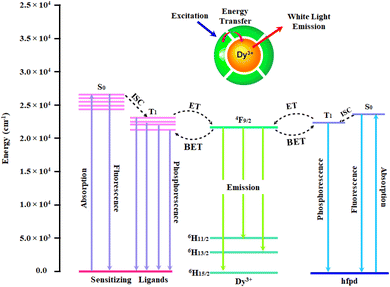 |
| Fig. 11 Schematic diagram showing energy levels and energy transfer pathway in Dy1–Dy4 (S0: Singlet; T1: Triplet; ISC: Inter System Crossing; ET: Energy Transfer; BET: Back Energy Transfer). | |
3.6 CV analysis
For the purpose of studying electrochemical characteristics of synthesized Dy1–Dy4 complexes, CV scans were recorded in DCM utilizing Fc/Fc+ (ferrocene/ferricenium) redox couple ion as internal standard. A three-electrode system comprising of working electrode (glassy carbon), reference electrode (Ag/AgCl) and a counter electrode (Pt. wire) were employed along with supporting electrolyte (Tetra-n-butylammonium perchlorate) to obtain the CV curves (Fig. 12).
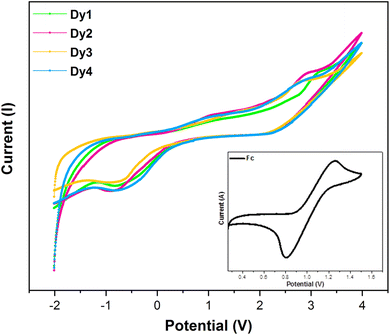 |
| Fig. 12 CV profiles of Dy1 and Dy4, with the inset displaying the CV profile of ferrocene. | |
Utilizing data obtained from a CV plot, the oxidation (Eox) and reduction (Ered) potentials were determined, resulting in values of 3.12 V (Dy1), 2.89 V (Dy2), 2.78 V (Dy3), 2.80 V (Dy4) for oxidation and −0.79 V (Dy1), −0.88 V (Dy2), −0.86 V (Dy3), −0.79 V (Dy4) for reduction. All the complexes exhibited a reversible electrochemical behaviour. Estimation of the energy of frontier molecular orbitals (FMO) involves the incorporation of the Eox and Ered values into eqn (8) and (9).77,78
|
EHOMO = −[(Eox − E1/2(ferrocene)) + 4.8] eV
| (8) |
|
ELUMO = −[(Ered − E1/2(ferrocene)) + 4.8] eV
| (9) |
The CV data of trivalent dysprosium complexes are tabulated in Table 7. E1/2 of Fc as determined from their CV curve is equal to 1.03 V.79 From values of FMOs, electronic band gap (Ebg) of Dy1–Dy4 complexes was calculated which exhibited a decreasing order by virtue of extended pi-electron conjugation. The electronic band gap values ranging from 3 to 4 eV confirm their semiconducting properties, making them suitable for application in display devices.
Table 7 Comparative analysis of redox potential and electronic Ebg for Dy1–Dy4
Complex |
aEox |
aEred |
aEHOMO |
aELUMO |
aEbg |
Eox and Ered are in (V); EHOMO, ELUMO and Ebg are in (eV). |
Dy1 |
3.12 |
−0.79 |
−6.89 |
−2.98 |
3.91 |
Dy2 |
2.89 |
−0.88 |
−6.66 |
−2.89 |
3.77 |
Dy3 |
2.78 |
−0.86 |
−6.55 |
−2.91 |
3.64 |
Dy4 |
2.80 |
−0.79 |
−6.57 |
−2.98 |
3.59 |
3.7 Thermal characterization
One of the key advantages of lanthanide complexes for use in optoelectronic devices is their remarkable thermal stability. This stability is clearly evident in Fig. 13, which shows the thermogravimetric (TG) plot over a wide range (25–650 °C). As the TG plot demonstrates, Dy4 remains stable up to a temperature of 208 °C. However, at 210 °C and 578 °C, inflection point was observed on the TG plot, corresponding to 88.43% (calcd.: 86.06%) weight loss. This sharp change in weight indicates decomposition of Dy4. This remarkable stability makes it a promising candidate for various applications in optoelectronic devices. Above 617 °C, dysprosium oxide (Dy2O3) is identified as the stable oxide residue. The absence of an inflection point in the thermogram before 200 °C implies the absence of water, whether crystalliferous or coordinated.80,81
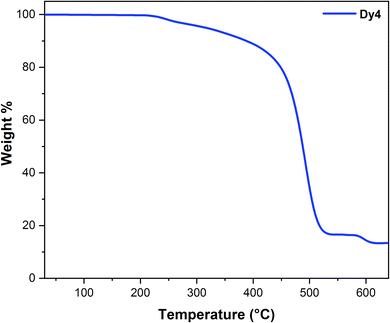 |
| Fig. 13 Thermogravimetric curve of Dy4 complex. | |
4 Conclusions
Heteroleptic ternary anhydrous dysprosium fluorinated β-diketonates were synthesized in one-step using four different auxiliary ligands of varying denticities. The triplet (T1) states of ligands show close alignment with the emissive level of Dy3+ ion (4F9/2) which induced BET from this emissive state to the excited states of ligand. Consequently, this phenomenon gave rise to ligand based emission in blue region along with yellow-blue emission from Dy3+ ion. This characteristic was used to achieve near white-light emission. The observed white light emission (WLE) of Dy1 (x = 0.3191, y = 0.3143), Dy2 (0.3330, 0.3305) and Dy3 (0.3545, 0.3714) is in close proximity to ideal white light, with CCT values in cold white-light region. From the study, it was found that the yellow emission in synthesized complexes is significantly influenced by the choice of coordinating moieties. Furthermore, the color coordinates are also influenced by the excitation wavelength used while recording the emission spectra. These complexes have applications in lasers, WLEDs, photoluminescent films and optoelectronic devices due to their semiconducting nature and large thermal stability. Additionally, the potential for wider utilization in multifunctional molecular materials, such as lighting and molecular magnets, introduces an intriguing element for further design exploration.
Data availability
The authors affirm that the information/data of this research article is available inside the article.
Author contributions
Sofia Malik = data curation, writing – original draft; Komal Jakhar and Devender Singh = writing – review & editing and supervision; Anjli Hooda = formal analysis; Sonia Redhu = methodology; Swati Dalal = data curation; Vandana Aggarwal = investigation; Sumit Kumar = visualization; Rajender Singh Malik = resources and validation; Parvin Kumar = project administration.
Conflicts of interest
The authors declare that they have no known competing financial interests or personal relationships that could have appeared to influence the work reported in this paper.
Acknowledgements
Sofia Malik is thankful to UGC-New Delhi for providing JRF [221610212505].
References
- E. F. Schubert and J. K. Kim, Solid-state light sources getting smart, Science, 2005, 308, 1274–1278, DOI:10.1126/science.1108712.
- P. Kumar, S. Singh, I. Gupta, K. Nehra, V. Kumar and D. Singh, Structural refinement and optical characteristics of single-phase Gd3Al5O12: Er3+ nanophosphors for luminescent applications, J. Lumin., 2022, 252, 119338, DOI:10.1016/j.jlumin.2022.119338.
- I. Gupta, D. Singh, S. Singh, P. Kumar, S. Bhagwan and V. Kumar, Study of structural and spectroscopic characteristics of novel color tunable yellowish-white Dy3+ doped Gd4Al2O9 nanophosphors for NUV-based WLEDs, J. Mol. Struct., 2023, 1272, 134199, DOI:10.1016/j.molstruc.2022.134199.
- I. Gupta, D. Singh, S. Singh, P. Kumar, S. Bhagwan and V. Kumar, Phase recognition and spectroscopic characteristics of single-phase Tb3+ doped Gd4Al2O9 nanophosphors for NUV energized advanced photonic appliances, J. Lumin., 2022, 252, 119327, DOI:10.1016/j.jlumin.2022.119327.
- A. De Almeida, B. Santos, B. Paolo and M. Quicheron, Solid state lighting review–Potential and challenges in Europe, Renewable Sustainable Energy Rev., 2014, 34, 30–48, DOI:10.1016/j.rser.2014.02.029.
- N. T. Kalyani and S. J. Dhoble, Organic light emitting diodes: Energy saving lighting technology—A review, Renewable Sustainable Energy Rev., 2012, 16, 2696–2723, DOI:10.1016/j.rser.2012.02.021.
- T. S. Mahapatra, H. Singh, A. Maity, A. Dey, S. K. Pramanik, E. Suresh and A. Das, White-light-emitting lanthanide and lanthanide-iridium doped supramolecular gels: modular luminescence and stimuli-responsive behaviour, J. Mater. Chem. C, 2018, 6, 9756–9766, 10.1039/C8TC03487G.
- V. Aggarwal, D. Singh, A. Hooda, S. Malik, S. Dalal, S. Redhu, S. Kumar, R.S. Malik and P. Kumar, Comprehensive investigation of ternary dysprosium complexes for white light emission: Synthesis, spectroscopic and colorimetric analyses, J. Lumin., 2024, 270, 120555, DOI:10.1016/j.jlumin.2024.120555.
- L. Xu, Y. Li, Q. Pan, D. Wang, S. Li, G. Wang, Y. Chen, P. Zhu and W. Qin, Dual-mode light-emitting lanthanide metal–organic frameworks with high water and thermal stability and their application in white LEDs, ACS Appl. Mater. Interfaces, 2020, 12, 18934–18943, DOI:10.1021/acsami.0c02999.
- M. Chen, Y. Zhao, L. Yan, S. Yang, Y. Zhu, I. Murtaza, G. He, H. Meng and W. Huang, A Unique Blend of 2-Fluorenyl-2-anthracene and 2-Anthryl-2-anthracence Showing White Emission and High Charge Mobility, Angew. Chem., Int. Ed., 2017, 56, 722–727, DOI:10.1002/anie.201608131.
- Z. Xie, Q. Huang, T. Yu, L. Wang, Z. Mao, W. Li, Z. Yang, Y. Zhang, S. Liu, J. Xu, Z. Chi and M. P. Aldred, Hydrogen-Bonding-Assisted Intermolecular Charge Transfer: A New Strategy to Design Single-Component White-Light-Emitting Materials, Adv. Funct. Mater., 2017, 27, 1703918, DOI:10.1002/adfm.201703918.
- J. Manzur, R. C. De Santana, L. J. Q. Maia, A. Vega and E. Spodine, Tuning white light emission in dinuclear phenoxo bridged DyIII complexes, Inorg. Chem., 2019, 58, 10012–10018, DOI:10.1021/acs.inorgchem.9b01153.
- G. L. Law, K. L. Wong, H. L. Tam, K. W. Cheah and W. T. Wong, White OLED with a single-component europium complex, Inorg. Chem., 2009, 48, 10492–10494, DOI:10.1021/ic9018037.
- S. Redhu, D. Singh, A. Hooda, S. Malik, V. Aggarwal, S. Dalal, S. Kumar, R. S. Malik and P. Kumar, Synthesis, characterization and luminescent properties of N-donor based samarium-tris-β-diketonate: Tuning optoelectronic characteristics for displays applications, J. Mol. Struct., 2024, 1308, 138056, DOI:10.1016/j.molstruc.2024.138056.
- A. Dalal, K. Nehra, A. Hooda, S. Singh, S. Bhagwan, D. Singh and S. Kumar, 2, 2′-Bipyridine based fluorinated β-Diketonate Eu (III) complexes as red emitter for display applications, Inorg. Chem. Commun., 2022, 140, 109399, DOI:10.1016/j.inoche.2022.109399.
- P. P. F. da Rosa, Y. Kitagawa and Y. Hasegawa, Luminescent lanthanide complex with seven-coordination geometry, Coord. Chem. Rev., 2020, 406, 213153, DOI:10.1016/j.ccr.2019.213153.
- S. Redhu, D. Singh, A. Hooda, S. Malik, V. Aggarwal, S. Dalal, S. Kumar, R. S. Malik and P. Kumar, Photoluminescence tuning of terbium tris-1, 1, 1-trifluoro-5, 5-dimethyl-2, 4-hexanedione complexes: Synthesis, spectroscopic, thermal and electrochemical analyses, J. Lumin., 2024, 271, 120588, DOI:10.1016/j.jlumin.2024.120588.
- L. Zhong, W. B. Chen, Z. J. OuYang, M. Yang, Y. Q. Zhang, S. Gao, M. Schulze, W. Wernsdorfer and W. Dong, Unprecedented one-dimensional chain and two-dimensional network dysprosium (III) single-molecule toroics with white-light emission, Chem. Commun., 2020, 56, 2590–2593, 10.1039/C9CC08852K.
- L. Zhong, W. B. Chen, X. H. Li, Z. J. OuYang, M. Yang, Y. Q. Zhang, S. Gao and W. Dong, Four dinuclear and one-dimensional-chain dysprosium and terbium complexes based on 2-Hydroxy-3-methoxybenzoic acid: Structures, fluorescence, single-molecule-magnet, and ab initio investigation, Inorg. Chem., 2020, 59, 4414–4423, DOI:10.1021/acs.inorgchem.9b03555.
- D. Mara, L. Pilia, M. Van de Steen, I. Miletto, M. Zeng, K. Van Hecke, A. Serpe, P. Deplano, R. Van Deun and F. Artizzu, Single-component panchromatic white light generation, and tuneable excimer-like visible orange and NIR emission in a Dy quinolinolate complex, J. Mater. Chem. C, 2021, 9, 15641–15648, 10.1039/D1TC04191F.
- S. Redhu, D. Singh, K. Nehra, S. Dalal, S. Malik, V. Aggarwal, S. Kumar, R. S. Malik, P. Kumar and J. Sindhu, NUV-excitation Dy (III) complexes: Synthesis, structural study and impact of secondary ligands on optoelectronic properties, J. Mol. Struct., 2024, 1311, 138380, DOI:10.1016/j.molstruc.2024.138380.
- A. Hooda, K. Nehra, A. Dalal, S. Singh, R. K. Saini, S. Kumar and D. Singh, Terbium complexes of an asymmetric β-diketone: preparation, photophysical and thermal investigation, Inorg. Chim. Acta, 2022, 536, 120881, DOI:10.1016/j.ica.2022.120881.
- R. F. Li, R. H. Li, X. F. Liu, X. H. Chang and X. Feng, Lanthanide complexes based on a conjugated pyridine carboxylate ligand: structures, luminescence and magnetic properties, RSC Adv., 2020, 10, 6192–6199, 10.1039/C9RA10975G.
- K. Nehra, A. Dalal, A. Hooda, S. Bhagwan, R. K. Saini, B. Mari, S. Kumar and D. Singh, Lanthanides β-diketonate complexes as energy-efficient emissive materials: A review, J. Mol. Struct., 2022, 1249, 131531, DOI:10.1016/j.molstruc.2021.131531.
- S. Ahmad Bhat and K. Iftikhar, Optical Properties of New Terbium (III) Ternary Complexes Containing Anionic 6, 6, 7, 7, 8, 8, 8-heptafluoro-2, 2-dimethyl-3, 5-octanedione and Neutral Sensitizers in Solution, Solid and PMMA Thin Films: Intra and Interphase Colour Tuning, J. Photochem. Photobiol., 2021, 97, 688–699, DOI:10.1111/php.13382.
- Z. Ahmed and K. Iftikhar, Synthesis, luminescence and NMR studies of lanthanide (III) complexes with hexafluoroacetylacetone and phenanthroline. Part II, Inorg. Chim. Acta, 2012, 392, 165–176, DOI:10.1016/j.ica.2012.06.032.
- J. Rocha, C. D. Brites and L. D. Carlos, Lanthanide organic framework luminescent thermometers, Chem. - Eur. J., 2016, 22, 14782–14795, DOI:10.1002/chem.201600860.9756.
- A. Dalal, K. Nehra, A. Hooda, S. Singh, S. Bhagwan, D. Singh and S. Kumar, 2, 2′-Bipyridine based fluorinated β-Diketonate Eu (III) complexes as red emitter for display applications, Inorg. Chem. Commun., 2022, 140, 109399, DOI:10.1016/j.inoche.2022.109399.
- D. Mara, F. Artizzu, B. Laforce, L. Vincze, K. Van Hecke, R. Van Deun and A. M. Kaczmarek, Novel tetrakis lanthanide β-diketonate complexes: Structural study, luminescence properties and temperature sensing, J. Lumin., 2019, 213, 343–355, DOI:10.1016/j.jlumin.2019.05.035.
- L. Ruan, X. Gao, J. Zhao, C. Xu and D. Liang, Preparation and characteristics of Eu(DBM)3phen: Synthesis, single-crystal structure and spectroscopic analysis, J. Mol. Struct., 2017, 1149, 265–272, DOI:10.1016/j.molstruc.2017.08.003.
- A. Hooda, D. Singh, K. Nehra, S. Dalal, V. Aggarwal, S. Kumar, R. S. Malik and P. Kumar, Preparation and optical studies of octacoordinated luminescent complexes of Dy(III) derived from fluorinated β-diketone and heteroaromatic neutral ligands, Inorg. Chim. Acta, 2023, 556, 121674, DOI:10.1016/j.ica.2023.121674.
- H. Ogoshi and K. Nakamoto, Normal-Coordinate Analyses of Hydrogen-Bonded Compounds. V. The Enol Forms of Acetylacetone and Hexafluoroacetylacetone, J. Chem. Phys., 1966, 45, 3113–3120, DOI:10.1063/1.1728068.
- S. Dasari, S. Singh, P. Kumar, S. Sivakumar and A. K. Patra, Near-infrared excited cooperative upconversion in luminescent Ytterbium (III) bioprobes as light-responsive theranostic agents, Eur. J. Med. Chem., 2019, 163, 546–559, DOI:10.1016/j.ejmech.2018.12.010.
- M. Sengar and A. K. Narula, Luminescence sensitization of Eu (III) complexes with aromatic Schiff base and N, N’-donor heterocyclic ligands: synthesis, luminescent properties and energy transfer, J. Fluoresc., 2019, 29, 111–120, DOI:10.1007/s10895-018-2315-3.
- S. Malik, K. Jakhar, D. Singh, S. Dalal, A. Hooda, K. Nehra, S. Kumar, R. S. Malik and P. Kumar, Exploring the role of neutral ligands in modulating the photoluminescence of samarium complexes with 1, 1, 1, 5, 5, 5-hexafluoro-2, 4-pentanedione, Luminescence, 2024, 39, e4810, DOI:10.1002/bio.4810.
- L. Li, J. Gou, D. F. Wu, Y. J. Wang, Y. Y. Duan, H. H. Chen, H. L. Gao and J. Z. Cui, Near-infrared luminescence and magnetic properties of dinuclear rare earth complexes modulated by β-diketone co-ligands, New J. Chem., 2020, 44, 3912–3921, 10.1039/D0NJ00164C.
- R. Ilmi and K. Iftikhar, Optical emission studies of new europium and terbium dinuclear complexes with trifluoroacetylacetone and bridging bipyrimidine. Fast radiation and high emission quantum yield, Polyhedron, 2015, 102, 16–26, DOI:10.1016/j.poly.2015.07.046.
- A. Hooda, A. Dalal, K. Nehra, D. Singh, S. Kumar, R. S. Malik and P. Kumar, Preparation and optical investigation of green luminescent ternary terbium complexes with aromatic β-diketone, Chem. Phys. Lett., 2022, 794, 139495, DOI:10.1016/j.cplett.2022.139495.
- Z. Ahmed and K. Iftikhar, Sensitization of visible and NIR emitting lanthanide (III) ions in noncentrosymmetric complexes of hexafluoroacetylacetone and unsubstituted monodentate pyrazole, J. Phys. Chem. A, 2013, 117, 11183–11201, DOI:10.1021/jp403668j.
- Y. Y. Duan, D. F. Wu, H. H. Chen, Y. J. Wang, L. Li, H. L. Gao and J. Z. Cui, New dinuclear lanthanide complexes derived from Schiff base ligand and β-diketonate co-ligand: Synthesis, crystal structures, luminescent and magnetic properties, Polyhedron, 2022, 225, 116070, DOI:10.1016/j.poly.2022.116070.
- R. Ilmi and K. Iftikhar, Synthesis and photoluminescence
properties of pink luminescent heteroleptic Sm(III) complexes; the role of DMSO in transforming the inner coordination sphere and on photophysical properties. Sparkle/RM1 calculation, Polyhedron, 2017, 127, 191–202, DOI:10.1016/j.poly.2017.01.035.
- Z. Ahmed and K. Iftikhar, Efficient photoluminescent complexes of 400–1800 nm wavelength emitting lanthanides containing organic sensitizers for optoelectronic devices, RSC Adv., 2014, 4, 63696–63711, 10.1039/C4RA11330F.
- D. Singh, K. Nehra, R. K. Saini, A. Dalal, S. Bhagwan, K. Singh, A. P. Simantilleke and S. Kumar, Luminescence intensification of terbium(III) ion complexes with dipivaloylmethane (tmhd) and monodentate auxiliary ligands, Optik, 2020, 206, 164338, DOI:10.1016/j.ijleo.2020.164338.
- S. Dalal, D. Singh, A. Dalal, A. Hooda, S. Malik, S. Kumar, R. S. Malik, P. Kumar and J. Sindhu, Samarium (III) complexes with tunable luminescence: efficient sensitization and semiconducting properties for optoelectronic devices, J. Mater. Sci.: Mater. Electron., 2024, 35, 632, DOI:10.1007/s10854-024-12353-6.
- A. Dalal, K. Nehra, A. Hooda, P. Kumar, D. Singh, S. Kumar, R. Kumar and P. Kumar, Red emissive β-diketonate Ln (III) complexes for displays: Preparation, spectroscopic and optical investigations, Optik, 2023, 276, 170648, DOI:10.1016/j.ijleo.2023.170648.
- H. L. Wang, X. F. Ma, H. H. Zou, K. Wang, B. Li, Z. L. Chen and F. P. Liang, Mixed chelating ligands used to regulate the luminescence of Ln (III) complexes and single-ion magnet behavior in Dy-based analogues, Dalton Trans., 2018, 47, 15929–15940, 10.1039/C8DT03133A.
- W. A. Dar, Z. Ahmed and K. Iftikhar, Cool white light emission from the yellow and blue emission bands of the Dy (III) complex under UV-excitation, J. Photochem. Photobiol., A, 2018, 356, 502–511, DOI:10.1016/j.jphotochem.2017.12.017.
- A. Hooda, D. Singh, A. Dalal, S. Malik, S. Redhu, K. Jakhar, S. Kumar, R. S. Malik and P. Kumar, Preparation and spectral features of Dy(III) β-Diketonates with m,m′-Disubstituted N-donor aromatic auxiliary moieties for displays, Inorg. Chem. Commun., 2023, 155, 111018, DOI:10.1016/j.inoche.2023.111018.
- I. Gupta, S. Singh, P. Kumar, S. Bhagwan, V. Kumar and D. Singh, Structural, morphological and optoelectronic aspects of YAlO3: Dy3+ doped nanocrystalline materials for NUV energized WLEDs, Curr. Appl. Phys., 2022, 43, 78–89, DOI:10.1016/j.cap.2022.08.011.
- F. Gu, S. F. Wang, M. K. Lü, G. J. Zhou, D. Xu and D. R. Yuan, Structure evaluation and highly enhanced luminescence of Dy3+-doped ZnO nanocrystals by Li+ doping via combustion method, Langmuir, 2004, 20, 3528–3531, DOI:10.1021/la049874f.
- S. Dalal, D. Singh, A. Hooda, S. Redhu, S. Malik, V. Aggarwal, S. Kumar, R. S. Malik, P. Kumar and J. Sindhu, Enhancement in photophysical properties of octacoordinated Dy (III) complexes via energy transfer from photosensitizing ligands, Opt. Mater., 2024, 150, 115159, DOI:10.1016/j.optmat.2024.115159.
- W. A. Dar, A. B. Ganaie and K. Iftikhar, Synthesis and photoluminescence study of two new complexes [Sm(hfaa)3(impy)2] and [Eu(hfaa)3(impy)2] and their PMMA based hybrid films, J. Lumin., 2018, 202, 438–449, DOI:10.1016/j.jlumin.2018.05.032.
- A. Pereira, G. Conte, A. D. Faceto, L. A. Nunes, W. G. Quirino, C. Legnani, H. Gallardo, M. Cremona, I. H. Bechtold and F. E. Guimarães, Influence of nonradiative Auger process in the lanthanide complexes lifetime near interfaces in organic light-emitting diode structures, J. Appl. Phys., 2019, 126, 165501, DOI:10.1063/1.5099014.
- A. Hooda, A. Dalal, K. Nehra, S. Singh, S. Kumar and D. Singh, Red-emitting β-diketonate Eu(III) Complexes With Substituted 1,10-phenanthroline Derivatives: Optoelectronic and Spectroscopic Analysis, J. Fluoresc., 2022, 32, 1413–1424, DOI:10.1007/s10895-022-02951-0.
- H. F. Li, P. F. Yan, P. Chen, Y. Wang, H. Xu and G. M. Li, Highly luminescent bis-diketone lanthanide complexes with triple-stranded dinuclear structure, Dalton Trans., 2012, 41, 900–907, 10.1039/C1DT11496D.
- S. Malik, K. Jakhar, D. Singh, A. Hooda, K. Nehra, S. Kumar, R. S. Malik and P. Kumar, Near ultra-violet excitable Tb (III)-tris-hexafluoro-2, 4-pentanedione complexes for OLEDs: Insights into the impact of ancillary ligands on photoluminescent characteristics, J. Mol. Struct., 2024, 1311, 138334, DOI:10.1016/j.molstruc.2024.138334.
- K. Nehra, A. Dalal, A. Hooda, K. Jakhar, D. Singh and S. Kumar, Preparation, optoelectronic and spectroscopic analysis of fluorinated heteroleptic samarium complexes for display applications, Inorg. Chim. Acta, 2022, 537, 120958, DOI:10.1016/j.ica.2022.120958.
- K. Nehra, A. Dalal, A. Hooda, S. Singh, D. Singh and S. Kumar, Spectroscopic and optical investigation of 1, 10-phenanthroline based Tb (III) β-diketonate complexes, Inorg. Chim. Acta, 2022, 536, 120860, DOI:10.1016/j.ica.2022.120860.
- A. Dalal, K. Nehra, A. Hooda, D. Singh, K. Jakhar and S. Kumar, Preparation and photoluminescent characteristics of green Tb (III) complexes with β-diketones and N donor auxiliary ligands, Inorg. Chem. Commun., 2022, 139, 109349, DOI:10.1016/j.inoche.2022.109349.
- S. Singh, A. P. Simantilleke and D. Singh, Crystal structure and photoluminescence investigations of Y3Al5O12:Dy3+ nanocrystalline phosphors for WLEDs, Chem. Phys. Lett., 2021, 765, 138300, DOI:10.1016/j.cplett.2020.138300.
- A. Dalal, A. Hooda, K. Nehra, D. Singh, S. Kumar, R. S. Malik and P. Kumar, Effect of substituted 2,2’ -bipyridine derivatives on luminescence characteristics of green emissive terbium complexes: Spectroscopic and optical analysis, J. Mol. Struct., 2022, 1265, 133343, DOI:10.1016/j.molstruc.2022.133343.
- O. E. Zheleznikova, S. V. Prytkov and A. M. Kokinov, Selection of optimal method of correlated colour temperature calculation, J. Mech. Contin. Math. Sci., 2020, 8, 134–143, DOI:10.26782/jmcms.spl.8/2020.04.00010.
- I. Gupta, S. Singh, P. Kumar, S. Bhagwan, V. Kumar and D. Singh, Structural, morphological and optoelectronic aspects of YAlO3: Dy3+ doped nanocrystalline materials for NUV energized WLEDs, Curr. Appl. Phys., 2022, 43, 78–89, DOI:10.1016/j.cap.2022.08.011.
- C. S. McCamy, Correlated color temperature as an explicit function of chromaticity coordinates, Color Res. Appl., 1992, 17, 142–144, DOI:10.1002/col.5080170211.
- P. Kumar, S. Singh, I. Gupta, A. Hooda, V. Kumar and D. Singh, Reddish-orange color tunable Sm3+ activated Gd3Al5O12 phosphors: Crystallographic and photophysical investigation for lighting applications, J. Mol. Struct., 2023, 1271, 134074, DOI:10.1016/j.molstruc.2022.134074.
- K. Nehra, A. Dalal, A. Hooda, R. K. Saini, D. Singh and S. Kumar, Synthesis and photoluminescence characterization of the complexes of samarium dibenzoylmethanates with 1,10-phenanthroline derivatives, Polyhedron, 2022, 217, 115730, DOI:10.1016/j.poly.2022.115730.
- A. Hooda, K. Nehra, A. Dalal, S. Singh, R. K. Saini, S. Kumar and D. Singh, Deep red emissive octacoordinated heteroleptic Sm (III) complexes: preparation and spectroscopic investigation, J. Mol. Struct., 2022, 1260, 132848, DOI:10.1016/j.molstruc.2022.132848.
- D. L. Dexter, A theory of sensitized luminescence in solids, J. Chem. Phys., 1953, 21, 836–850, DOI:10.1063/1.1699044.
- S. Sato and M. Wada, Relations between intramolecular energy transfer efficiencies and triplet state energies in rare earth β-diketone chelates, Bull. Chem. Soc. Jpn., 1970, 43, 1955–1962, DOI:10.1246/bcsj.43.1955.
- Y. Hasegawa and Y. Kitagawa, Luminescent lanthanide coordination polymers with transformative energy transfer processes for physical and chemical sensing applications, J. Photochem. Photobiol., C, 2022, 51, 100485, DOI:10.1016/j.jphotochemrev.2022.100485.
- C. Doffek, J. Wahsner, E. Kreidt and M. Seitz, Breakdown of the energy gap law in molecular lanthanoid luminescence: the smallest energy gap is not universally relevant for nonradiative deactivation, Inorg. Chem., 2014, 53, 3263–3265, DOI:10.1021/ic500017a.
- O. V. Kotova, S. V. Eliseeva, A. A. Volosnikov, V. A. Oleinikov, L. S. Lepnev, A. G. Vitukhnovskii and N. P. Kuz’mina, Influence of heteroligand complexation on the thermal, photoluminescent, and film-forming properties of some aromatic terbium (III) carboxylates, Russ. J. Coord. Chem., 2006, 32, 901–909, DOI:10.1134/S1070328406120086.
- H. Yu, Q. Liu, J. Li, Z. M. Su, X. Li, X. Wang, X. Wang, J. Sun, C. Zhou and X. Hu, A dual-emitting mixed-lanthanide MOF with high water-stability for ratiometric fluorescence sensing of Fe3+ and ascorbic acid, J. Mater. Chem. C, 2021, 9, 562–568, 10.1039/D0TC04781C.
- S. Malik, K. Jakhar, D. Singh, S. Kumar, R. S. Malik and P. Kumar, Optimizing hexafluoro-2, 4-pentanedione based Eu (III) complexes: A comprehensive study on the synthesis, spectroscopic characterization with Judd-Ofelt calculation, Opt. Mater., 2024, 150, 115257, DOI:10.1016/j.optmat.2024.115257.
- V. I. Adamovich, S. R. Cordero, P. I. Djurovich, A. Tamayo, M. E. Thompson, B. W. D'Andrade and S. R. Forrest, New charge-carrier blocking materials for high efficiency OLEDs, Org. Electron., 2003, 4, 77–87, DOI:10.1016/j.orgel.2003.08.003.
- R. Ilmi, D. Zhang, L. Tensi, H. Al-Sharji, N. K. Al Rasbi, A. Macchioni, L. Zhou, W. Y. Wong, P. R. Raithby and M. S. Khan, Salts of Lanthanide(III) Hexafluoroacetylacetonates [Ln= Sm(III), Eu(III) and Tb(III)] with Dipyridylammonium cations: Synthesis, characterization, photophysical properties and OLED fabrication, Dyes Pigm., 2022, 203, 110300, DOI:10.1016/j.dyepig.2022.110300.
- A. Dalal, K. Nehra, A. Hooda, D. Singh, J. Dhankhar and S. Kumar, Fluorinated β-diketone-based Sm(III) complexes: spectroscopic and optoelectronic characteristics, Luminescence, 2022, 37, 1328, DOI:10.1002/bio.4300.
- A. Hooda, A. Dalal, K. Nehra, P. Kumar, D. Singh, S. Kumar, R. S. Malik, R. Kumar and P. Kumar, Mononuclear luminous β-diketonate Ln(III) complexes with heteroaromatic auxiliary ligands: synthesis and luminescence characteristics, Luminescence, 2022, 37, 1921–1931, DOI:10.1002/bio.4376.
- K. Nehra, A. Dalal, A. Hooda, D. Singh, R. S. Malik and S. Kumar, Spectroscopic and optoelectronic investigations of 3,8-Bis(3,4-(ethylenedioxy)thien-2-yl)-1,10-phenanthroline, J. Mater. Sci.: Mater. Electron., 2022, 33, 115–125, DOI:10.1007/s10854-021-07268-5.
- M. I. Saleh, M. Y. Choo, T. W. Chan and M. R. Razali, Effect of second ligand on the luminescence of Samarium (III) dibenzoylmethane complexes: Syntheses, crystal structures, thermal analysis and luminescence study, J. Chem. Sci., 2015, 127, 2241–2249, DOI:10.1007/s12039-015-0986-8.
- A. Hooda, K. Nehra, A. Dalal, S. Singh, S. Bhagwan, K. Jakhar and D. Singh, Preparation and photoluminescent analysis of Sm3+ complexes based on unsymmetrical conjugated chromophoric ligand, J. Mater. Sci.: Mater. Electron., 2022, 33, 11132–11142, DOI:10.1007/s10854-022-08089-w.
|
This journal is © The Royal Society of Chemistry 2024 |
Click here to see how this site uses Cookies. View our privacy policy here.