DOI:
10.1039/D4RA04043K
(Review Article)
RSC Adv., 2024,
14, 26077-26090
Electrospinning technology: a promising approach for tendon–bone interface tissue engineering
Received
2nd June 2024
, Accepted 29th July 2024
First published on 19th August 2024
Abstract
The regeneration of tendon–bone interface tissue has become a topic of great interest in recent years. However, the complex nature of this interface has posed challenges in finding suitable solutions. Tissue engineering, with its potential to improve clinical outcomes and play a crucial role in musculoskeletal function, has been increasingly explored for tendon–bone interface regeneration. This review focuses on the research advancements of electrospinning technology in interface tissue engineering. By utilizing electrospinning, researchers have been able to fabricate scaffolds with tailored properties to promote the regeneration and integration of tendon and bone tissues. The review discusses the unique structure and function of the tendon–bone interface, the mechanisms involved in its healing, and the limitations currently faced in achieving successful regeneration. Additionally, it highlights the potential of electrospinning technology in scaffold fabrication and its role in facilitating the development of functional and integrated tendon–bone interface tissues. Overall, this review provides valuable insights into the application of electrospinning technology for tendon–bone interface tissue engineering, emphasizing its significance in addressing the challenges associated with regeneration in this complex interface.
Introduction
The musculoskeletal system of the human body functions through the coordinated actions of various tissues, providing support and stability while allowing organized movement of muscles and bones. Ligaments attach bones to bones and tendons connect muscles to bones, making connective tissue an important part of our bodies. The transitional portion from muscle to bone, known as the tendon–bone interface (TBI), is a highly specialized site that can effectively transmit tensile loads from soft to hard tissues.1 These interfaces demonstrate gradient changes in structural, compositional and mechanical properties to efficiently transmit stresses between tendons and bones.2,3 Tears of tendon or ligament insertions are common clinical problems encountered in orthopedic practice. Injuries to the rotator cuff are among the most common sports injuries, usually causing pain, weakness, and limited range of motion in the shoulder, ultimately imposing a heavy financial burden on families and society.4 Rotator cuff tears restrict shoulder joint movement and severely impact patients' daily lives. Surgical repair is usually needed for rotator cuff tears. While some patients see significant improvements in shoulder function post-surgery, rerupture rates are still high, ranging from 15–94%.5,6 The high rerupture rate can be attributed to the fact that the injured site is typically located at the tendon–bone interface, a complex structure and composition that makes repair difficult, while scarring causes weak tissues.7,8 Faced with the current situation, rotator cuff surgery remains a challenge and better solutions need to be developed to avoid postoperative reruptures.
Between tendons and bones, there are four layers of structural and compositional transition: tendon, non-mineralized fibrocartilage, mineralized fibrocartilage, and bone9 (Fig. 1). Within the tendon–bone interface, cells and extracellular matrix are arranged in a gradient direction along the interface, with this transitional zone mediating load transfer from tendon to bone and helping transmit forces from the relatively soft tendon tissue to the rigid bone, so as to minimize stress concentrations. However, in the healing process from tendon to bone, this unique transitional tissue between tendon and bone is not reconstructed.5 There may be issues with fibrocartilage regeneration, bone loss, and immune dysregulation due to an imbalance between pro-inflammatory and anti-inflammatory macrophages after injury at the bone–tendon interface.11,12 During the early stages of rotator cuff tears (RCT), pro-inflammatory macrophages disproportionately produce interleukin 1 (IL-1), interleukin 6 (IL-6), and tumor necrosis factor (TNF-α),13 excessive secretion of inflammatory factors inhibits fibrocartilage layer regeneration and enhances osteoclast activity.12 As a result, M2 macrophages do not have sufficient numbers to support bone and fibrocartilage regeneration during tendon–bone healing.12 Therefore, it is feasible to improve the abnormal inflammatory response, promote cartilage regeneration, reduce bone loss and promote osteogenic differentiation in the process of tendon–bone healing.14,15
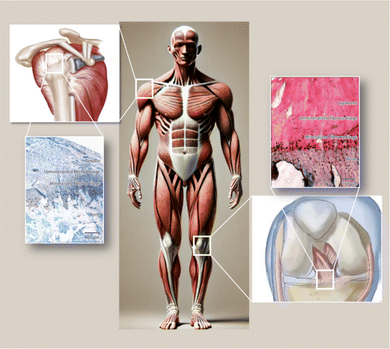 |
| Fig. 1 Common anatomical locations of fibrocartilage insertions in the human body and a schematic diagram illustrating the transitional zones within the insertion site.10 | |
In recent years, tissue engineering of the tendon–bone interface has gained increasing attention as a potential therapeutic option. For tissue repair, tissue engineering is important because it can mimic natural tissues and provide an extracellular matrix (ECM) environment that mimics natural tissues. ECM microenvironment regulates stem cell behaviors and fates in important ways. With electrospinning, polymer fibers can be produced with diameters of 50–1000 nm, which are several orders of magnitude smaller than fibers produced with other conventional fabrication methods.16 It has therefore been proposed that electrospinning can produce scaffolds composed of fibers that are more similar to natural collagen fibers in tendons in terms of their diameter scale and layered structures. There have been numerous studies showing that electrospun nanofiber scaffolds promote cellular adhesion, growth, proliferation and even differentiation in tendon tissue engineering applications, as well as demonstrating promising regenerative outcomes.17
This review focuses on the tendon–bone interface tissue. First, the current development characteristics of the attachment points of bone tendon and the healing mechanism of the injured interface are discussed. In the following sections, electrospinning technology will be primarily discussed in the context of tendon and bone interface tissue engineering. The second part introduces the design strategy of biomimetic stents prepared by loading active substances, last but not least, it summarizes the field's potential challenges, as well as future directions.
The structural and development of the tendon–bone interface
Tendon–bone transitions in vivo occur in two forms – fibrous attachments and fibrocartilaginous attachments.18 The characteristic of fibrous attachments is that the tendon–bone interface has dense fibrous connective tissue, found in tendons and ligaments attaching to the shaft or end of long bones (such as the medial collateral ligament, triceps tendon).19,20 Fibrous attachments can be further divided into two types: entheses and osteotendinous junctions. In the former, the tendon is indirectly attached to the bone by the periosteum, while in the latter, it is directly attached to the bone by the tendon.19 In comparison, fibrocartilaginous attachments occurring at bone protuberances and epicondyles (including the rotator cuff and anterior cruciate ligament) (Fig. 1) are more commonly encountered in human injuries.19,20 This paper will discuss fibrocartilaginous attachments. Fibrocartilage at bone attachments can be divided into four transitional zones (Fig. 2). A transition occurs between soft tissues and hard tissues at these four areas, which contain different collagen, minerals, cells, and other substances. The aim of this transition is to transmit external loads between soft tissues and hard tissues, ensuring stresses are minimized as much as possible and promoting joint movement. Zone I: fibrious connective tissue zone with elongated fibroblasts and mainly type I collagen, dispersed in a polysaccharide and glycoprotein matrix, with little type III collagen and elastin.10,22–24 Zone II: unmineralized fibrocartilage zone, avascular and mainly composed of type II and III collagen forming a mesh-like structure. This region also contains small amounts of type I collagen, aggrecan proteoglycans, cartilage-specific chondroitin sulfate glycosaminoglycans (GAGs).9,20–22,25–27 It delineates zones II and III mechanically by following unmineralized fibrocartilage and is called the tidemark.22 Zone III: mineralized fibrocartilage zone, avascular and principally comprised of type II collagen and enlarged fibrocartilage cells surrounded by proteoglycans, type I and X collagens. This region is highly irregular and represents the true junction between tendon and bone, interfacing with subchondral bone.21,22,27 Zone IV: an osteoclastic, osteocyte, and osteoblastic zone of bone tissue that contains mineralized type I collagen.9,22,27,28 While these four tissue regions differ compositionally, structurally they are continuous. There is a gradual increase in mineral content and decrease in collagenous fiber organization during the transition from tendon into bone. The fibers are also aligned and parallel at the tendon origin but become more curved, crossed and disorganized nearer the bone.9 This architecture provides a more rational stress distribution, enhances adherence strength and reduces risk of rupture or tearing24 (Table 1).
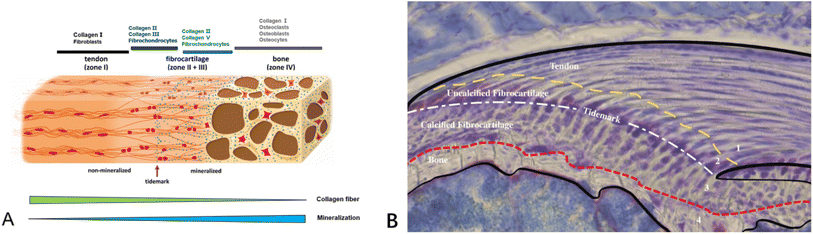 |
| Fig. 2 (A) Schematic diagram illustrating the structural and compositional changes within the transitional zones at the tendon–bone insertion site.21 (B) Schematic diagram of an histopathological section of the supraspinatus tendon–bone insertion in mice. The Alcian blue staining of proteoglycans in tendon, fibrocartilage, and mineralized fibrocartilage shows the presence of proteoglycans, highlighting the compositional gradient characteristic of the insertion site.10 | |
Table 1 Zone of fibrocartilaginous enheses
|
Zone 1 (dense fibrous connective tissue) |
Zone 2 (uncalcified fibrocartilage) |
Tidemark |
Zone 3 (calcified fibrocartilage) |
Zone 4 (bone) |
Composition |
Fibroblasts |
Fibrochondrocytes |
|
Fibrochondrocytes |
Osteocytes |
Type I collagen |
Proteoglycan aggrecan |
|
Type II collagen |
Osteoblasts |
Type III collagen |
Collagen (types I–III) |
|
Type I collagen |
Osteoclasts |
|
|
|
Type X collagen |
Type I collagen |
Significance |
Linearly arranged collagens whose mechanical properties are similar to that of the mid-substance tendon |
Dissipates bending of collagen fibers in tendon |
The basophilic demarcation between uncalcified and calcified fibrocartilage, representing the boundary between soft and hard tissues |
Irregularity of attachments into bone give mechanical integrity of enthesis |
Provides an attachment site for tendons |
While new tendons and bones emerge almost simultaneously during fetal development, formation of the transitional tissue between them occurs postnatally.29–34 In the initial stages of development, cartilage is mineralized to form bone through endochondral ossification, followed by fibrocartilage transition at the interface. During embryonic skeletal development, progenitor cells in the primary cartilage template promote bone protuberances to serve as tendon anchor points.35–37 During tendon formation and differentiation, Scleraxis (Scx) is an important transcription factor. The Scleraxis gene is found in progenitor cells as well as in cells of all tendinous tissues.38–40 Experiments show Scx knockout leads to severe developmental abnormalities of insertions.35 It plays an important role in chondrogenesis through transcription, expression in proliferating chondrocytes, and differentiation of chondrocytes.41,42 SOX9 remains active throughout early and late stages of chondrogenesis. With advancing development and differentiation, expressions of SOX9 and SCX gradually decrease regulated by various molecular drivers, including the transforming growth factor β (TGF-β) subfamily as a major factor since TGF-β signaling controls progenitor cell behavior and is crucial for tendon and cartilage formation.43 TGF-β also modulates SCX expression, with lack of SCX leading to abnormal tendon and ligament development.44 In addition to TGF-β, bone morphogenetic protein (BMP) is another influential factor. BMP-4 colocalizes with SCX in progenitor cells of bone protuberances and is regulated by SCX, inducing protuberances formation in the tendon–cartilage attachment region. The SCX/BMP-4 signaling transduction is necessary for these progenitor cells to differentiate into cartilage without the SCX/BMP-4 signaling transduction.29,30 It is thought that Indian hedgehog (Ihh) and parathyroid hormone-related protein (PTHrP) promote chondrocyte proliferation and differentiation, which act as a negative feedback loop in order to maintain the number and quality of chondrocytes.21 Research elucidated extracellular matrix expression during development with fibroblasts characteristically expressing type I collagen, collagen type II chondrocytes and collagen type X chondrocytes.30,31
Current understanding of tendon–bone interface healing
Current animal studies have shown that, unlike the organized and distinct development of four regions with cartilage attachment, in the case of tendon healing, scar tissue forms over the natural site of insertion, rather than reconstructing the site of insertion during embryonic development.20,22,45–49 The development of this fibrovascular scar tissue occurs in three stages: inflammation (0–7 days), repair (5–14 days), and remodeling (>14 days).50,51 The inflammation stage begins with platelet deposition of fibrin and fibronectin, leading to macrophage response and accumulation of insulin-like growth factor 1 (IGF-1), platelet-derived growth factor (PDGF), and transforming growth factor β (TGF-β).22 Getting tendon attachments healed requires TGF-1 and TGF-3, which are responsible for growth and differentiation of skeletal muscle.52,53 A significant amount of TGF-1 is responsible for cell migration and angiogenesis, while TGF-3 has been found to have an important role in the regeneration of articular cartilage for adults and in the healing of scarless wounds in newborns.54–56 When macrophages begin to secrete TGF-β1, the transition to the repair stage occurs, leading to fibroblast proliferation and scar tissue formation. Type III collagen is the main component of scar tissue.55 Although some degree of healing is achieved when tendon tissue is surgically fixed to bone, physiologically normal tissues have different mechanical properties from repaired tissues,57 even differing by orders of magnitude.57 Histologically, there are significant differences between the healed tissue and the physiological state, with loss of continuity in collagen fibers and no apparent gradient in mineral content, and the tissue at the healing interface consists of disorganized scar tissue.58 Damage to the interface between soft tissue and bone is accompanied by bone loss,58,59 making the repair of soft tissue to bone more complex. The bone mineral density of rat rotator cuffs was significantly reduced after tendon injury and repair.60 Similar results were found in a study on canine distal phalanges, with decreased bone mineral density suggesting that bone resorption may be a contributing factor to the poor outcomes at the repair site.59
In the development of tendons and bones, mechanical loading plays a significant role.61 Although the role of mechanobiology in the healing process is not yet clear, all cell types found near the attachment site have shown mechanical responsiveness.62 Current research suggests that cells are able to convert mechanical signals into gene regulation, which not only affects cell migration but also proliferation and differentiation.63 Additionally, the differences in the quantity of fibrocartilage found in different attachment sites may be related to mechanical forces.64,65 Muscle loading is essential for the growth and maturation of the attachment site, as evidenced by the reduction in mineral deposition and fibrocartilage formation observed when muscle loading is reduced using botulinum toxin, which affects postnatal attachment site maturation,66 resulting in unorganized fiber distribution and poorer mechanical properties.67 Several animal models have yielded similar results, with low-level loading (e.g., plaster fixation) being optimal for healing.57,68,69 A rotator cuff injury animal model showed that plaster fixation was more effective than exercise at promoting tendon healing.57,68 Similar results were found in a study comparing the effects of immediate and delayed mechanical loading on tendon–bone healing in an ACL model. Delayed loading led to greater healing than immediate or prolonged loading.57,69 In addition to mechanical stimuli, other physical factors such as electrical stimulation, ultraviolet radiation, and sound waves (e.g., ultrasound) have been studied. Through the stimulation of biological cascades, the increase of growth factors and cytokines levels, and the regulation of gene expression, they promote cell proliferation, differentiation, and osteogenesis. For example, low-intensity pulsed ultrasound (LIPUS) has been shown to increase levels of vascular endothelial growth factor (VEGF), thereby significantly improving vascular distribution at the attachment site.70,71
Cell phenotype and intercellular communication are another important influencing factor in the regeneration of the attachment site. During the insertion of tendon into bone, tendons, fibrocartilages, mineralized fibrocartilages, and bone are present. Each tissue type exhibits its own cell phenotypes and matrix composition. Interactions between the three resident cell populations can be crucial for fibroblast, fibrochondrocyte, and osteoblast regeneration at the interface. Osteoblast–fibroblast interactions mediated by heterotypic cell interactions can contribute to attachment site regeneration, resulting in osteoblastic and/or fibroblastic transdifferentiation. The interactions may also lead to the differentiation of stem cells into fibrocartilage cells, thereby regenerating the interface between the cartilage and the fibrocartilage.72 Numerous in vitro studies have also demonstrated the importance of heterotypic cell interactions in enhancing regeneration at interfaces.73–75 In vitro studies were conducted using co-cultures and tri-cultures using interface-related cell populations to examine the influence of cell communication on the development of fibrocartilage-specific markers.76–78 It is still unclear how intercellular communication works and what modulatory soluble factors are secreted in the healing environment, but intercellular communication encourages interface regeneration in both homing and healing environments.
Strategies for electrospinning to reconstruct special structures
Due to their high surface-to-volume ratios and interconnected porosities, fiberous materials have attracted a lot of attention from tissue engineering researchers, and diverse characteristics. Currently, there are several methods available for synthesizing nanofiber networks, including phase separation, template synthesis, and self-assembly.79,80 However, these methods have drawbacks such as long preparation time, limited fiber length, and discontinuous fiber scaffold structure.81 Therefore, electrospinning technology stands out among many nanofiber fabrication methods due to its obvious advantages, including the ability to produce continuous fiber networks relatively quickly and the versatility to work with various materials. Using a strong electric field, electrospinning produces micro- and nanofibers by jetting spinning from polymer melts or solutions. It is a novel processing method for producing nanoscale ultrafine fibers and can rapidly and cost-effectively manufacture nonwoven fiber structures.82,83 This fiber formation technique overcomes surface tension of the solution by applying voltage, promoting the formation of Taylor cones, jetting streams that pass through the air, and stretching out fibers with diameters ranging from nanometers to micrometers. These fibers deposit on collectors with opposite charges or grounded.84 Currently, most tissue engineering scaffolds produced are relatively homogeneous and possess isotropic mechanical properties. One end of a tendon–bone repair scaffold should have uniaxially aligned nanofibers, while the other end should have randomly oriented nanofibers.16,17 Additionally, it should mimic the mineralization changes at the tendon–bone interface, with a structure that gradually increases in hardness from soft to hard. It has been reported that electrospun polymer scaffolds with aligned fibers can enhance stem cell differentiation, alignment, and collagen fiber formation,85–90 while randomly oriented fibers play a role in osteogenesis and chondrogenesis.91 Although scaffolds with compositional, structural, and mechanical property gradients may have the potential to promote tendon–bone interface tissue growth, it is challenging to manufacture structures that transition from aligned fibers to randomly oriented fibers. Therefore, new manufacturing strategies are needed to prepare biomimetic scaffolds for interface tissue engineering (Fig. 3).
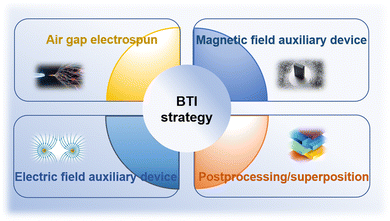 |
| Fig. 3 Schematic representation of manufacturing strategies for biomimetic scaffolds in interface tissue engineering. | |
Over the past two decades, electrospinning has been positively explored for fabricating nanofibers, enabling controllable preparation of nanofiber scaffolds with tunable composition, structure, alignment and functionality by modifying material choice, collector design, number of spinnerets, and electrospinning parameters. Typically, aligned nanofibers are produced using a high-speed rotating drum or framed collectors with gap spacing between edges. Researchers have modulated collector design to control electrospun nanofiber structure, rendering them more suitable for repair of highly oriented tissues by achieving directional nanofiber alignment mimicking native microenvironment topography. For example, customized collector configurations such as patterned drums or arrays of conductive nanowires have enabled fabrication of anisotropically aligned nanofiber yarns or woven textiles for tendon/ligament regeneration. Overall, electrospinning offers a versatile nanofibrous scaffold generation platform with tunable structural and biochemical cues to interface tendon/ligament repair. Xie et al. developed a collector consisting of two metal frames shaped like staplers, where nanoparticles were deposited in an aligned manner on the metal and a random manner in the gaps,92 fabricating an “aligned-to-random” electrospun nanofiber scaffold. Using this scaffold, the tendon–bone insertion site could be modeled as collagen fibers are structured. Studies showed this scaffold exhibited significantly increased modulus and ultimate tensile strength. When cells were cultured on this scaffold, tendon fibroblasts in the aligned and random portions respectively demonstrated highly oriented and non-oriented morphologies, and tendon fibroblasts implanted in the random and aligned portions of the scaffold produced type I collagen rather than type II collagen.92 This suggests fibroblasts cultured in this system could generate an appropriate ECM for tendon repair. Fig. 4A and B demonstrates the experimental setup for fabricating a typical “ordered and disordered” electrospun nanofiber scaffold. Kishan et al. implemented uniform fibrous mat collection via custom collectors equipped with synchronized rotation and utilized periodic copper wires to guide directional fiber alignment (Fig. 4C). Biodegradable polyurethane (BPUR) at varying contents were electrospun to fabricate composition gradient mesh scaffolds with and without fiber orientation for tendon–bone grafting.93 The polymer gradient from BPUR50 to BPUR10 along the aligned direction allowed progressive transition in mechanical properties.93 In addition to allowing continuous gradient in polymer content, the technique can be used to generate activity gradients along the arrangement direction by adding additives into the polymer solution. Neither the compositional gradients nor fiber alignment of the scaffolds affected cell attachment, and meshes with different alignments exhibited significant stiffness gradients. Both studies employed gap electrospinning for scaffold preparation, requiring almost no additional equipment beyond standard electrospinning apparatus, representing an economical and convenient approach achieving unique microstructural control. However, the method is limited in fabricating scaffolds of large sizes and thicknesses.96,97 In this configuration, the ordered and disordered nanofiber regions mimic the graded tendon-to-bone transition. Such modulated nanofiber topography could guide cell behaviors relevant to regenerating the zonal interfaces.
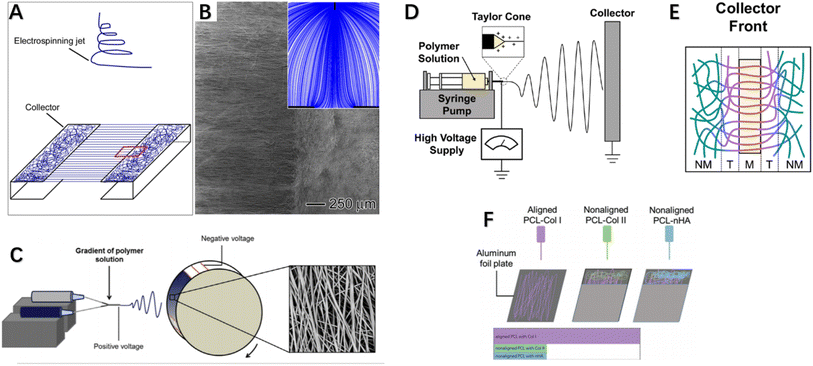 |
| Fig. 4 (A) Stapler-like device for manufacturing nanofiber scaffolds and (B) SEM images of randomly arranged and uniaxial nanofiber scaffolds were obtained. Inset: schematic illustration of electric field lines between needle and collector. (C) Electrospinning device with custom rotating collector for fabricating component gradients and SEM images of fibers. (D) Electrospinning apparatus side view, and (E) collector equipped with a single magnet. (F) Schematic illustration of multi-layered electrospinning fiber stacking to achieve alignment-to-random orientation effects.92–95 | |
Researchers from Tindell et al. demonstrated that magnetically assisted electrospinning allows for fine spatial control over fiber alignments, resulting in wavy interfaces between aligned and random fiber regions.94 Ajao et al. employed magnetically assisted electrospinning technique using additional cylindrical magnets where well-oriented nanofibers were observed at magnet tops while non-aligned at other positions98 (Fig. 4D and E). By adjusting magnet configurations, magnetically assisted electrospinning can achieve various fiber gradients including random, multi-directional and other complex gradients. This method only requires minor modifications to conventional electrospinning setup via addition of an array of magnets of different types and configurations to spatially control fiber alignments and generate desired fiber architectures. An advantage of this general device is its ability to be configured in a modular manner without changing the original setup. Researchers also employed auxiliary electrodes to influence electrospinning jet trajectory and thereby control deposition regions and direction of electrospun fibers.99,100 Magnetically and electrically assisted fields provide technological basis for fabrication of structurally graded scaffolds needed for tendon–bone interface regeneration. In summary, these studies demonstrated modulated fiber alignments and compositional gradients mimicking tendon–bone transition using simple yet effective electrospinning modifications, holding promise for interface regeneration applications. The techniques of magnetically or electrically assisted electrospinning allow spatial control over fiber deposition enabling generation of fibers with gradients to interface repair. In addition to the aforementioned enhancements made directly on electrospinning equipment, post-processing methods on electrospun fibers can also fabricate scaffolds meeting interface tissue engineering requirements. Manufacturing post-treatment methods can improve orientation by converting poorly aligned fibers into highly oriented meshes via combined application of tensile stretching and thermal annealing.101,102 Zong et al. first described a post-drawing method, investigating the microstructure, morphology and texture of electrospun poly(lactic-co-glycolic acid) non-woven membranes after stretching and heat treatment, as well as their degradation and mechanical properties. Results showed the stretched and annealed membranes had higher crystallinity and evident lamellar structuring with improved orientation. Material orientation and tensile strength both increased with rising draw ratios.103 Post-drawing provides a viable alternative to obtaining highly aligned fibers or increasing orientation from randomly oriented meshes, with significantly enhanced mechanical properties. However, drawbacks exist such as ∼20% reduction in microstructural porosity post-stretching.103 Unlike post-drawing, Yu et al. employed photothermal welding technique on aligned fiber scaffolds to successfully fabricate gradients from alignment to randomness.104 They premixed ICG directly into the PU solution for electrospinning using a drum-type collector to generate uniaxially aligned nanofibers. Post fabrication, laser irradiation activated the photothermal material (ICG) to generate heat, melting the nanofibers at their melting points and welding them. The photothermally-induced structural changes in nanofiber scaffolds satisfied structural gradation, with subsequent graded mineralization mimicking biological gradients. In vitro studies showed the scaffold was biocompatible and guided tendon stem cell morphological elongation and tenogenic and osteogenic differentiation, with in vivo investigations via immunohistochemical and biomechanical analyses confirming improved rabbit supraspinatus injury healing. To achieve aligned-to-random orientation in electrospun nanofiber membranes, researchers have also employed stacking of multiple electrospun fiber layers to induce orientation transition effects,95 as depicted in Fig. 4F where Cong et al. separately fabricated membranes including aligned nanofibers PCL (aPCL), random PCL (nPCL), aPCL-collagen I, nPCL-collagen II and nPCL-nanohydroxyapatite (nHA) fibers. They layer-electrospun nPCL-collagen II and nPCL-nHA onto one end of aPCL-collagen I in a stepwise manner. By interlayering Col I, Col II and nHA onto PCL scaffolds, they simulated bone grafts and conducted prosthetic reconstruction. Results showed scaffolds with good biocompatibility, with significantly more neo-fibrocartilage formation observed at experimental group bone–graft junctions versus controls, indicating improved bone ingrowth, larger fibrocartilage formation and better biomechanical properties. In addition to the above-mentioned methods of fabricating nanofiber scaffolds with aligned-random orientation gradients via various improved apparatus, some researchers have incorporated various active components into conventionally aligned orientation or random orientation fiber membranes to aim at promoting tendon–bone healing. We will discuss these related studies in more detail in the next section (Table 2).
Table 2 Summary of methods for fabricating aligned-to-random scaffold
Author |
Strategy |
Ref. |
Xie et al. |
Combination of gapped electrospinning and conventional electrospinning using a collector composed of two binder clip-shaped metal frames |
92 |
Kishan et al. |
Fiber alignment achieved by periodic copper wires combined with synchronized rotation of a mesh collector |
93 |
Ajao et al. |
Addition of auxiliary magnetic field device |
98 |
Tindell et al. |
Addition of auxiliary magnetic field device |
94 |
Teo et al. |
Addition of auxiliary electrode device |
99 |
Leon M. et al. |
Addition of auxiliary electrode device |
100 |
Zong et al. |
Stretching and thermal treatment |
103 |
Yu et al. |
Incorporation of photothermal agent into fiber membrane, utilizing photothermal reaction |
104 |
Cong et al. |
Layering multiple electrospun fiber membranes |
95 |
Strategies for preparing polymers by electrospinning
The selection of biomaterials plays a crucial role in determining the key parameters of tissue engineering scaffolds, such as structure, biochemical properties, and mechanical performance. However, it is still unclear which biomaterial is the optimal choice for generating such scaffolds. It has been proven that bone-to-tendon tissue engineering scaffolds can be constructed from a variety of biomaterials. Commonly used biodegradable synthetic materials for tissue engineering include poly-L-lactic acid (PLLA),105 polyglycolic acid (PGA),106 poly(lactic-co-glycolic acid) (PLGA),107,108 and polycaprolactone (PCL).109–111 Because PLGA was approved by the U.S. Food and Drug Administration (FDA), it has gained widespread attention as a scaffold for tissue engineering and drug delivery.109 Common natural polymers include alginate,112 silk,107,113,114 and collagen (Fig. 5).114–116
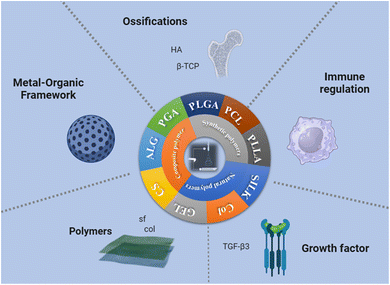 |
| Fig. 5 Summary diagram of interface tissue engineering scaffold materials prepared by electrospinning. | |
Currently, the primary focus has been on copolymers, blends or polymer-ceramic/bioactive glass composites, aimed at fully leveraging the properties of different materials. Composite strategies attract significant interest in interface tissue engineering as incorporating different materials can better mimic the graded structures of native attachments and exert distinct biological effects. Liu et al. employed electrospinning to generate scaffolds with gradient concentration of PLGA and PCL, demonstrating the ability to recreate the tendon–bone microenvironment and reconstruct the interface.108 Evaluation of the scaffolds' induction of adipose-derived mesenchymal stem cells (ASCs) osteogenic differentiation showed the gradient in mineral content on the nanofiber surfaces could guide graded ASC differentiation into osteoblasts for rotator cuff repair.108 Naghashzargar et al. fabricated a novel scaffold material using silk fibroin as the core encapsulated by P3HB and PCL fibers via electrospinning. Results indicated this material possessed enhanced tensile strength meeting the mechanical demands for tendon–bone reconstruction, and demonstrated good biocompatibility in L929 cell studies.117
Bone loss frequently accompanies tendon–bone injuries, and incorporating bone bioactive materials is a common polymer strategy to enhance electrospun scaffold osteogenic activity. These include calcium phosphate ceramics (particularly hydroxyapatite (HA)118,119 and β-tricalcium phosphate (β-TCP)105,120), bioactive glasses (mainly silicate-based 45S5 Bioglass®121,122). Lv et al. incorporated hydroxyapatite (HA) into poly(lactic acid) (PLLA) to fabricate electrospun nanofiber membranes as rotator cuff repair patches. The results showed hydrophilicity of electrospun fibers improved with good cytocompatibility, enhancing alkaline phosphatase (ALP) expression of rat bone marrow mesenchymal stem cells (BMSCs), indicating better induction of rat BMSC osteogenesis.123 Soo Kim et al. generated a tendon-to-bone structural scaffold consisting of 4 layers – collagen forms the tendon layer, fibrocartilage bonds to chondroitin sulfate to form fibrocartilage, HA forms the mineralized fibrocartilage, and calcium forms the bone layer. Young's modulus increased while elongation decreased.124 Further analysis showed that tenocytes, chondrocytes, and osteoblasts throughout the tendon and fibrocartilage layers, as well as osteoblasts in the bone layer, showed enhanced proliferation.124 Erisken et al.125 fabricated β-TCP graded electrospun PCL nanofiber membranes and analyzed their mechanical properties. During tensile deformation, Young's modulus increased gradually along the scaffold with increasing mineral content, whereas elongation decreased.125,126 Furthermore, MC3T3 pre-osteoblastic cell attachment and migration showed different behaviors and morphologies along the scaffold.125,126 According to these studies, structural and chemical gradients affect mechanical properties and cellular behavior of scaffolds, especially mineral gradients.
Biological factors like TGF-β3 may also be important promotors in the rotator cuff tear healing process. Reifenrath et al. studied a TGF-β3 loaded electrospun shellac-grafted-polycaprolactone (CS-g-PCL) fiber scaffold and compared its biomechanical and histological effects on tendon healing with unloaded fiber scaffolds in a rat chronic tendon defect model. The results showed the fiber scaffold with better degradability and biocompatibility, while fibrosis appeared reduced as a foreign body encapsulation and scar formation indicator.127 Gao et al. synthesized strontium-doped mesoporous bioactive glass nanoparticles (Sr-MBG) via a sol–gel method and prepared a biphasic inductive and immunomodulatory electrospun fibrous scaffold containing Sr-MBG (BIIEFS). Mesenchymal stem cells (MSCs) were shown to differentiate osteogenically and chondrogenically when treated with BIIEFS, with multiple bioactive ions being released, and macrophages were confirmed to acquire an M2 phenotype when stimulated with BIIEFS. Experimental results showed that electrospun scaffolds increased the number of M2 macrophages, while synchronous regeneration of tendon, fibrocartilage, and bone was observed, which significantly enhanced supraspinatus tendon-humerus biomechanical strength.128
These studies exemplify how incorporating bioactive factors and materials into electrospun scaffolds holds promise for developing clinically translatable therapeutics through regulating the wound microenvironment and cellular responses during interface tissue regeneration. Continued optimizations modulating scaffold composition, topography and biomechanical integrity are important next steps towards achieving functional restoration. Metal ions play important roles in tissue repair and have wide clinical applications. However, their use is limited due to narrow metabolic and healing windows. In this study, Yang et al. produced biphasic metal-flexible electrospun fiber membranes from a MOF carrier through continuous electrospinning and matching the longitudinal spatial morphology of multiple tissues simultaneously.129 As a carrier, MOF not only enables sustained metal ion release but also promotes osteogenesis and tenogenesis on the scaffold. Studies showed this layered electrospun fibrous structure could accelerate tenogenesis, biological mineralization and vascularization. During in vivo validation, the agent was shown to promote tendon and bone tissue repair, as well as fibrocartilage reconstruction, facilitating synchronized regeneration of several tissues at the injured tendon–bone interface. In summary, Using MOFs as the base, electrospun fiber membranes will be biphasic metal-flexible represent a novel class of biodegradable soft scaffolds with tremendous potential for reconstructing tissue defects, especially graded tissue injuries.129 These studies exemplify how incorporating bioactive factors and materials into electrospun scaffolds holds promise for developing clinically translatable therapeutics through regulating the wound microenvironment and cellular responses during interface tissue regeneration. Continued optimizations modulating scaffold composition, topography and biomechanical integrity are important next steps towards achieving functional restoration. There is also a kind of acellular matrix materials used as interface tissue engineering to promote tendon and bone healing, but this review mainly focuses on the materials and applications of electrospinning technology, so it will not be discussed (Table 3).
Table 3 Summary of electrospun polymer strategies for tendon–bone interface tissue engineering
Author |
Polymer strategy |
Function |
Ref. |
Lv et al. |
PLLA-HA |
Induced osteogenic differentiation of BMSCs |
123 |
Liu et al. |
PLGA-PCL-HA |
Induced osteogenic differentiation of ASCs |
108 |
Naghashzargar et al. |
P3HB-PCL-SF |
Met mechanical requirements for tendon–bone reconstruction |
117 |
Soo Kim et al. |
Collagen-HA |
Increased Young's modulus, promoted proliferation of fibroblasts, chondrocytes, and osteoblasts |
124 |
Erisken et al. |
PCL-β-TCP |
Increased Young's modulus, promoted MC3T3 cell adhesion |
125 and 126 |
Reifenrath et al. |
CS-g-PCL-TGF-β3 |
Excellent degradation properties, reduced in vivo fibrosis |
127 |
Gao et al. |
BIEFS |
Promoted osteogenic and chondrogenic differentiation of MSCs, and polarization of macrophages towards M2 phenotype |
128 |
Yang et al. |
PLA-HKUST-1/PLA-ZIF-11 |
Enhanced osteogenesis and tendon formation |
129 |
Evaluation and analysis of mechanical properties of bionic scaffolds
Since the function of the BTI interface is to bear and transmit loads between mechanically different tissues, it must be able to withstand certain mechanical loads. Evidence also suggests that cells near the interface can sense mechanical forces, which, through genetic regulation, further influence cell migration, extracellular matrix adhesion, and cell proliferation and differentiation.21 Therefore, excellent mechanical strength is a necessary condition for the fabricated bionic scaffold. In this section, we will discuss the mechanical strength of some of the electrospun bionic scaffolds mentioned above.
In the study by Xie et al.,92 tensile tests were used to evaluate the mechanical properties of the scaffold. The scaffold was tested on a tensile testing machine to measure its tensile strength and elastic modulus. Aligned fibers: tensile strength reached approximately 15 MPa, and elastic modulus was 100 MPa. Random fibers: tensile strength was about 5 MPa, and elastic modulus was 50 MPa. The tensile strength of the scaffold depends on the arrangement of the fibers. The tensile strength of the aligned fibers was significantly higher than that of the random fibers. In the study by Kishan et al.,93 various testing methods were used to evaluate the mechanical properties of the scaffold, including tensile tests, compression tests, and bending tests. The tensile strength of the aligned fiber scaffold was 23.5 ± 1.2 MPa. The tensile strength of the random fiber scaffold was 12.8 ± 0.9 MPa. The elastic modulus of the aligned fiber scaffold was 162 ± 10 MPa. The elastic modulus of the random fiber scaffold was 92 ± 8 MPa. Elongation at break: the elongation at break of the aligned fiber scaffold was 21.7 ± 1.8%. The elongation at break of the random fiber scaffold was 15.3 ± 1.4%. Similarly, the elastic modulus of the aligned fibers was higher than that of the random fibers, indicating that aligned fibers provide stronger mechanical support and stability. Similarly, other studies have recorded tensile strengths ranging from 12 to 45 MPa and elastic moduli from 90 to 220 MPa. Specific data are summarized in Table 4. These studies demonstrate the importance of mechanical properties for tissue engineering scaffolds. In summary, these studies found that aligned fibers generally have superior tensile strength and elastic modulus compared to random fibers. However, considering the complex structural variations of the BTI interface, the quality of a bionic scaffold cannot be judged solely by its mechanical strength.
Table 4 Summary of mechanical properties of bionic scaffolds
Tensile strength (aligned) |
Tensile strength (random) |
Elastic modulus (aligned) |
Elastic modulus (random) |
Elongation at break (aligned) |
Elongation at break (random) |
Ref. |
15 |
5 |
100 |
50 |
— |
— |
92 |
23.5 ± 1.2 |
12.8 ± 0.9 |
162 ± 10 |
92 ± 8 |
21.7 ± 1.8 |
15.3 ± 1.4 |
93 |
35 |
— |
200 |
— |
— |
— |
96 |
45 |
— |
220 |
— |
— |
— |
97 |
30 |
20 |
150 |
100 |
— |
— |
94 |
12 |
— |
90 |
— |
— |
— |
98 |
28 |
— |
180 |
— |
— |
— |
99 |
18 |
— |
140 |
— |
— |
— |
100 |
22 |
— |
160 |
— |
— |
— |
101 |
25 |
— |
130 |
— |
— |
— |
102 |
20 |
— |
120 |
— |
— |
— |
103 |
32 |
— |
210 |
— |
— |
— |
104 |
35 |
— |
220 |
— |
— |
— |
95 |
Summary and future perspectives
As part of this article, the author summarizes how electrospinning technology can be used in the design of tendon–bone interface tissues. Various cell types maintain stability within the tendon–bone interface, which enables efficient transmission of muscular forces to the skeleton. The mechanism of tendon–bone interface healing is complex yet crucial for patient prognosis, and current treatments still fall short in achieving complete reconstruction of the tendon–bone interface. The author summarizes the research on the biological and mechanical mechanisms of tendon–bone interface healing and discusses the developmental characteristics and healing mechanisms of injured interfaces, which primarily involve molecular biology, physical factor stimulation, and mechanical stimulation. The article also delves into the strategies and materials involved in scaffold preparation for bone–tendon interface tissue engineering using electrospinning technology. Tissue engineering to achieve regeneration of muscle-tendon/ligament–bone interfaces presents an attractive strategy for providing functional transplants and improving clinical outcomes following injuries. In spite of this, attachment site tissue engineering represents a significant challenge for biologists and engineers because of the complex structure and the critical interdependences between structure, function, and mechanical properties. Tissue engineering has made rapid advances in recent years, but functional attachment site-like tissues have yet to be created. In order to improve the quality of attachment sites, a deeper understanding of their structure–function relationship, as well as mechanisms of attachment site development, homeostasis, and regeneration, is necessary. Biomechanical and biological factors drive the development of naturally occurring insertion sites, which are functionally graded tissues composed of multiple cells types and extracellular matrix components. Transcription factors specific to different types of cells initiate the development of graded interfaces between bone and muscle-tendon or ligament. Consequently, muscle strength signals determine the maturity and growth of the attachment site. As such, fundamental research on the molecular and mechanical factors regulating natural attachment site development and homeostasis is essential for advancing future interface tissue engineering approaches. The interface between soft tissues and bones plays an important role in musculoskeletal function, and its regeneration through tissue engineering promises to improve clinical results. However, there are still several challenges to overcome before the application of current research in clinical practice: (1) regarding the direction of mechanism research, although there have been numerous studies on the molecular mechanisms of tendon–bone interface healing, the core keys to healing remain unclear. (2) In terms of experimental translation, interdisciplinary collaboration is required to demonstrate the classification, composition, and implantation methods of various scaffold materials, aiming to obtain higher-level clinical research and find the most suitable treatment options. (3) Alongside the emergence of new technologies and materials, ethical concerns have also arisen. Complex tissue engineering implants and the introduction of exogenous cells pose risks to patients. Therefore, close attention to ethical issues is still necessary before implementing these treatment strategies.
Data availability
No data was used for the research described in the article.
Conflicts of interest
The authors declare that they have no known competing financial interests or personal relationships that could have appeared to influence the work reported in this paper.
Acknowledgements
This research was funded by the Science and Technology Department of National Natural Science Foundation of China (No. 82260426).
References
- K. Sharif, C. Bridgewood, S. Dubash and D. McGonagle, Intestinal and enthesis innate immunity in early axial spondyloarthropathy, Rheumatology, 2020, 59(l4), iv67–iv78 CrossRef CAS
. - M. Benjamin, E. J. Evans and L. Copp, The histology of tendon attachments to bone in man, J. Anat., 1986, 149, 89–100 CAS
. - K. L. Moffat, W. H. Sun, P. E. Pena, N. O. Chahine, S. B. Doty and G. A. Ateshian, et al., Characterization of the structure-function relationship at the ligament-to-bone interface, Proc. Natl. Acad. Sci. U. S. A., 2008, 105(23), 7947–7952 CrossRef CAS
. - R. Z. Tashjian, Epidemiology, natural history, and indications for treatment of rotator cuff tears, Clin. Sports Med., 2012, 31(4), 589–604 CrossRef
. - S. Thomopoulos, G. R. Williams and L. J. Soslowsky, Tendon to bone healing: differences in biomechanical, structural, and compositional properties due to a range of activity levels, J. Biomech. Eng., 2003, 125(1), 106–113 CrossRef CAS PubMed
. - B. R. Neri, K. W. Chan and Y. W. Kwon, Management of massive and irreparable rotator cuff tears, J. Shoulder Elbow Surg., 2009, 18(5), 808–818 CrossRef
. - E. Pennisi, Tending tender tendons, Science, 2002, 295(5557), 1011 CrossRef CAS
. - D. L. Butler, N. Juncosa and M. R. Dressler, Functional efficacy of tendon repair processes, Annu. Rev. Biomed. Eng., 2004, 6, 303–329 CrossRef CAS PubMed
. - H. M. Shaw and M. Benjamin, Structure–function relationships of entheses in relation to mechanical load and exercise, Scand. J. Med. Sci. Sports, 2007, 17(4), 303–315 CrossRef CAS
. - H. H. Lu and S. Thomopoulos, Functional attachment of soft tissues to bone: development, healing, and tissue engineering, Annu. Rev. Biomed. Eng., 2013, 15, 201–226 CrossRef CAS
. - Z. Chen, M. Jin, H. He, J. Dong, J. Li and J. Nie, et al., Mesenchymal stem cells and macrophages and their interactions in tendon–bone healing, J. Orthop. Transl., 2023, 39, 63–73 Search PubMed
. - H. Gao, L. Wang, H. Jin, Z. Lin, Z. Li and Y. Kang, et al., Regulating Macrophages through Immunomodulatory Biomaterials Is a Promising Strategy for Promoting Tendon–Bone Healing, J. Funct. Biomater., 2022, 13(4), 243 CrossRef CAS PubMed
. - J. Y. Sunwoo, C. D. Eliasberg, C. B. Carballo and S. A. Rodeo, The role of the macrophage in tendinopathy and tendon healing, J. Orthop. Res., 2020, 38(8), 1666–1675 CrossRef
. - J. Ye, C. Xie, C. Wang, J. Huang, Z. Yin and B. C. Heng, et al., Promoting musculoskeletal system soft tissue regeneration by biomaterial-mediated modulation of macrophage polarization, Bioact. Mater., 2021, 6(11), 4096–4109 CAS
. - Z. Julier, A. J. Park, P. S. Briquez and M. M. Martino, Promoting tissue regeneration by modulating the immune system, Acta Biomater., 2017, 53, 13–28 CrossRef CAS PubMed
. - G. Jin, R. He, B. Sha, W. Li, H. Qing and R. Teng, et al., Electrospun three-dimensional aligned nanofibrous scaffolds for tissue engineering, Mater. Sci. Eng., C, 2018, 92, 995–1005 CrossRef CAS
. - M. B. Guner, A. D. Dalgic, A. Tezcaner, S. Yilanci and D. Keskin, A dual-phase scaffold produced by rotary jet spinning and electrospinning for tendon tissue engineering, Biomed. Mater., 2020, 15(6), 065014 CrossRef CAS
. - J. Apostolakos, T. J. Durant, C. R. Dwyer, R. P. Russell, J. H. Weinreb and F. Alaee, et al., The enthesis: a review of the tendon-to-bone insertion, Muscles Ligaments Tendons J., 2014, 4(3), 333–342 Search PubMed
. - J. Padulo, F. Oliva, A. Frizziero and N. Maffulli, Muscles, Ligaments and Tendons Journal - Basic principles and recommendations in clinical and field Science Research: 2016 Update, Muscles Ligaments Tendons J., 2016, 6(1), 1–5 Search PubMed
. - M. Benjamin, T. Kumai, S. Milz, B. M. Boszczyk, A. A. Boszczyk and J. R. Ralphs, The skeletal attachment of tendons--tendon “entheses”, Comp. Biochem. Physiol., Part A: Mol. Integr. Physiol., 2002, 133(4), 931–945 CrossRef CAS
. - T. S. Font, E. R. Balmayor and M. Van Griensven, Strategies to engineer tendon/ligament-to-bone interface: Biomaterials, cells and growth factors, Adv. Drug Delivery Rev., 2015, 94, 126–140 CrossRef
. - M. E. Angeline and S. A. Rodeo, Biologics in the management of rotator cuff surgery, Clin. Sports Med., 2012, 31(4), 645–663 CrossRef PubMed
. - M. Laiho, M. B. Weis and J. Massagué, Concomitant loss of transforming growth factor (TGF)-beta receptor types I and II in TGF-beta-resistant cell mutants implicates both receptor types in signal transduction, J. Biol. Chem., 1990, 265(30), 18518–18524 CrossRef CAS
. - J. L. Wrana, L. Attisano, R. Wieser, F. Ventura and J. Massagué, Mechanism of activation of the TGF-beta receptor, Nature, 1994, 370(6488), 341–347 CrossRef CAS
. - N. Friese, M. B. Gierschner, P. Schadzek, Y. Roger and A. Hoffmann, Regeneration of Damaged Tendon–Bone Junctions (Entheses)-TAK1 as a Potential Node Factor, Int. J. Mol. Sci., 2020, 21(15), 5177 CrossRef CAS
. - A. Scott, J. L. Cook, D. A. Hart, D. C. Walker, V. Duronio and K. M. Khan, Tenocyte responses to mechanical loading in vivo: a role for local insulin-like growth factor 1 signaling in early tendinosis in rats, Arthritis Rheum., 2007, 56(3), 871–881 CrossRef CAS PubMed
. - M. Benjamin and J. R. Ralphs, Entheses--the bony attachments of tendons and ligaments, Ital. J. Anat. Embryol., 2001, 106(2 Suppl 1), 151–157 CAS
. - L. Smith, Y. Xia, L. M. Galatz, G. M. Genin and S. Thomopoulos, Tissue-engineering strategies for the tendon/ligament-to-bone insertion, Connect. Tissue Res., 2012, 53(2), 95–105 CrossRef CAS
. - S. Thomopoulos, G. M. Genin and L. M. Galatz, The development and morphogenesis of the tendon-to-bone insertion – what development can teach us about healing, J. Musculoskeletal Neuronal Interact., 2010, 10(1), 35–45 CAS
. - S. Thomopoulos, G. R. Williams, J. A. Gimbel, M. Favata and L. J. Soslowsky, Variation of biomechanical, structural, and compositional properties along the tendon to bone insertion site, J. Orthop. Res., 2003, 21(3), 413–419 CrossRef
. - I. E. Wang, S. Mitroo, F. H. Chen, H. H. Lu and S. B. Doty, Age-dependent changes in matrix composition and organization at the ligament-to-bone insertion, J. Orthop. Res., 2006, 24(8), 1745–1755 CrossRef CAS PubMed
. - Y. S. Bland and D. E. Ashhurst, Fetal and postnatal development of the patella, patellar tendon and suprapatella in the rabbit; changes in the distribution of the fibrillar collagens, J. Anat., 1997, 190(Pt 3), 327–342 CrossRef CAS PubMed
. - Y. S. Bland and D. E. Ashhurst, The hip joint: the fibrillar collagens associated with development and ageing in the rabbit, J. Anat., 2001, 198(Pt 1), 17–27 CrossRef CAS PubMed
. - H. Fujioka, G. J. Wang, K. Mizuno, G. Balian and S. R. Hurwitz, Changes in the expression of type-X collagen in the fibrocartilage of rat Achilles tendon attachment during development, J. Orthop. Res., 1997, 15(5), 675–681 CrossRef CAS PubMed
. - M. L. Killian and S. Thomopoulos, Scleraxis is required for the development of a functional tendon enthesis, FASEB J., 2016, 30(1), 301–311 CrossRef CAS
. - Y. Sugimoto, A. Takimoto, H. Akiyama, R. Kist, G. Scherer and T. Nakamura, et al., Scx+/Sox9+ progenitors contribute to the establishment of the junction between cartilage and tendon/ligament, Development, 2013, 140(11), 2280–2288 CrossRef CAS
. - E. Blitz, A. Sharir, H. Akiyama and E. Zelzer, Tendon–bone attachment unit is formed modularly by a distinct pool of Scx- and Sox9-positive progenitors, Development, 2013, 140(13), 2680–2690 CrossRef CAS PubMed
. - P. Cserjesi, D. Brown, K. L. Ligon, G. E. Lyons, N. G. Copeland and D. J. Gilbert, et al., Scleraxis: a basic helix-loop-helix protein that prefigures skeletal formation during mouse embryogenesis, Development, 1995, 121(4), 1099–1110 CrossRef CAS PubMed
. - R. Schweitzer, J. H. Chyung, L. C. Murtaugh, A. E. Brent, V. Rosen and E. N. Olson, et al., Analysis of the tendon cell fate using Scleraxis, a specific marker for tendons and ligaments, Development, 2001, 128(19), 3855–3866 CrossRef CAS
. - A. E. Brent, R. Schweitzer and C. J. Tabin, A somitic compartment of tendon progenitors, Cell, 2003, 113(2), 235–248 CrossRef CAS PubMed
. - H. Akiyama, Control of chondrogenesis by the transcription factor Sox9, Mod. Rheumatol., 2008, 18(3), 213–219 CrossRef CAS
. - W. Huang, U. I. Chung, H. M. Kronenberg and B. de Crombrugghe, The chondrogenic transcription factor Sox9 is a target of signaling by the parathyroid hormone-related peptide in the growth plate of endochondral bones, Proc. Natl. Acad. Sci. U. S. A., 2001, 98(1), 160–165 CrossRef CAS
. - M. Benjamin, H. Toumi, J. R. Ralphs, G. Bydder, T. M. Best and S. Milz, Where tendons and ligaments meet bone: attachment sites ('entheses') in relation to exercise and/or mechanical load, J. Anat., 2006, 208(4), 471–490 CrossRef CAS
. - E. Blitz, S. Viukov, A. Sharir, Y. Shwartz, J. L. Galloway and B. A. Pryce, et al., Bone ridge patterning during musculoskeletal assembly is mediated through SCX regulation of Bmp4 at the tendon-skeleton junction, Dev. Cell, 2009, 17(6), 861–873 CrossRef PubMed
. - S. C. Juneja and C. Veillette, Defects in tendon, ligament, and enthesis in response to genetic alterations in key proteoglycans and glycoproteins: a review, Arthritis, 2013, 2013, 154812 CrossRef
. - S. A. Rodeo, S. P. Arnoczky, P. A. Torzilli, C. Hidaka and R. F. Warren, Tendon-healing in a bone tunnel. A biomechanical and histological study in the dog, J. Bone Jt. Surg., Am. Vol., 1993, 75(12), 1795–1803 CrossRef CAS
. - M. Aoki, H. Oguma, S. Fukushima, S. Ishii, S. Ohtani and G. Murakami, Fibrous connection to bone after immediate repair of the canine infraspinatus: the most effective bony surface for tendon attachment, J. Shoulder Elbow Surg., 2001, 10(2), 123–128 CrossRef CAS PubMed
. - S. A. Rodeo, S. P. Arnoczky, P. A. Torzilli, C. Hidaka and R. F. Warren, Tendon-healing in a bone tunnel. A biomechanical and histological study in the dog, J. Bone Jt. Surg., Am. Vol., 1993, 75(No.12), 1795–1803 CrossRef CAS
. - H. Fujioka, R. Thakur, G. J. Wang, K. Mizuno, G. Balian and S. R. Hurwitz, Comparison of surgically attached and non-attached repair of the rat achilles tendon–bone interface. Cellular organization and type X collagen expression, Connect. Tissue Res., 1998, 37(3–4), 205–218 CrossRef CAS
. - L. V. Gulotta and S. A. Rodeo, Growth factors for rotator cuff repair, Clin. Sports Med., 2009, 28(1), 13–23 CrossRef PubMed
. - J. E. Carpenter, S. Thomopoulos and L. J. Soslowsky, Animal models of tendon and ligament injuries for tissue engineering applications, Clin. Orthop. Relat. Res., 1999,(367), S296–S311 CrossRef CAS
. - R. Merino, Y. Gañan, D. Macias, A. N. Economides, K. T. Sampath and J. M. Hurle, Morphogenesis of digits in the avian limb is controlled by FGFs, TGFbetas, and noggin through BMP signaling, Dev. Biol., 1998, 200(1), 35–45 CrossRef PubMed
. - L. M. Galatz, L. J. Sandell, S. Y. Rothermich, R. Das, A. Mastny and N. Havlioglu, et al., Characteristics of the rat supraspinatus tendon during tendon-to-bone healing after acute injury, J. Orthop. Res., 2006, 24(3), 541–550 CrossRef CAS PubMed
. - C. Soo, S. R. Beanes, F. Y. Hu, X. Zhang, C. Dang and G. Chang, et al., Ontogenetic transition in fetal wound transforming growth factor-beta regulation correlates with collagen organization, Am. J. Pathol., 2003, 163(6), 2459–2476 CrossRef CAS
. - S. L. Edwards, T. S. Lynch, M. D. Saltzman, M. A. Terry and G. W. Nuber, Biologic and pharmacologic augmentation of rotator cuff repairs, J. Am. Acad. Orthop. Surg., 2011, 19(10), 583–589 CrossRef PubMed
. - E. H. Lee and J. H. Hui, The potential of stem cells in orthopaedic surgery, J. Bone Jt. Surg., Br. Vol., 2006, 88(7), 841–851 CrossRef CAS
. - S. Thomopoulos, G. R. Williams and L. J. Soslowsky, Tendon to bone healing: differences in biomechanical, structural, and compositional properties due to a range of activity levels, J. Biomech. Eng., 2003, 125(1), 106–113 CrossRef CAS PubMed
. - G. R. Wohl, R. C. Shymkiw, J. R. Matyas, R. Kloiber and R. F. Zernicke, Periarticular cancellous bone changes following anterior cruciate ligament injury, J. Appl. Physiol., 2001, 91(1), 336–342 CrossRef CAS
. - K. Ditsios, M. I. Boyer, N. Kusano, R. H. Gelberman and M. J. Silva, Bone loss following tendon laceration, repair and passive mobilization, J. Orthop. Res., 2003, 21(6), 990–996 CrossRef
. - E. R. Cadet, G. C. Vorys, R. K. Rahman, S.-H. Park, T. R. Gardner and F. Y. Lee, et al., Improving bone density at the rotator cuff footprint increases supraspinatus tendon failure stress in a rat model, J. Orthop. Res., 2010, 28(3), 308–314 CrossRef
. - L. Galatz, S. Rothermich, K. VanderPloeg, B. Petersen, L. Sandell and S. Thomopoulos, Development of the supraspinatus tendon-to-bone insertion: localized expression of extracellular matrix and growth factor genes, J. Orthop. Res., 2007, 25(12), 1621–1628 CrossRef PubMed
. - H. H. Lu and S. Thomopoulos, Functional Attachment of Soft Tissues to Bone: Development, Healing, and Tissue Engineering, Annu. Rev. Biomed. Eng., 2013, 15(1), 201–226 CrossRef CAS
. - C. Yang, M. W. Tibbitt, L. Basta and K. S. Anseth, Mechanical memory and dosing influence stem cell fate, Nat. Mater., 2014, 13(6), 645–652 CrossRef CAS PubMed
. - H. M. Shaw and M. Benjamin, Structure-function relationships of entheses in relation to mechanical load and exercise, Scand. J. Med. Sci. Sports, 2007, 17(4), 303–315 CrossRef CAS
. - M. Benjamin and J. R. Ralphs, Fibrocartilage in tendons and ligaments — an adaptation to compressive load, J. Anat., 1998, 193(4), 481–494 CrossRef
. - S. Thomopoulos, H.-M. Kim, S. Y. Rothermich, C. Biederstadt, R. Das and L. M. Galatz, Decreased muscle loading delays maturation of the tendon enthesis during postnatal development, J. Orthop. Res., 2009, 27(1), 141 CrossRef
. - A. Schwartz, J. Lipner, J. Pasteris, G. Genin and S. Thomopoulos, Muscle loading is necessary for the formation of a functional tendon enthesis, Bone, 2013, 55(1), 44–51 CrossRef CAS PubMed
. - A. J. Gimbel, Long Durations of Immobilization in the Rat Result in Enhanced Mechanical Properties of the Healing Supraspinatus Tendon Insertion Site, J. Biomech. Eng., 2006, 129(3), 400–404 CrossRef PubMed
. - M. Asheesh Bedi, M. David Kovacevic, J. S. Alice, M. Fox, W. Carl, P. Imhauser, M. Mark Stasiak and P. M. Jonathan, et al., Effect of Early and Delayed Mechanical Loading on Tendon-to-Bone Healing After Anterior Cruciate Ligament Reconstruction, J. Bone Jt. Surg., Am. Vol., 2010, 92(14), 2387–2401 CrossRef PubMed
. - H. Lu, F. Liu, H. Chen, C. Chen, J. Qu and D. Xu, et al., The effect of low-intensity pulsed ultrasound on bone–tendon junction healing: Initiating after inflammation stage, J. Orthop. Res., 2016, 34(10), 1697–1706 CrossRef PubMed
. - H. Lu, L. Qin, P. Fok, W. Cheung, K. Lee and X. Guo, et al., Low-intensity pulsed ultrasound accelerates bone–tendon junction healing: a partial patellectomy model in rabbits, Am. J. Sports Med., 2006, 34(8), 1287–1296 CrossRef PubMed
. - H. H. Lu and J. Jiang, Interface tissue engineering and the formulation of multiple-tissue systems, Adv. Biochem. Eng./Biotechnol., 2006, 102, 91–111 CrossRef CAS
. - J. Jiang, N. L. Leong, J. C. Mung, C. Hidaka and H. H. Lu, Interaction between zonal populations of articular chondrocytes suppresses chondrocyte mineralization and this process is mediated by PTHrP, Osteoarthritis Cartilage, 2008, 16(1), 70–82 CrossRef CAS PubMed
. - J. P. Spalazzi, K. L. Dionisio, J. Jiang and H. H. Lu, Osteoblast and chondrocyte interactions during coculture on scaffolds, IEEE Eng. Med. Biol. Mag., 2003, 22(5), 27–34 Search PubMed
. - I.-N. E. Wang, J. Shan, R. Choi, S. Oh, C. K. Kepler and F. H. Chen, et al., Role of osteoblast-fibroblast interactions in the formation of the ligament-to-bone interface, J. Orthop. Res., 2007, 25(12), 1609–1620 CrossRef CAS PubMed
. - D. K. G. Vogel, A. Ördög, G. Pogány and J. Oláh, Proteoglycans in the compressed region of human tibialis posterior tendon and in ligaments, J. Orthop. Res., 1993, 11(1), 68–77 CrossRef PubMed
. - K. G. Vogel, The effect of compressive loading on proteoglycan turnover in cultured fetal tendon, Connect. Tissue Res., 1996, 34(3), 227–237 CrossRef CAS PubMed
. - A. Takimoto, M. Oro, Y. Hiraki and C. Shukunami, Direct conversion of tenocytes into chondrocytes by Sox9, Exp. Cell Res., 2012, 318(13), 1492–1507 CrossRef CAS PubMed
. - Z. Huang, Y. Zhang, M. Kotaki and S. Ramakrishna, A review on polymer nanofibers by electrospinning and their applications in nanocomposites, Compos. Sci. Technol., 2003, 63(15), 2223–2253 CrossRef CAS
. - Y. Zhang, X. Liu, L. Zeng, J. Zhang, J. Zuo and J. Zou, et al., Polymer Fiber Scaffolds for Bone and Cartilage Tissue Engineering, Adv. Funct. Mater., 2019, 29(36), 1903279 CrossRef
. - I. Alghoraibi, and S. Alomari, Different Methods for Nanofiber Design and Fabrication, 2018, Handbook of Nanofibers, pp. 1–46 Search PubMed
. - N. G. Rim, C. S. Shin and H. Shin, Current approaches to electrospun nanofibers for tissue engineering, Biomed. Mater., 2013, 8(1), 014102 CrossRef CAS PubMed
. - H. Yoshimoto, Y. M. Shin, H. Terai and J. P. Vacanti, A biodegradable nanofiber scaffold by electrospinning and its potential for bone tissue engineering, Biomaterials, 2003, 24(12), 2077–2082 CrossRef CAS PubMed
. - Y. Zheng, N. Meng and B. Xin, Effects of Jet Path on Electrospun Polystyrene Fibers, Polymers, 2018, 10(No.8), 842 CrossRef PubMed
. - S. Ghazanfari, A. Khademhosseini and T. H. Smit, Mechanisms of lamellar collagen formation in connective tissues, Biomaterials, 2016, 97, 74–84 CrossRef CAS PubMed
. - J. Lin, Y. Shi, Y. Men, X. Wang, J. Ye and C. Zhang, Mechanical Roles in Formation of Oriented Collagen Fibers, Tissue Eng., Part B, 2020, 26(2), 116–128 CrossRef CAS PubMed
. - M. Ghollasi and D. Poormoghadam, Enhanced neural differentiation of human-induced pluripotent stem cells on aligned laminin-functionalized polyethersulfone nanofibers; a comparison between aligned and random fibers on neurogenesis, J. Biomed. Mater. Res., Part A, 2022, 110(3), 672–683 CrossRef CAS PubMed
. - D. Sankar, U. Mony and J. Rangasamy, Combinatorial effect of plasma treatment, fiber alignment and fiber scale of poly (ε-caprolactone)/collagen multiscale fibers in
inducing tenogenesis in non-tenogenic media, Mater. Sci. Eng., C, 2021, 127, 112206 CrossRef CAS PubMed
. - S. B. Orr, A. Chainani, K. J. Hippensteel, A. Kishan, C. Gilchrist and N. W. Garrigues, et al., Aligned multilayered electrospun scaffolds for rotator cuff tendon tissue engineering, Acta Biomater., 2015, 24, 117–126 CrossRef CAS PubMed
. - Z. Yin, X. Chen, H. X. Song, J. J. Hu, Q. M. Tang and T. Zhu, et al., Electrospun scaffolds for multiple tissues regeneration in vivo through topography dependent induction of lineage specific differentiation, Biomaterials, 2015, 44, 173–185 CrossRef CAS PubMed
. - X. Li, R. Cheng, Z. Sun, W. Su, G. Pan and S. Zhao, et al., Flexible bipolar nanofibrous membranes for improving gradient microstructure in tendon-to-bone healing, Acta Biomater., 2017, 61, 204–216 CrossRef CAS PubMed
. - J. Xie, X. Li, J. Lipner, C. N. Manning, A. G. Schwartz and S. Thomopoulos, et al., “Aligned-to-random” nanofiber scaffolds for mimicking the structure of the tendon-to-bone insertion site, Nanoscale, 2010, 2(6), 923–926 RSC
. - A. P. Kishan, A. B. Robbins, S. F. Mohiuddin, M. Jiang, M. R. Moreno and E. M. Cosgriff-Hernandez, Fabrication of macromolecular gradients in aligned fiber scaffolds using a combination of in-line blending and air-gap electrospinning, Acta Biomater., 2017, 56, 118–128 CrossRef CAS PubMed
. - R. K. Tindell, L. P. Busselle and J. L. Holloway, Magnetic fields enable precise spatial control over electrospun fiber alignment for fabricating complex gradient materials, J. Biomed. Mater. Res., Part A, 2023, 111(6), 778–789 CrossRef CAS PubMed
. - S. Cong, Y. Sun, J. Lin, S. Liu and J. Chen, A Synthetic Graft With Multilayered Co-Electrospinning Nanoscaffolds for Bridging Massive Rotator Cuff Tear in a Rat Model, Am. J. Sports Med., 2020, 48(No.8), 1826–1836 CrossRef PubMed
. - W. E. Teo and S. Ramakrishna, A review on electrospinning design and nanofibre assemblies, Nanotechnology, 2006, 17(14), R89–r106 CrossRef CAS PubMed
. - X. Cai, P. Zhu, X. Lu, Y. Liu, T. Lei and D. Sun, Electrospinning of very long and highly aligned fibers, J. Mater. Sci., 2017, 52(24), 14004–14010 CrossRef CAS
. - J. A. Ajao, A. A. Abiona, S. Chigome, A. Y. Fasasi, G. A. Osinkolu and M. Maaza, Electric-magnetic field-induced aligned electrospun poly (ethylene oxide) (PEO) nanofibers, J. Mater. Sci., 2010, 45(9), 2324–2329 CrossRef CAS
. - W. E. Teo, M. Kotaki, X. M. Mo and S. Ramakrishna, Porous tubular structures with controlled fibre orientation using a modified electrospinning method, Nanotechnology, 2006, 16(6), 918–924 CrossRef
. - L. M. Bellan and H. G. Craighead, Control of an electrospinning jet using electric focusing and jet-steering fields, J. Vac. Sci. Technol., B: Microelectron. Nanometer Struct.--Process., Meas., Phenom., 2006, 24(6), 3179–3183 CrossRef CAS
. - A. M. Afifi, H. Nakajima, H. Yamane, Y. Kimura and S. Nakano, Fabrication of aligned poly(L-lactide) fibers by electrospinning and drawing(Article), Macromol. Mater. Eng., 2009, 294(10), 658–665 CrossRef CAS
. - X. Zong, H. Bien, C. Chung, L. Yin, D. Fang and B. Hsiao, et al., Electrospun fine-textured scaffolds for heart tissue constructs, Biomaterials, 2005, 26(26), 5330–5338 CrossRef CAS PubMed
. - X. Zong, S. Ran, D. Fang, B. S. Hsiao and B. Chu, Control of structure, morphology and property in electrospun poly (glycolide-co-lactide) non-woven membranes via post-draw treatments, Polymer, 2003, 44(17), 4959–4967 CrossRef CAS
. - C. Yu, T. Wang, H. Diao, N. Liu, Y. Zhang and H. Jiang, et al., Photothermal-Triggered Structural Change of Nanofiber Scaffold Integrating with Graded Mineralization to Promote Tendon–Bone Healing, Adv. Fiber Mater., 2022, 4(4), 908–922 CrossRef CAS
. - T. Lou, X. Wang, G. Song, Z. Gu and Z. Yang, Fabrication of PLLA/β-TCP nanocomposite scaffolds with hierarchical porosity for bone tissue engineering, Int. J. Biol. Macromol., 2014, 69, 464–470 CrossRef CAS PubMed
. - H. Cao and N. Kuboyama, A biodegradable porous composite scaffold of PGA/beta-TCP for bone tissue engineering, Bone, 2010, 46(2), 386–395 CrossRef CAS PubMed
. - S. Sahoo, S. L. Toh and J. C. Goh, A bFGF-releasing silk/PLGA-based biohybrid scaffold for ligament/tendon tissue engineering using mesenchymal progenitor cells, Biomaterials, 2010, 31(11), 2990–2998 CrossRef CAS PubMed
. - W. Liu, J. Lipner, J. Xie, C. N. Manning, S. Thomopoulos and Y. Xia, Nanofiber scaffolds with gradients in mineral content for spatial control of osteogenesis, ACS Appl. Mater. Interfaces, 2014, 6(4), 2842–2849 CrossRef CAS PubMed
. - R. James, S. G. Kumbar, C. T. Laurencin, G. Balian and A. B. Chhabra, Tendon tissue engineering: adipose-derived stem cell and GDF-5 mediated regeneration using electrospun matrix systems, Biomed. Mater., 2011, 6(No.2), 025011 CrossRef CAS PubMed
. - T. J. Sill and H. Av Recum, Electrospinning: Applications in drug delivery and tissue engineering, Biomaterials, 2008, 29(No.13), 1989–2006 CrossRef CAS PubMed
. - P. P. Vergroesen, R. J. Kroeze, M. N. Helder and T. H. Smit, The use of poly(L-lactide-co-caprolactone) as a scaffold for adipose stem cells in bone tissue engineering: application in a spinal fusion model, Macromol. Biosci., 2011, 11(6), 722–730 CrossRef CAS PubMed
. - X. Wang, E. Wenk, X. Zhang, L. Meinel, G. Vunjak-Novakovic and D. L. Kaplan, Growth factor gradients via microsphere delivery in biopolymer scaffolds for osteochondral tissue engineering, J. Controlled Release, 2009, 134(2), 81–90 CrossRef CAS PubMed
. - X. Chen, Z. Yin, J. L. Chen, H. H. Liu, W. L. Shen and Z. Fang, et al., Scleraxis-overexpressed human embryonic stem cell-derived mesenchymal stem cells for tendon tissue engineering with knitted silk-collagen scaffold, Tissue Eng., Part A, 2014, 20(11–12), 1583–1592 CrossRef CAS PubMed
. - S. Y. Kwon, J. W. Chung, H. J. Park, Y. Y. Jiang, J. K. Park and Y. K. Seo, Silk and collagen scaffolds for tendon reconstruction. Proceedings of the Institution of Mechanical Engineers Part H, J. Eng. Med., 2014, 228(4), 388–396 CrossRef PubMed
. - X. Cheng, C. Tsao, V. L. Sylvia, D. Cornet, D. P. Nicolella and T. L. Bredbenner, et al., Platelet-derived growth-factor-releasing aligned collagen-nanoparticle fibers promote the proliferation and tenogenic differentiation of adipose-derived stem cells, Acta Biomater., 2014, 10(3), 1360–1369 CrossRef CAS PubMed
. - W. Shen, X. Chen, Y. Hu, Z. Yin, T. Zhu and J. Hu, et al., Long-term effects of knitted silk-collagen sponge scaffold on anterior cruciate ligament reconstruction and osteoarthritis prevention, Biomaterials, 2014, 35(28), 8154–8163 CrossRef CAS PubMed
. - E. Naghashzargar, S. Farè, V. Catto, S. Bertoldi, D. Semnani and S. Karbasi, et al., Nano/micro hybrid scaffold of PCL or P3HB nanofibers combined with silk fibroin for tendon and ligament tissue engineering, J. Appl. Biomater. Funct. Mater., 2015, 13(2), e156–e168 CAS
. - S. B. Sulaiman, T. K. Keong, C. H. Cheng, A. B. Saim and R. B. Idrus, Tricalcium phosphate/hydroxyapatite (TCP-HA) bone scaffold as potential candidate for the formation of tissue engineered bone, Indian J. Med. Res., 2013, 137(6), 1093–1101 Search PubMed
. - Ba L. N. Thuy, D. Mondal and B. T. Lee, In vitro study of CaTiO3-hydroxyapatite composites for bone tissue engineering, ASAIO J., 2014, 60(6), 722–729 CrossRef PubMed
. - S. Ozkan, D. M. Kalyon and X. Yu, Functionally graded beta-TCP/PCL nanocomposite scaffolds: in vitro evaluation with human fetal osteoblast cells for bone tissue engineering, J. Biomed. Mater. Res., Part A, 2010, 92(3), 1007–1018 CrossRef PubMed
. - R. Detsch, S. Alles, J. Hum, P. Westenberger, F. Sieker and D. Heusinger, et al., Osteogenic differentiation of umbilical cord and adipose derived stem cells onto highly porous 45S5 Bioglass®-based scaffolds, J. Biomed. Mater. Res., Part A, 2015, 103(3), 1029–1037 CrossRef PubMed
. - W. Lu, K. Ji, J. Kirkham, Y. Yan, A. R. Boccaccini and M. Kellett, et al., Bone tissue engineering by using a combination of polymer/Bioglass composites with human adipose-derived stem cells, J. Cell Tissue Res., 2014, 356(1), 97–107 CrossRef CAS PubMed
. - Y. Lv, X. Sang, Z. Tian, S. Jiang, C. Li and Q. Guo, et al., Electrospun hydroxyapatite loaded L-polylactic acid aligned nanofibrous membrane patch for rotator cuff repair, Int. J. Biol. Macromol., 2022, 217, 180–187 CrossRef CAS PubMed
. - B. S. Kim, E. J. Kim, J. S. Choi, J. H. Jeong, C. H. Jo and Y. W. Cho, Human collagen-based multilayer scaffolds for tendon-to-bone interface tissue engineering, J. Biomed. Mater. Res., Part A, 2014, 102(11), 4044–4054 CrossRef PubMed
. - C. Erisken, D. M. Kalyon and H. Wang, Functionally graded electrospun polycaprolactone and beta-tricalcium phosphate nanocomposites for tissue engineering applications, Biomaterials, 2008, 29(30), 4065–4073 CrossRef CAS PubMed
. - X. Li, J. Xie, J. Lipner, X. Yuan, S. Thomopoulos and Y. Xia, Nanofiber scaffolds with gradations in mineral content for mimicking the tendon-to-bone insertion site, Nano Lett., 2009, 9(7), 2763–2768 CrossRef CAS PubMed
. - J. Reifenrath, M. Wellmann, M. Kempfert, N. Angrisani, B. Welke and S. Gniesmer, et al., TGF-β3 Loaded Electrospun Polycaprolacton Fibre Scaffolds for Rotator Cuff Tear Repair: An in Vivo Study in Rats, Int. J. Mol. Sci., 2020, 21(3) CAS
. - H. Gao, L. Wang, Z. Lin, H. Jin, Y. Lyu and Y. Kang, et al., Bi-lineage inducible and immunoregulatory electrospun fibers scaffolds for synchronous regeneration of tendon-to-bone interface, Mater. Today Bio, 2023, 22, 100749 CrossRef CAS PubMed
. - R. Yang, Y. Zheng, Y. Zhang, G. Li, Y. Xu and Y. Zhang, et al., Bipolar Metal Flexible Electrospun Fibrous Membrane Based on Metal-Organic Framework for Gradient Healing of Tendon-to-Bone Interface Regeneration, Adv. Healthcare Mater., 2022, 11(12), e2200072 CrossRef PubMed
.
Footnote |
† Chengzhi Liang and Zaiwei Fan contribute equally. |
|
This journal is © The Royal Society of Chemistry 2024 |
Click here to see how this site uses Cookies. View our privacy policy here.