DOI:
10.1039/D4RA04402A
(Review Article)
RSC Adv., 2024,
14, 20884-20897
The utilization of quantum dot labeling as a burgeoning technique in the field of biological imaging
Received
16th June 2024
, Accepted 27th June 2024
First published on 2nd July 2024
Abstract
Quantum dots (QDs), with their unique optical and physical properties, have revolutionized the field of biological imaging, providing researchers with tools to explore cellular processes and molecular interactions in unprecedented detail. This review explores the diverse properties of QDs, emphasizing their application in biological imaging and addressing both their advantages and challenges. We discuss the developments in QD technology that have facilitated their integration into bioimaging, highlighting the role of surface modifications in enhancing their biocompatibility and functionality. The varied applications of QDs in both in vitro and in vivo imaging settings are examined, showcasing their capacity to deliver brighter, more stable, and multiplexed imaging solutions compared to traditional fluorescent dyes. Furthermore, we delve into the challenges associated with QD use, particularly concerns regarding their potential toxicity and long-term effects on biological systems, and explore ongoing research aimed at mitigating these issues. Finally, we discuss future directions in QD technology, anticipating advancements that will further solidify their role in biological imaging and open up new avenues for scientific exploration.
1. Introduction
Quantum dots (QDs), semiconductor nanocrystals ranging from 1–100 nm in size, have emerged as a revolutionary tool in biological imaging, offering a peek into the intricate workings of living organisms at the cellular and molecular levels.1,2 The adoption of QDs in biological imaging has been driven by their unparalleled optical properties, including size-dependent fluorescence, exceptional photostability, and high quantum yield, which collectively surpass the capabilities of traditional fluorescent dyes and enhance resolution, stability, and specificity in imaging applications.3,4 The exceptional photostability of QDs is particularly significant compared to traditional fluorescent dyes, which are prone to photobleaching. This allows for prolonged imaging sessions without signal degradation, ensuring consistent and high-quality images. Additionally, QDs reduce the risk of phototoxicity to biological samples, making them safer for long-term observation. Additionally, the surface of QDs can be functionalized with various biomolecules, such as antibodies, peptides, or nucleic acids, through bioconjugation techniques. This enables specific targeting and imaging of biological structures or processes with high specificity and multifunctionality, which is particularly significant compared to traditional fluorescent dyes. QDs can achieve multi-target detection without signal interference, provide high sensitivity with low background noise, and offer stable and reproducible results in various biological environments. The stability of QDs in biological environments is also a crucial factor, as it ensures consistent performance and reduces potential cytotoxicity. Encapsulation strategies and surface modifications are commonly employed to enhance the stability and biocompatibility of QDs in in vivo and in vitro applications.
These QDs can be broadly categorized into three main types based on their core composition: Cd-based metal QDs, Cd-free metal QDs, and metal-free QDs, as depicted in Fig. 1. Traditionally, Cd-based core QDs, encompassing variants such as CdTe, CdSe, and CdS, have been considered the gold standard for bioimaging applications.5 However, it has been discovered that these QDs are capable of releasing highly toxic Cd ions.6 To counteract this biotoxicity,7 a common approach has been to encapsulate the core with a protective shell composed of materials like ZnS, ZnSe, or a CdS/ZnS hybrid.8 This not only significantly enhances luminescence efficiency but also acts as a barrier, preventing the release of toxic heavy metal ions.9 Nevertheless, it is important to note that the intracellular environment, particularly within cell lysosomes, is acidic, which means that the protective shell is not fully effective in inhibiting the release of cadmium ions, leading to a limited protective effect.10–12
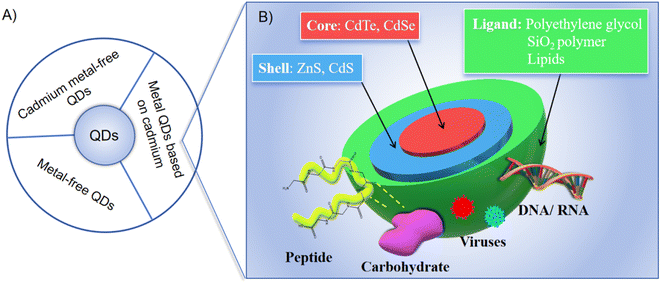 |
| Fig. 1 (A) Classification of QDs; (B) metal QDs based on cadmium.5 | |
In light of the intrinsic drawbacks associated with traditional cadmium-containing metal QDs, there has been a noticeable trend towards the exploration and adoption of safer alternatives, specifically Cd-free metal QDs and metal-free QD materials, for bioimaging applications.13–15
This review aims to meticulously explore the role of QDs in biological imaging, delving into their properties, diverse applications, and ongoing research endeavors that are continually broadening their horizons. By providing a balanced discourse on their advantages and challenges, this article seeks to underscore the transformative influence of QDs on biological imaging, spotlighting their potential to catalyze future breakthroughs in the life sciences.
2. Properties of quantum dots
2.1 Photostability and brightness
One of the most significant advantages of QDs over traditional fluorescent dyes is their exceptional photostability.16,17 QDs can endure prolonged exposure to intense light without undergoing photobleaching, ensuring consistent and reliable imaging across extended durations.18,19 Tang et al. developed NB2C quantum dots with excellent photostability and biodegradability may very well prove to be useful for future applications in environmental monitoring, biomedical diagnostics, visual displays, and anti-counterfeiting.20
The core composition of QDs, typically made from semiconductor materials such as cadmium selenide (CdSe), cadmium sulfide (CdS), or silicon (Si), plays a crucial role in their photostability and brightness. These materials have unique electronic properties that allow for high quantum yield and resistance to photobleaching. For instance, CdSe/ZnS core–shell QDs are well-known for their bright and stable emission, making them ideal for long-term cellular imaging studies. To further enhance their performance, QDs often have a protective shell made from materials like zinc sulfide (ZnS) or zinc selenide (ZnSe), which helps to improve their fluorescence efficiency and prevent degradation under intense light exposure. This shell structure not only enhances the photostability but also helps in reducing the non-radiative recombination of excitons, thereby increasing the overall brightness. Moreover, the solubility and stability of QDs can be improved by modifying ligands or functional groups, such as mercaptan groups or carboxyl groups, while reducing surface defects and improving fluorescence efficiency.21
In addition to their stability, QDs are known for their high quantum yield, which translates to unparalleled brightness. Gongalsky et al. prepared high-luminosity, low-toxicity, ultrapure silicon-based quantum dots, promising to be unique for cancer therapeutics applications.22 Similarly, graphene quantum dots have shown high photostability and strong fluorescence, making them suitable for bioimaging and biosensing applications. This brightness enhances the visibility of labeled targets, allowing for more detailed and precise imaging.23 The combination of photostability and brightness ensures that QDs provide robust and reliable signals, even in challenging imaging conditions. Furthermore, advancements in QD technology have led to the development of cadmium-free quantum dots, such as copper indium sulfide (CIS) and silicon-based quantum dots, which offer high brightness and stability while mitigating potential toxicity concerns. For example, CIS/ZnS QDs have been used successfully in in vivo imaging due to their favorable optical properties and reduced toxicity. Metal-free quantum dots like carbon dots and graphene quantum dots also offer high brightness and photostability, broadening the scope of QD applications in various fields, from medical diagnostics to optoelectronics.
Overall, the unique material composition and structural design of QDs provide significant advantages in terms of photostability and brightness over traditional fluorescent dyes, making them indispensable tools in modern biological imaging and beyond.
2.2 Tunable emission
The size, shape, and composition of QDs dictate their fluorescence properties, allowing for tunable emission across a broad spectrum. By manipulating these parameters, researchers can tailor the fluorescence of QDs to suit specific imaging needs.24 This tunability is a critical advantage in multiplexed imaging, where the ability to differentiate between multiple targets is essential.25 The core composition of QDs, typically made from semiconductor materials such as cadmium selenide (CdSe), cadmium sulfide (CdS), indium phosphide (InP), or silicon (Si), plays a crucial role in determining their emission wavelength. When visible light from the outside irradiates the semiconductor material, photons stimulate some electrons to jump to higher energy levels. When the electrons return to a stable ground state, the material emits light at a specific frequency, depending on the composition and size of the QDs.26 For instance, CdSe QDs can be precisely tuned to emit light across the visible spectrum by adjusting their size, with smaller QDs emitting blue light and larger QDs emitting red light. Similarly, InP QDs offer a cadmium-free alternative with tunable emission properties, making them suitable for applications where lower toxicity is desired.
Generally speaking, smaller QDs tend to emit light at shorter wavelengths, producing blues and greens, while larger QDs emit at longer wavelengths, resulting in reds and near-infrared colors (Fig. 2).27,28 This size-dependent emission enables the simultaneous visualization of multiple QD populations, each labeled with a different target, providing a comprehensive view of complex biological systems.29 Additionally, the shape of QDs, such as spherical or rod-like structures, can also influence their optical properties, further expanding the tunability of their emission. Protective shell materials, such as zinc sulfide (ZnS) or zinc selenide (ZnSe), are often used to enhance the quantum yield and stability of the QDs, ensuring consistent performance across different biological environments.30
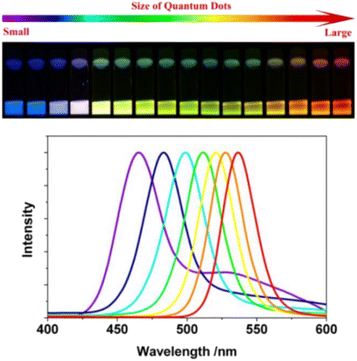 |
| Fig. 2 Emission colors and photoluminescence spectra of ODs with different size.28 | |
By carefully selecting and engineering the core and shell materials, researchers can create QDs with specific emission properties tailored to a wide range of imaging applications. This tunable emission capability, combined with their inherent brightness and stability, makes QDs an invaluable tool for advanced biological imaging and multiplexed diagnostics.
2.3 Bioconjugation
Compared with traditional organic fluorescent molecules, quantum dots have obvious advantages, but their high toxicity and poor biocompatibility are the key factors that can not be ignored. If quantum dots are to play a more important role in the field of living biological imaging, the negative effects caused by its structure must be solved.31,32 Among them, quantum dot biological coupling technology is a very promising way. Quantum dots bind to different biomolecules (such as antibodies, proteins, peptides, aptamer nucleic acids, small molecules, liposomes, lectins and monosaccharides) in covalent or non-covalent ways to specifically identify biomolecule targets. This process is a key step to enable quantum dots to cross the cell membrane and target inside and outside the cell through different pathways, and is widely used in the field of specific and non-specific targeting and biological imaging.
2.3.1 Classification of biological coupling of quantum dots. Generally speaking, the biological coupling of quantum dots can be divided into covalent coupling and non-covalent coupling. Covalent binding is a covalent reaction between QDs and biomolecule functional groups to form covalent bonds. Non-covalent binding is the hydrophobic, electrostatic and other non-covalent interactions between biomolecules and ligands on QDs surface or QDs surface.
2.3.1.1 Covalent combination mode. The methods of covalent binding include covalent binding of amino and carboxyl groups, covalent binding of amino and sulfhydryl groups, and covalent binding of aldehydes and hydrazides. Covalent binding of amino and carboxyl groups usually uses zero-length cross-linking agent EDC, which activates carboxyl groups on the surface of quantum dots to form amide bonds with amino groups on the surface of antibodies to form amide bonds; covalent binding of amino and sulfhydryl groups is through SMCC reaction, in which DTT activates antibodies to produce sulfhydryl groups, and then binds to maleimide groups on the surface of quantum dots. This method can selectively bind antibody fragments and improve the stability and water solubility of quantum dots. The covalent binding of aldehyde group and acylhydrazide activates the carbohydrate group of antibody F fragment into aldehyde group through oxidation, and then reacts with the hydrazide group on the surface of quantum dots. This method can control the binding ratio of quantum dots to antibodies, thus improving the specificity and stability of the coupling.
2.3.1.2 Non-covalent combination mode. (1) The primary methods for non-covalent bonding of quantum dots (QDs) encompass the following four categories. Firstly, through the biotin/streptavidin system, QDs are surface-functionalized with either biotin or avidin, followed by conjugation with biomolecules modified with corresponding ligands. The interaction between avidin and biotin exhibits high affinity, rapid binding kinetics, specificity, stability, and multi-stage amplification effect. For instance, Xin Huang et al. demonstrated a specific application of this system where Ag2S QDs were surface-modified with a PD-L1 aptamer through DNA modification. This modification enabled the QDs to selectively recognize and bind to PD-L1 overexpressing thermal tumor cells. Such targeted binding enhances imaging selectivity and accuracy, minimizing effects on normal tissues.33 This approach enables efficient labeling of antibodies and enzymes labeled with biotin without compromising their activity.(2) Biomolecules can be directly connected to the surface of QDs by covalent binding, for example, using the mercaptan group of cysteine or the imidazole group of histidine to form a bond with metal atoms on the surface of QDs. Compared with biotin–avidin binding, this histidine labeling method has many advantages: it can control the direction of ligand binding (histidine tags can easily bind to specific sites of proteins or peptides), compact probe size and low production cost.
(3) Ligands (such as 3-mercaptopropionic acid or dihydrolipoic acid) were connected to the surface of QDs by the ligand exchange method, and then combined with biomolecules through the carboxyl group at the end of the ligand. This coupling method has an outstanding advantage: it can control the antibody to face the antigen binding site outward, ensuring that the activity of the antibody remains unchanged after coupling with the quantum dots. It is through this coupling principle that Peng Zhang et al. successfully developed nanoprobes coupled with PbS/CdS core–shell quantum dots and carboxyl ligands, achieving real-time, non-invasive, non-radiation direct visualization of bone health34 (Fig. 3).
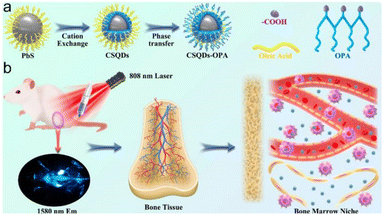 |
| Fig. 3 (a) Schematic diagram for the construction of CSQDs–OPA; (b) schematic illustration of NIR light-guided bone marrow imaging in mice.34 | |
(4) QDs was encapsulated in a shell, using silica or hydrophilic copolymers with high biocompatibility, and then covalently combined with biomolecules by the terminal functional groups in the shell to improve the biocompatibility and fluorescence stability of QDs.
2.3.2 Advantages and applications of biological coupling quantum dots technology. This technique can connect quantum dots with specific biomolecules (such as antibodies, peptides, nucleic acids, etc.) to achieve highly specific labeling and detection of specific cells, molecules or biological structures.35–37 For example, the quantum dots of coupled antibodies can specifically label cancer cells without affecting the surrounding healthy cells, and specific targeting will improve the accuracy and safety of diagnosis and treatment. This specificity is difficult to be achieved by traditional fluorescent dyes.38,39 And quantum dots can be coupled with biocompatible materials such as polyethylene glycol (PEG), which can greatly reduce its immune reaction and toxicity in vivo, and improve its safety in in vivo imaging and diagnosis.40,41 For instance, through the design of a modular peptide library, Qiuju Dai et al. optimized the peptide sequence to achieve high fluorescence, with fluorescence retention of up to 93%. They developed efficient GGT-responsive quantum dots that remained stable in aqueous solution for 3 months and demonstrated strong tumor penetration, showcasing the potential for long-term and effective in vivo imaging (Fig. 4).42
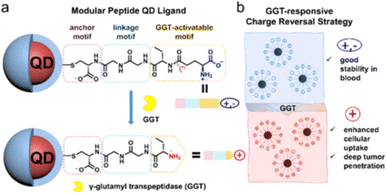 |
| Fig. 4 Design of modular peptides (a) to realize GGT-responsive charge reversal strategy (b).42 | |
Lequeux et al. showcased the significant role of surface modifications by employing directed bioconjugation with complete IgG antibodies to create QD nanobioconjugates. These nanobioconjugates demonstrated exceptional intracellular and extracellular stability and biorecognition capabilities, proving their efficacy in imaging the intricate intracellular dynamics of the transmembrane cell receptor CB1, particularly within neurons (Fig. 5).43 The adaptability provided by various bioconjugation techniques means that QDs can be customized to suit the unique requirements of diverse imaging applications, offering researchers a potent and precise tool for targeted imaging endeavors.44 At the same time, the surface of quantum dots can be coated or modified by biological coupling to reduce the release of toxic ions and improve its safety in biological systems. If some hydrophilic groups (–OH, –NH2, etc.) are connected to the surface of quantum dots, the solubility and stability of quantum dots in aqueous solution can be improved, and the aggregation or precipitation in biological environment can be prevented, thus its fluorescence performance and imaging effect can be maintained. In addition, biological coupling can enable quantum dots to have a variety of functions, such as simultaneous imaging and treatment (such as photodynamic therapy), or combined with different detection methods (such as fluorescence imaging and magnetic resonance imaging).45 To achieve multimodal imaging and comprehensive diagnosis. In short, the biological coupling of quantum dots significantly improves its application value in the biomedical field, making it a powerful tool for high-resolution imaging, disease diagnosis and accurate treatment.
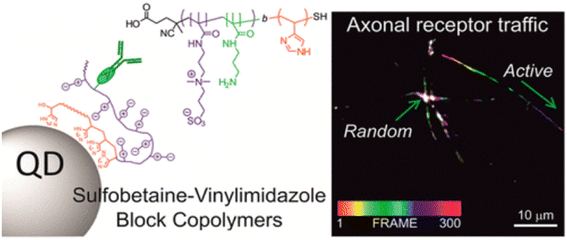 |
| Fig. 5 Schematic diagram of biological coupling of QDs.43 | |
3. Applications in biological imaging
Quantum dots (QDs) have revolutionized biological imaging, providing a sophisticated approach to visualize and analyze intricate biological systems with unparalleled clarity and precision. These nanometer-sized semiconductor particles are versatile tools that can be employed in various domains of biological imaging, including single molecule tracking, cell and tissue imaging, in vivo imaging, and multi-channel imaging (Fig. 6). Due to their inherent optical properties, quantum dots offer remarkable stability within biological environments and the ability to penetrate cellular membranes, allowing for meticulous observation of intracellular activities and interactions. Their multicolor fluorescence capabilities further enhance their application, enabling simultaneous imaging of multiple cellular components to yield a holistic view of cellular function and dynamics. The adoption of quantum dots in biological imaging has substantially deepened our understanding of cellular behavior, interactions, and responses to external stimuli, marking a significant stride forward in both scientific research and medical diagnostics. By integrating quantum dots into the realm of biological imaging, we have gained a powerful tool that promises to continue driving advancements and innovations in the field.
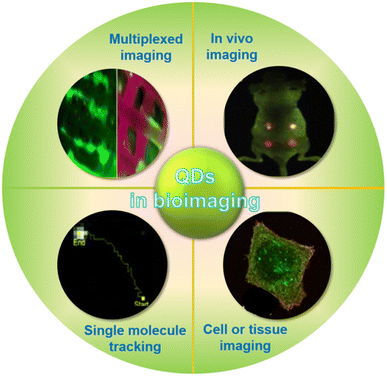 |
| Fig. 6 Application of QDs in biological imaging.51,53 | |
3.1 Single-molecule tracking
The application of quantum dots in single molecule tracking (SMT) is a breakthrough technology that allows researchers to observe the dynamics of individual molecules with unprecedented resolution and sensitivity.46,47 These nanoscale semiconductor crystals stand out in the field of biological imaging because of their excellent brightness and long-term stability.48 Quantum dots emit light with wide absorption and narrow emission spectrum, which makes it possible to track multiple tags in complex biological systems at the same time.49 By precisely adjusting their size, researchers can obtain the required specific fluorescence characteristics, which can be used in the dynamic observation of intracellular molecules, the study of intracellular molecular interactions, and revealing the mechanism of intracellular transport.50,51
The following sections will delve into intriguing topics showcasing the potentials of single QD-based single molecule tracking. These studies include exploring membrane dynamics, understanding the function of neurons, and understanding the life cycle stages of individual viruses in order to better understand their dynamics in living cells. All these emphasize the diversity and transformation of the application of quantum dots in biological research.52,53
Huang et al. have contributed to this field by synthesizing water-soluble quantum dots with diameters ranging from 10–12 nm, which are immediately activatable. Their compact size, combined with stability and high specificity to related biotinylated proteins, enables meticulous observation of molecular dynamics across various biological systems and applications (Fig. 7).54 These QDs' attributes facilitate detailed studies, further enriching our understanding of cellular processes.
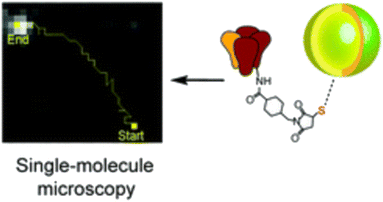 |
| Fig. 7 Single molecule tracking trajectory of QDs.54 | |
In 2003, a significant milestone was reached when Dahan et al. utilized QDs in a single particle tracking (SPT) experiment, imaging the rapid diffusion of QD-tagged glycine receptors in rat spinal cord cultures.55 This experiment marked the beginning of a series of developmental milestones in QD SPT, including multiplexed SPT of up to eight spectrally distinct QD species,56 QD SPT in living rat brain slices,57,58 and 3D QD SPT using advanced optical setups.59 Such advancements underscore the time- and space-dependent variations in molecular behavior, shedding light on diverse cellular phenomena.
In addition to labeling proteins, quantum dots can also be used to track viruses. Zhang et al. reported a method of labeling enveloped viruses with QD, which makes it easy to “see” the virus and track its infection, thus promoting the widespread use of single virus tracking and the discovery of complex infection mechanisms.60
Despite these advancements, challenges remain in the application of QDs, particularly concerning their diffusion through cellular membranes into the cytosol. Techniques such as microinjection and electroporation offer potential solutions, each with its own set of advantages and limitations. Microinjection provides precision and the capability for direct nuclear delivery,61 while electroporation offers a more scalable approach for delivering QDs to multiple cells.62
The application of quantum dots in the field of single molecule tracking, with its extraordinary brightness and stability, provides an unprecedented detailed perspective for revealing molecular dynamics and interactions in living cells.63 This breakthrough technology has greatly promoted the development of molecular biology, enabling us to observe and understand basic biological processes, health and diseases with unprecedented accuracy. In the complex intracellular environment, quantum dots make accurate visualization and long-term tracking of individual molecules possible, thus overcoming many challenges.
However, in practical applications, single molecule tracking technology also faces challenges such as the biocompatibility of quantum dots, high-precision positioning requirements and a large number of data processing. With the continuous progress of imaging technology and data analysis methods, the application of quantum dots in the field of single molecular tracking is becoming more and more mature, which provides a powerful new research tool for the fields of cell biology and molecular biology, enables us to explore the subtle mechanisms of life.
3.2 In vitro cell and tissue imaging
Quantum dots have become a key tool in the field of cell and tissue imaging, and their unique brightness, stability and specificity play an important role in biomedical research.41,64,65 The unique ability of these nanoscale semiconductor crystals enables them to penetrate the biofilm and maintain stability in the cell, thus contributing to the in-depth exploration of intracellular structures and processes.66 In particular, their photostability provides support for long-term imaging research, which is particularly critical in deciphering cell differentiation, signal pathways and responses to various stimuli.67,68
Aizik et al. developed QD (LipQDs) liposome delivery system with high encapsulation yield, which has strong optical properties, fluorescence stability and low cytotoxicity, which may be a promising imaging strategy for inflammatory tissue.65
The amine and sulfonate co-functionalized graphene quantum dots GQDs synthesized by Wang et al. exhibit two-photon fluorescence properties, no cytotoxicity and minimal photothermal effects, and can be used as stable and efficient one-photon and two-photon fluorescent probes for cellular imaging.69
Cai et al. developed a powerful and versatile host-guest peptide (HGP) toolkit for creating highly stable and specific peptide-based quantum dots for extracellular matrix and cell imaging. The HGP system includes host peptides and guest peptides with specific sequence patterns, thus improving the stability and specificity of quantum dots to target biomarkers. Six kinds of multicolor HGP modified quantum dots have been developed, which are specifically used to label extracellular matrix proteins and major cellular components, providing an effective tool for real-time monitoring of extracellular matrix proteins.70
The wide absorption and narrow emission spectra of quantum dots not only enable the use of a single wavelength light source to excite a variety of different colors of quantum dots, but also promote the development of multi-parameter imaging technology.71 They play a key role in cell type identification, visualization of cell internal structure, pathological research and cell dynamic tracking.65 Although there are challenges in biocompatibility and deep tissue imaging, with the continuous progress of technology, the application prospect of quantum dots in the biomedical field is still very broad, providing us with a powerful and accurate research tool.
3.3 In vivo imaging
The application of quantum dots in the field of in vivo imaging is one of its most challenging and valuable directions.72 Their high brightness and stability make it possible to visualize biological processes in vivo clearly and in detail even in deep tissues, opening up new possibilities in the fields of developmental biology, oncology and neuroscience.73 This capacity provides us with insights into disease progression, treatment responses and basic biological processes.74
The journey of quantum dots in the human body includes several key steps: uptake, fixation, imaging and excretion. Through the surface modification and biomolecule modification of quantum dots, their biocompatibility is improved, which makes them more safe for in vivo imaging.75 Their unique targeting imaging ability, especially the characteristics of polychromatic imaging, make them particularly important in the study of disease models, drug dynamic monitoring, cell migration and immune response and other complex biological processes.76
3.3.1 Ingestion. The entry of quantum dots into the human body is facilitated through three principal methods. Injection: This method introduces quantum dots directly into the bloodstream or tissue, bypassing external barriers. Inhalation: Quantum dots can be inhaled as aerosols or particles, entering the respiratory system. Oral ingestion: Consumption of quantum dots leads to their absorption by the digestive system (Fig. 8).
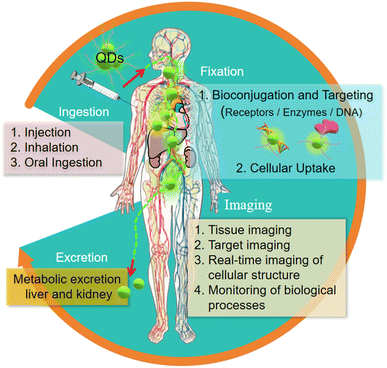 |
| Fig. 8 The imaging process of quantum dots in vivo.76 | |
3.3.2 Fixation. Post entry, quantum dots undergo a surface modification to enhance biocompatibility and targeting. Within the body, these engineered quantum dots seek out and bind to specific biomolecules—receptors, enzymes, or DNA. This bioconjugation allows for the precise localization of quantum dots to designated cells or tissues.
3.3.3 Imaging. Once internalized by cells, quantum dots serve as tools for a spectrum of imaging tasks. Tissue imaging: Quantum dots help delineate tissue architecture. Target imaging: They spotlight particular entities, such as neoplastic growths or regions of infection. Real-time cellular imaging: They facilitate the observation of cellular processes as they unfold in real time. Biological process monitoring: Quantum dots enable the longitudinal tracking of biological phenomena within the body.
3.3.4 Excretion. The final phase involves the clearance of quantum dots from the body, predominantly through metabolic pathways in the liver and kidneys, akin to the elimination of metabolic waste.The utilization of quantum dots, especially those incorporating heavy metals like cadmium, raises concerns regarding potential long-term accumulation. This has galvanized research towards the development of quantum dots that are both safer for use and biodegradable, to mitigate any adverse effects stemming from their persistence in biological systems.
These characteristics not only enable researchers to directly observe the development of diseases such as tumor growth and metastasis, but also help to monitor the distribution and efficacy of drugs in vivo in real time. Therefore, the application of quantum dots in in vivo imaging not only provides a revolutionary window for biomedical research, but also plays a key role in understanding the nature of life. With the continuous progress of technology, the application prospect of quantum dots in the field of biomedicine will continue to expand.
In the field of oncology, the application of QDs has shown remarkable potential for enhancing tumor imaging and diagnosis. For instance, Sun et al. reported a significant advancement with the development of fluorescent Ag–In–S/ZnS quantum dots (AIS/ZnS QDs) that emit red light, specifically designed for quick tumor drainage lymph node imaging. These AIS/ZnS QDs were synthesized through a solution reaction and dispersed in poly(vinyl pyrrolidone) for effective in vivo applications. Their suitable hydrodynamic radius of 295 nm and good dispersion in water make them apt for lymph node imaging.77 Following this, Ge et al. introduced a novel biocompatible near-infrared fluorescent probe for long-term in vivo imaging of tumors. This innovation involved modifying glucose, which is avidly absorbed by cancer cells, onto the surface of near-infrared Ag2Se quantum dots (NIR Ag2Se QDs), resulting in glucose-functionalized Ag2Se QDs (Glc-Ag2Se QDs). These Glc-Ag2Se QDs demonstrated their ability to target and illuminate tumors in vivo, with their fluorescence being observable for at least seven days, highlighting their potential as a tool for long-term observation of tumor evolution.78 Building on these developments, Wang et al. further expanded the scope of QD application in oncology by developing αvβ3-integrin-targeted quantum-dots-loaded liposome–microbubble (iRGD-QDLM) complexes. These complexes, which enable dual-modality molecular imaging by combining ultrasound with optical imaging, showed excellent binding capability to 4T1 breast cancer cells. Notably, enhanced ultrasound molecular signals were observed in 4T1 tumors using iRGD-QDLM, compared to non-targeted QDLM. This study went a step further by demonstrating that iRGD-QDLM could be delivered into tumors via ultrasound-mediated microbubble destruction and adhere to αvβ3 integrin on breast cancer cells for transvascular fluorescent imaging. This approach opens new possibilities for dual-modality molecular imaging of αvβ3 integrin in tumor tissues, potentially improving the early detection and understanding of tumor progression and metastasis.79
Together, these examples underscore the versatility and efficacy of quantum dots in oncological applications, from lymph node imaging to long-term tumor observation and dual-modality molecular imaging. They represent significant strides in leveraging nanotechnology for cancer diagnosis and treatment, offering innovative solutions for complex biological challenges in oncology.
QDs have shown tremendous potential in a variety of internal applications beyond oncology, offering innovative solutions in medical imaging and diagnostics. Kong et al. made a significant contribution by synthesizing PbS quantum dots (RNase-A@PbS quantum dots) that emit in the second near-infrared biological window, using rapid microwave heating. These QDs, with a high quantum yield of 17.3% and peak emission at 1300 nm, enable deep optical penetration into muscle tissue (up to 1.5 cm) and provide excellent imaging contrast at very low doses of 5.2 pmol (∼1 μg) per mouse. Notably, these protein-coated QDs are non-toxic to model neurons, normal cells, and cancer cells in vitro, making them highly promising for accurate disease screening and diagnostic applications due to their high brightness, strong photostability, and excellent biocompatibility (Fig. 9).80 Building upon this, Deng et al. developed fluorescence and radioisotope QDs for multimodal imaging of macrophages in different sizes and environments, including in vivo, ex vivo, and in situ. They employed a variety of imaging techniques, such as in vivo positron emission tomography/computed tomography (PET/CT), in vivo and ex vivo fluorescence imaging, postmortem isotopic analysis, and optical microscopy, to assess the targeting ability of dextran-mimetic quantum dots in visceral adipose tissue. The results showed that these quantum dots exhibit a high signal-to-noise ratio, excellent optical quantification, long-term photostability, and resistance to chemical fixation.66 Furthermore, Ren et al. explored the applications of hydrophilic and fluorescence stable carbon quantum dots derived from peach leaves in in vitro cell cultures and in vivo zebrafish experiments. These carbon quantum dots (CQDs) demonstrated ultra-low toxicity and exceptional biological imaging performance. Remarkably, even at high concentrations, the CQDs maintained over 90% biological activity and provided bright fluorescence. This study underlines the potential of these biomass-derived CQDs as a safer, more sustainable, and cost-effective alternative for biological imaging applications.81
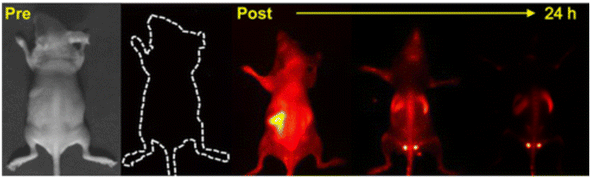 |
| Fig. 9 Imaging of RNase-A@PbS QDs in mice.80 | |
The application of quantum dots in the field of medical imaging shows its ability to provide detailed and accurate imaging in a variety of biological environments and scales. The unique properties of these nanomaterials, such as deep tissue penetration, high brightness and low toxicity, make them valuable tools for promoting medical diagnosis and research.82 Despite the challenges of image depth, resolution and security in the field of in vivo imaging, quantum dots still have a broad application prospect and play a more and more important role in biomedical research and clinical applications.
Especially in the study of tumor growth and metastasis, monitoring the distribution and efficacy of drugs in vivo, quantum dots show its unique value. They not only provide a new perspective for biomedical research, but also provide key support in clinical diagnosis and treatment. With the progress of imaging technology and a better understanding of the toxicity of quantum dots, it is expected that quantum dots will continue to bring innovation and breakthroughs in the field of medical imaging, providing a powerful tool for us to explore and understand the nature of life.
3.4 Multiplexed imaging
Because of its tunable emission properties, quantum dots become an ideal choice for multi-channel imaging, which is a technique that allows multiple biological targets to be visualized at the same time.83,84 The wide absorption and narrow emission spectra of these nanoscale semiconductor crystals make them particularly effective in polychromatic imaging.3,85 Fluorescence emission of many different colors can be achieved by adjusting the size and composition. This ability is essential for understanding complex biological interactions and processes, providing a comprehensive cellular and molecular dynamics perspective.86
In terms of multiple detection, the high brightness and stability of quantum dots make it an ideal marker for disease diagnosis and biomarker analysis.87 It can be excited by a single wavelength light source and emit light of different colors at the same time, which greatly simplifies the complexity of the imaging system and improves the sensitivity and accuracy of detection. This makes quantum dots a powerful tool for the study of diseases such as cancer and can effectively detect multiple tumor markers at the same time.
Sun et al. have introduced a novel dual-channel sensing platform that utilizes sulfur quantum dots for high-throughput metal ion recognition. This innovative platform employs statistical techniques to convert signal changes into unique identifiers for various metal ions, demonstrating its effectiveness as a cost-efficient and powerful tool for environmental testing.88 Furthermore, Tang et al. have contributed to this field by designing a 16-channel high-precision bias source, specifically tailored for quantum dot device experiments. Their design not only provides an effective and feasible topology for experimental applications but also focuses on the precision control of quantum dot devices, enhancing the accuracy and reliability of these experiments.89
Additionally, Sagar et al. have made significant strides in the development of nitrogen-sulfur co-doped graphene quantum dots (NS-GQD) and nitrogen-doped carbon quantum dots (N-CQD) for use in multiphoton imaging. Their research highlights the potential of these quantum dots as dual-wavelength detectors, which are particularly useful in sensing applications and optical nano-temperature measurement, further expanding the versatility of quantum dot technology.90 Lastly, Chen et al. have conducted an in-depth investigation into the quantum transport in multichannel silicon nanowire transistors. This research is crucial for the advancement of quantum logic platforms. By exploring the bias-dependent hole transport spectra in lightly boron-doped multi-channel silicon nanowire transistors, they have provided valuable insights into the mechanisms of quantum transport through multiple channels.91
Although there are challenges in probe design and data analysis, the application prospect of quantum dots in polychromatic imaging and multiple detection is still broad. This technology provides researchers with a versatile tool to help them gain a more comprehensive understanding of life processes at the molecular and cellular levels. The application of quantum dots not only improves the depth and breadth of biological imaging technology, but also deepens our understanding of the practical application of quantum mechanics, and emphasizes its growing importance in the field of science and technology.
4. Challenges and safety concerns
Although the application of quantum dots in biological imaging is full of potential, they also face a series of challenges and safety problems. Among them, the potential toxicity of quantum dots is of particular concern, especially those containing heavy metal elements such as cadmium. The long-term effects of this toxicity on biological systems are not fully understood, so it is necessary to conduct extensive safety assessments to ensure their safe use in biological applications.
To address these problems, research is under way to develop safer and biocompatible quantum dots, with a focus on reducing or eliminating the use of toxic materials and enhancing the biocompatibility of quantum dot surfaces. In addition, another challenge in the application of quantum dots is to accurately calibrate and optimize the imaging system to make full use of the brightness and stability of quantum dots. Although this requires complex and specialized equipment, its unique properties and versatility make quantum dots a valuable tool in biological imaging.
It is expected that ongoing research and technological development will address these challenges and improve the safety and applicability of quantum dots. Through interdisciplinary efforts, combined with nanotechnology and biomedical knowledge, quantum dots are expected to become a safer and more efficient medical imaging tool in the future. With the progress of technology and the solution of security problems, the application prospect of quantum dots in the field of biological imaging is promising, and it is expected to continue to play a role as a transformational tool.
5. Future prospects
The future prospect of quantum dots in the field of biological imaging is extremely broad and anticipated. With the development of non-toxic or low-toxic materials, such as silicon or graphene quantum dots, safer quantum dots are expected to be developed to greatly reduce the potential harm to organisms. These safe quantum dots will provide powerful tools for clinical and biomedical research, especially in highly specific biomarkers, improving the accuracy of early diagnosis of diseases and the effectiveness of therapeutic drugs.
In the future, quantum dots are expected to be integrated with other imaging technologies to form a multimodal imaging system to provide more comprehensive biomedical information. Their applications in real-time dynamic imaging will effectively monitor biological processes such as intracellular signal transduction, tumor growth and drug transport, thus providing researchers with unprecedented insight.
Quantum dots may also promote the development of personalized medicine, improving the effectiveness of treatment by customizing imaging schemes for specific patients. At the same time, their unique properties are expected to promote the exploration of new treatments, such as those that combine drug delivery and imaging.
To sum up, the application of quantum dots in the field of biological imaging not only indicates the great progress of medical research and clinical diagnosis, but also brings possible revolutionary changes in the field of biomedicine. With the continuous innovation and development of technology, the role of quantum dots in the future biological imaging field will become more and more important and diversified.
6. Conclusion
As a powerful tool in the field of biological imaging, quantum dots have significantly enhanced our ability to visualize and study biological processes. Their unique properties, including tunable emission, excellent photostability, and the potential for biological coupling, make them versatile for various applications such as single molecule tracking, cell imaging, in vivo research, and multiplexed imaging, providing researchers with unprecedented clarity and detail.
This review has highlighted specific advancements, such as the integration of luminescent silicon quantum dots with gadolinium ions within PEGylated micelles, demonstrating their utility in both fluorescence microscopy and MRI imaging. Furthermore, we discussed the successful use of cadmium-free quantum dots like CIS/ZnS QDs in in vivo imaging, showcasing their reduced toxicity and high performance.
Despite these significant advancements, quantum dots still face several challenges, particularly concerning their potential toxicity, the requirement for advanced imaging equipment, and ensuring long-term biocompatibility. The toxicity of quantum dots, especially those containing heavy metals like cadmium, raises concerns about their long-term effects on biological systems. Additionally, the need for precise calibration and optimization of imaging systems to fully exploit the brightness and stability of quantum dots necessitates complex and specialized equipment.
Looking forward, the application prospects of quantum dots in biological imaging remain very promising. Advances in non-toxic or low-toxic materials, such as silicon or graphene quantum dots, are expected to develop safer alternatives that minimize potential harm to organisms. These safer quantum dots will be powerful tools for clinical and biomedical research, improving the accuracy of early disease diagnosis and the effectiveness of therapeutic drugs.
Quantum dots are also anticipated to be integrated with other imaging technologies to form multimodal imaging systems, providing more comprehensive biomedical information. Their applications in real-time dynamic imaging will allow for effective monitoring of biological processes such as intracellular signal transduction, tumor growth, and drug transport. Furthermore, quantum dots may promote the development of personalized medicine by customizing imaging schemes for specific patients, thereby improving treatment effectiveness. Their unique properties are expected to facilitate the exploration of new therapies, such as those combining drug delivery and imaging.
To sum up, the application of quantum dots in biological imaging signifies substantial progress in medical research and clinical diagnostics. With continuous innovation and development, the role of quantum dots in biological imaging is set to become increasingly important and diversified, driving forward scientific discovery and innovation in life sciences and medicine. This transformative impact of quantum dots underscores the potential of nanotechnology to drive significant advances in science and medicine.
Data availability
Data sharing not applicable to this article as no datasets were generated or analysed during the current study.
Conflicts of interest
There are no conflicts to declare.
References
- J. Lin, X. Chen and P. Huang, Graphene-based nanomaterials for bioimaging, Adv. Drug Delivery Rev., 2016, 105, 242–254, DOI:10.1016/j.addr.2016.05.013.
- B. B. Karakoçak, A. Laradji, T. Primeau, M. Y. Berezin, S. Li and N. Ravi, Hyaluronan-Conjugated Carbon Quantum Dots for Bioimaging Use, ACS Appl. Mater. Interfaces, 2021, 13(1), 277–286, DOI:10.1021/acsami.0c20088.
- S. Pandey and D. Bodas, High-quality quantum dots for multiplexed bioimaging: A critical review, Adv. Colloid Interface Sci., 2020, 278, 102137, DOI:10.1016/j.cis.2020.102137.
- H. R. A. K. Al-Hetty, A. T. Jalil, J. H. Z. Al-Tamimi, H. G. Shakier, M. Kandeel, M. M. Saleh and M. Naderifar, Engineering and surface modification of carbon quantum dots for cancer bioimaging, Inorg. Chem. Commun., 2023, 149, 110433, DOI:10.1016/j.inoche.2023.110433.
- P. Zrazhevskiy, M. Sena and X. Gao, Designing multifunctional quantum dots for bioimaging, detection, and drug delivery, Chem. Soc. Rev., 2010, 39(11), 4326–4354, 10.1039/B915139G.
- A. Sahu and D. Kumar, Core-shell quantum dots: A review on classification, materials, application, and theoretical modeling, J. Alloys Compd., 2022, 924, 166508, DOI:10.1016/j.jallcom.2022.166508.
- E. Oh, R. Liu, A. Nel, K. B. Gemill, M. Bilal, Y. Cohen and I. L. Medintz, Meta-analysis of cellular toxicity for cadmium-containing quantum dots, Nat. Nanotechnol., 2016, 11(5), 479–486, DOI:10.1038/nnano.2015.338.
- M. Leu, P. Campbell and A.-V. Mudring, Synthesis of luminescent semiconductor nanoparticles in ionic liquids – the importance of the ionic liquid in the formation of quantum dots, Green Chem. Lett. Rev., 2021, 14(1), 128–136, DOI:10.1080/17518253.2021.1875057.
- J. F. Silva, J. Maria de Oliveira, W. F. Silva, A. C. Costa Soares, U. Rocha, N. Oliveira Dantas, E. Alves da Silva Filho, M. Duzzioni, A. Helmut Rulf Cofré and O. Wagner de Castro, et al., Supersensitive nanothermometer based on CdSe/CdSxSe1−x magic-sized quantum dots with in vivo low toxicity, Chem. Eng. Sci., 2022, 264, 118153, DOI:10.1016/j.ces.2022.118153.
- C. Y. Lee, N. Naik Mude, R. Lampande, K. J. Eun, J. E. Yeom, H. S. Choi, S. H. Sohn, J. M. Yoo and J. H. Kwon, Efficient Cadmium-Free Inverted Red Quantum Dot Light-Emitting Diodes, ACS Appl. Mater. Interfaces, 2019, 11(40), 36917–36924, DOI:10.1021/acsami.9b12514.
- G. Huang, Glyconanoparticles-an update, Curr. Med. Chem., 2013, 20(6), 782–788 CAS.
- F. Yang, G. Gao, J. Wang, R. Chen, W. Zhu, L. Wang, Z. Ma, Z. Luo and T. Sun, Chiral β-HgS quantum dots: Aqueous synthesis, optical properties and cytocompatibility, J. Colloid Interface Sci., 2019, 537, 422–430, DOI:10.1016/j.jcis.2018.11.057.
- G. Xu, S. Zeng, B. Zhang, M. T. Swihart, K.-T. Yong and P. N. Prasad, New Generation Cadmium-Free Quantum Dots for Biophotonics and Nanomedicine, Chem. Rev., 2016, 116(19), 12234–12327, DOI:10.1021/acs.chemrev.6b00290.
- Y. Han, X. Ding, J. Han, Y. Fang, Z. Jin, W. Kong and C. Liu, Oxygen-regulated carbon quantum dots as an efficient metal-free electrocatalyst for nitrogen reduction, Nanoscale, 2022, 14(27), 9893–9899, 10.1039/D2NR01551J.
- Q. Li, P. Shen, Y. Tian, X. Li and K. Chu, Metal-free BN quantum dots/graphitic C3N4 heterostructure for nitrogen reduction reaction, J. Colloid Interface Sci., 2022, 606, 204–212, DOI:10.1016/j.jcis.2021.08.032.
- K. Singh and S. K. Mehta, Luminescent ZnO quantum dots as an efficient sensor for free chlorine detection in water, Analyst, 2016, 141(8), 2487–2492, 10.1039/C5AN02599K.
- C. Xiang, L. Wu, Z. Lu, M. Li, Y. Wen, Y. Yang, W. Liu, T. Zhang, W. Cao and S.-W. Tsang, et al., High efficiency and stability of ink-jet printed quantum dot light emitting diodes, Nat. Commun., 2020, 11(1), 1646, DOI:10.1038/s41467-020-15481-9.
- Z. Zhang, Y. Miao, Q. Zhang and G. Yan, Facile and sensitive detection of protamine by enhanced room-temperature phosphorescence of Mn-doped ZnS quantum dots, Anal. Biochem., 2015, 478, 90–95, DOI:10.1016/j.ab.2015.03.010.
- G. Huang, F. Cheng, X. Chen, D. Peng, X. Hu and G. Liang, Recent progress on the applications of multifunctional glyconanoparticles, Curr. Pharm. Des., 2013, 19(13), 2454–2458 CrossRef CAS PubMed.
- G. Yang, J. Zhao, S. Yi, X. Wan and J. Tang, Biodegradable and photostable Nb2C MXene quantum dots as promising nanofluorophores for metal ions sensing and fluorescence imaging, Sens. Actuators, B, 2020, 309, 127735, DOI:10.1016/j.snb.2020.127735.
- C. G. Sanjayan, M. S. Jyothi and R. G. Balakrishna, Stabilization of CsPbBr3 quantum dots for photocatalysis, imaging and optical sensing in water and biological medium: a review, J. Mater. Chem. C, 2022, 10(18), 6935–6956, 10.1039/D2TC00340F.
- M. B. Gongalsky, L. A. Osminkina, A. Pereira, A. A. Manankov, A. A. Fedorenko, A. N. Vasiliev, V. V. Solovyev, A. A. Kudryavtsev, M. Sentis and A. V. Kabashin, et al., Laser-synthesized oxide-passivated bright Si quantum dots for bioimaging, Sci. Rep., 2016, 6(1), 24732, DOI:10.1038/srep24732.
- X. Niu, H. Chen, Y. Wang, W. Wang, X. Sun and L. Chen, Upconversion Fluorescence-SERS Dual-Mode Tags for Cellular and In Vivo Imaging, ACS Appl. Mater. Interfaces, 2014, 6(7), 5152–5160, DOI:10.1021/am500411m.
- P. Le, R. Vaidya, L. D. Smith, Z. Han, M. U. Zahid, J. Winter, S. Sarkar, H. J. Chung, P. Perez-Pinera and P. R. Selvin, et al., Optimizing Quantum Dot Probe Size for Single-Receptor Imaging, ACS Nano, 2020, 14(7), 8343–8358, DOI:10.1021/acsnano.0c02390.
- X. Liu, Y. Liu, S. Xu, C. Geng, Y. Xie, Z.-H. Zhang, Y. Zhang and W. Bi, Formation of “Steady Size” State for Accurate Size Control of CdSe and CdS Quantum Dots, J. Phys. Chem. Lett., 2017, 8(15), 3576–3580, DOI:10.1021/acs.jpclett.7b01238.
- B. Zhang, Y. Wang, C. Yang, S. Hu, Y. Gao, Y. Zhang, Y. Wang, H. V. Demir, L. Liu and K.-T. Yong, The composition effect on the optical properties of aqueous synthesized Cu–In–S and Zn–Cu–In–S quantum dot nanocrystals, Phys. Chem. Chem. Phys., 2015, 17(38), 25133–25141, 10.1039/C5CP03312H.
- H. Cao, J. Ma, L. Huang, H. Qin, R. Meng, Y. Li and X. Peng, Design and Synthesis of Antiblinking and Antibleaching Quantum Dots in Multiple Colors via Wave Function Confinement, J. Am. Chem. Soc., 2016, 138(48), 15727–15735, DOI:10.1021/jacs.6b10102.
- J. Pan, Z. Zheng, J. Yang, Y. Wu, F. Lu, Y. Chen and W. Gao, A novel and sensitive fluorescence sensor for glutathione detection by controlling the surface passivation degree of carbon quantum dots, Talanta, 2017, 166, 1–7, DOI:10.1016/j.talanta.2017.01.033.
- D. Thomas, H. O. Lee, K. C. Santiago, M. Pelzer, A. Kuti, L. J. Treadwell and M. Bahoura, Europium doping of cadmium selenide (CdSe) quantum dots via rapid microwave synthesis for optoelectronic applications, Dalton Trans., 2022, 51(1), 264–273, 10.1039/D1DT02920G.
- S. Cheng, J. Zhang, Y. Liu, Y. Wang, Y. Xiao and Y. Zhang, High quantum yield nitrogen and boron co-doped carbon dots for sensing Ag+, biological imaging and fluorescent inks, Anal. Methods, 2021, 13(45), 5523–5531, 10.1039/D1AY01582F.
- O. A. Goryacheva, A. S. Novikova, D. D. Drozd, P. S. Pidenko, T. S. Ponomaryeva, A. A. Bakal, P. K. Mishra, N. V. Beloglazova and I. Y. Goryacheva, Water-dispersed luminescent quantum dots for miRNA detection, TrAC, Trends Anal. Chem., 2019, 111, 197–205, DOI:10.1016/j.trac.2018.12.022.
- M. C. Biswas, M. T. Islam, P. K. Nandy and M. M. Hossain, Graphene Quantum Dots (GQDs) for Bioimaging and Drug Delivery Applications: A Review, ACS Mater. Lett., 2021, 3(6), 889–911, DOI:10.1021/acsmaterialslett.0c00550.
- X. Huang, J. Zhu, C. Dong, Y. Li, Q. Yu, X. Wang, Z. Chen, J. Li, Y. Yang and H. Wang, Polyvalent Aptamer-Functionalized NIR-II Quantum Dots for Targeted Theranostics in High PD-L1-Expressing Tumors, ACS Appl. Mater. Interfaces, 2024, 16(17), 21571–21581, DOI:10.1021/acsami.4c01486.
- P. Zhang, Y. Wang, X. Liu, L. Yuan, J. Liu, R. Guo and Y. Tian, Carboxyl-Modified Quantum Dots for NIR-IIb Bone Marrow Imaging, ACS Appl. Mater. Interfaces, 2024, 16(7), 8509–8517, DOI:10.1021/acsami.3c18282.
- E. S. Shibu, M. Hamada, S. Nakanishi, S.-i. Wakida and V. Biju, Photoluminescence of CdSe and CdSe/ZnS quantum dots: Modifications for making the invisible visible at ensemble and single-molecule levels, Coord. Chem. Rev., 2014, 263–264, 2–12, DOI:10.1016/j.ccr.2013.10.014.
- L. Cui, X. Ren, J. Wang and M. Sun, Synthesis of homogeneous carbon quantum dots by ultrafast dual-beam pulsed laser ablation for bioimaging, Mater. Today Nano, 2020, 12, 100091, DOI:10.1016/j.mtnano.2020.100091.
- W.-S. Kuo, X.-C. Shen, C.-Y. Chang, H.-F. Kao, S.-H. Lin, J.-Y. Wang and P.-C. Wu, Multiplexed Graphene Quantum Dots with Excitation-Wavelength-Independent Photoluminescence, as Two-Photon Probes, and in Ultraviolet-Near Infrared Bioimaging, ACS Nano, 2020, 14(9), 11502–11509, DOI:10.1021/acsnano.0c03915.
- B. Purushothaman and J. M. Song, Ag2S quantum dot theragnostics, Biomater. Sci., 2021, 9(1), 51–69, 10.1039/D0BM01576H.
- P. Linkov, P. Samokhvalov, S. Grokhovsky, M. Laronze-Cochard, J. Sapi and I. Nabiev, Selection of the Optimal Chromatography Medium for Purification of Quantum Dots and Their Bioconjugates, Chem. Mater., 2020, 32(21), 9078–9089, DOI:10.1021/acs.chemmater.0c03195.
- J. Sobhanan, J. V. Rival, A. Anas, E. Sidharth Shibu, Y. Takano and V. Biju, Luminescent quantum dots: Synthesis, optical properties, bioimaging and toxicity, Adv. Drug Delivery Rev., 2023, 197, 114830, DOI:10.1016/j.addr.2023.114830.
- V. Biju, T. Itoh and M. Ishikawa, Delivering quantum dots to cells: bioconjugated quantum dots for targeted and nonspecific extracellular and intracellular imaging, Chem. Soc. Rev., 2010, 39(8), 3031–3056, 10.1039/B926512K.
- Q. Dai, Z. Du, L. Jing, R. Zhang and W. Tang, Enzyme-Responsive Modular Peptides Enhance Tumor Penetration of Quantum Dots via Charge Reversal Strategy, ACS Appl. Mater. Interfaces, 2024, 16(5), 6208–6220, DOI:10.1021/acsami.3c11500.
- M. Tasso, E. Giovanelli, D. Zala, S. Bouccara, A. Fragola, M. Hanafi, Z. Lenkei, T. Pons and N. Lequeux, Sulfobetaine–Vinylimidazole Block Copolymers: A Robust Quantum Dot Surface Chemistry Expanding Bioimaging's Horizons, ACS Nano, 2015, 9(11), 11479–11489, DOI:10.1021/acsnano.5b05705.
- F. Erogbogbo, C.-W. Chang, J. L. May, L. Liu, R. Kumar, W.-C. Law, H. Ding, K. T. Yong, I. Roy and M. Sheshadri, et al., Bioconjugation of luminescent silicon quantum dots to gadolinium ions for bioimaging applications, Nanoscale, 2012, 4(17), 5483–5489, 10.1039/C2NR31002C.
- Y. Wang and L. Chen, Quantum dots, lighting up the research and development of nanomedicine, Nanomed. Nanotechnol. Biol. Med., 2011, 7(4), 385–402, DOI:10.1016/j.nano.2010.12.006.
- N. Le and K. Kim, Current Advances in the Biomedical Applications of Quantum Dots: Promises and Challenges, Int. J. Mol. Sci., 2023, 24(16), 12682 CrossRef CAS PubMed.
- G. Huang, X. Chen and F. Xiao, New fabrication and applications of carbohydrate arrays, Curr. Med. Chem., 2014, 21(3), 288–295 CrossRef CAS PubMed.
- J. Kim, S. Lee, Y. K. Lee, B. Seong, H.-M. Kim, S. Kyeong, W. Kim, K. Ham, X.-H. Pham and E. Hahm, et al., In Vitro Tracking of Human Umbilical Vein Endothelial Cells Using Ultra-Sensitive Quantum Dot-Embedded Silica Nanoparticles, Int. J. Mol. Sci., 2023, 24(6), 5794 CrossRef CAS PubMed.
- D. Jin, P. Xi, B. Wang, L. Zhang, J. Enderlein and A. M. van Oijen, Nanoparticles for super-resolution microscopy and single-molecule tracking, Nat. Methods, 2018, 15(6), 415–423, DOI:10.1038/s41592-018-0012-4.
- D. M. Bailey, O. Kovtun and S. J. Rosenthal, Antibody-Conjugated Single Quantum Dot Tracking of Membrane Neurotransmitter Transporters in Primary Neuronal Cultures, in Biomedical Nanotechnology: Methods and Protocols, ed. S. H. Petrosko and E. S. Day, Springer, New York, 2017, pp. 165–177 Search PubMed.
- T. D. Nguyen, Y.-I. Chen, L. H. Chen and H.-C. Yeh, Recent Advances in Single-Molecule Tracking and Imaging Techniques, Annu. Rev. Anal. Chem., 2023, 16(1), 253–284, DOI:10.1146/annurev-anchem-091922-073057.
- M. Rosendale, J. Flores, C. Paviolo, P. Pagano, J. Daniel, J. Ferreira, J.-B. Verlhac, L. Groc, L. Cognet and M. Blanchard-Desce, A Bottom-Up Approach to Red-Emitting Molecular-Based Nanoparticles with Natural Stealth Properties and their Use for Single-Particle Tracking Deep in Brain Tissue, Adv. Mater., 2021, 33(22), 2006644, DOI:10.1002/adma.202006644.
- J. Kang, A. Tahir, H. Wang and J. Chang, Applications of nanotechnology in virus detection, tracking, and infection mechanisms, Wiley Interdiscip. Rev.: Nanomed. Nanobiotechnol., 2021, 13(4), e1700, DOI:10.1002/wnan.1700.
- J. Lee, X. Feng, O. Chen, M. G. Bawendi and J. Huang, Stable, small, specific, low-valency quantum dots for single-molecule imaging, Nanoscale, 2018, 10(9), 4406–4414, 10.1039/C7NR08673C.
- M. Dahan, S. Lévi, C. Luccardini, P. Rostaing, B. Riveau and A. Triller, Diffusion Dynamics of Glycine Receptors Revealed by Single-Quantum Dot Tracking, Science, 2003, 302(5644), 442–445, DOI:10.1126/science.1088525.
- P. J. Cutler, M. D. Malik, S. Liu, J. M. Byars, D. S. Lidke and K. A. Lidke, Multi-Color Quantum Dot Tracking Using a High-Speed Hyperspectral Line-Scanning Microscope, PLoS One, 2013, 8(5), e64320, DOI:10.1371/journal.pone.0064320.
- B. Biermann, S. Sokoll, J. Klueva, M. Missler, J. S. Wiegert, J. B. Sibarita and M. Heine, Imaging of molecular surface dynamics in brain slices using single-particle tracking, Nat. Commun., 2014, 5(1), 3024, DOI:10.1038/ncomms4024.
- J. A. Varela, J. P. Dupuis, L. Etchepare, A. Espana, L. Cognet and L. Groc, Targeting neurotransmitter receptors with nanoparticles in vivo allows single-molecule tracking in acute brain slices, Nat. Commun., 2016, 7(1), 10947, DOI:10.1038/ncomms10947.
- L. Holtzer, T. Meckel and T. Schmidt, Nanometric three-dimensional tracking of individual quantum dots in cells, Appl. Phys. Lett., 2007, 90(5), 539021–539023, DOI:10.1063/1.2437066.
- L.-J. Zhang, S. Wang, L. Xia, C. Lv, H.-W. Tang, Z. Liang, G. Xiao and D.-W. Pang, Lipid-Specific Labeling of Enveloped Viruses with Quantum Dots for Single-Virus Tracking, mBio, 2020, 11(3), e00135, DOI:10.1128/mbio.00135-00120.
- C.-Y. Han, H.-S. Kim and H. Yang, Quantum Dots and Applications, Materials, 2020, 13(4), 897 CrossRef CAS PubMed.
- J.-Y. Zhao, G. Chen, Y.-P. Gu, R. Cui, Z.-L. Zhang, Z.-L. Yu, B. Tang, Y.-F. Zhao and D.-W. Pang, Ultrasmall Magnetically Engineered Ag2Se Quantum Dots for Instant Efficient Labeling and Whole-Body High-Resolution Multimodal Real-Time Tracking of Cell-Derived Microvesicles, J. Am. Chem. Soc., 2016, 138(6), 1893–1903, DOI:10.1021/jacs.5b10340.
- Q. Wang, H. He, Q. Zhang, Z. Feng, J. Li, X. Chen, L. Liu, X. Wang, B. Ge and D. Yu, et al., Deep-Learning-Assisted Single-Molecule Tracking on a Live Cell Membrane, Anal. Chem., 2021, 93(25), 8810–8816, DOI:10.1021/acs.analchem.1c00547.
- M. Majood, P. Garg, R. Chaurasia, A. Agarwal, S. Mohanty and M. Mukherjee, Carbon Quantum Dots for Stem Cell Imaging and Deciding the Fate of Stem Cell Differentiation, ACS Omega, 2022, 7(33), 28685–28693, DOI:10.1021/acsomega.2c03285.
- G. Aizik, N. Waiskopf, M. Agbaria, Y. Levi-Kalisman, U. Banin and G. Golomb, Delivery of Liposomal Quantum Dots via Monocytes for Imaging of Inflamed Tissue, ACS Nano, 2017, 11(3), 3038–3051, DOI:10.1021/acsnano.7b00016.
- H. Deng, C. J. Konopka, S. Prabhu, S. Sarkar, N. G. Medina, M. Fayyaz, O. H. Arogundade, H. E. Vidana Gamage, S. H. Shahoei and D. Nall, et al., Dextran-Mimetic Quantum Dots for Multimodal Macrophage Imaging In Vivo, Ex Vivo, and In Situ, ACS Nano, 2022, 16(2), 1999–2012, DOI:10.1021/acsnano.1c07010.
- P. Zrazhevskiy, L. D. True and X. Gao, Multicolor multicycle molecular profiling with quantum dots for single-cell analysis, Nat. Protoc., 2013, 8(10), 1852–1869, DOI:10.1038/nprot.2013.112.
- K. D. Wegner and N. Hildebrandt, Quantum dots: bright and versatile in vitro and in vivo fluorescence imaging biosensors, Chem. Soc. Rev., 2015, 44(14), 4792–4834, 10.1039/C4CS00532E.
- L. Wang, W. Li, M. Li, Q. Su, Z. Li, D. Pan and M. Wu, Ultrastable Amine, Sulfo Cofunctionalized Graphene Quantum Dots with High Two-Photon Fluorescence for Cellular Imaging, ACS Sustain. Chem. Eng., 2018, 6(4), 4711–4716, DOI:10.1021/acssuschemeng.7b03797.
- X. Cai, B. Wang, L. Nian, S. Zhao and J. Xiao, A robust and
versatile host–guest peptide toolbox for developing highly stable and specific quantum dot-based peptide probes for imaging extracellular matrices and cells, J. Mater. Chem. B, 2024, 12(4), 1031–1042, 10.1039/D3TB02749J.
- M. Asha Krishnan, K. Yadav, P. Roach and V. Chelvam, A targeted near-infrared nanoprobe for deep-tissue penetration and imaging of prostate cancer, Biomater. Sci., 2021, 9(6), 2295–2312, 10.1039/D0BM01970D.
- X. Michalet, F. F. Pinaud, L. A. Bentolila, J. M. Tsay, S. Doose, J. J. Li, G. Sundaresan, A. M. Wu, S. S. Gambhir and S. Weiss, Quantum Dots for Live Cells, in Vivo Imaging, and Diagnostics, Science, 2005, 307(5709), 538–544, DOI:10.1126/science.1104274.
- E. Petryayeva, W. R. Algar and I. L. Medintz, Quantum Dots in Bioanalysis: A Review of Applications across Various Platforms for Fluorescence Spectroscopy and Imaging, Appl. Spectrosc., 2013, 67(3), 215–252, DOI:10.1366/12-06948.
- X. Gao, Y. Cui, R. M. Levenson, L. W. K. Chung and S. Nie, In vivo cancer targeting and imaging with semiconductor quantum dots, Nat. Biotechnol., 2004, 22(8), 969–976, DOI:10.1038/nbt994.
- X. Guan, L. Zhang, S. Lai, J. Zhang, J. Wei, K. Wang, W. Zhang, C. Li, J. Tong and Z. Lei, Green synthesis of glyco-CuInS2 QDs with visible/NIR dual emission for 3D multicellular tumor spheroid and in vivo imaging, J. Nanobiotechnol., 2023, 21(1), 118, DOI:10.1186/s12951-023-01859-6.
- M. Pourmadadi, E. Rahmani, M. Rajabzadeh-Khosroshahi, A. Samadi, R. Behzadmehr, A. Rahdar and L. F. R. Ferreira, Properties and application of carbon quantum dots (CQDs) in biosensors for disease detection: A comprehensive review, J. Drug Delivery Sci. Technol., 2023, 80, 104156, DOI:10.1016/j.jddst.2023.104156.
- X. Sun, M. Shi, C. Zhang, J. Yuan, M. Yin, S. Du, S. Yu, B. Ouyang, F. Xue and S.-T. Yang, Fluorescent Ag–In–S/ZnS Quantum Dots for Tumor Drainage Lymph Node Imaging In Vivo, ACS Appl. Nano Mater., 2021, 4(2), 1029–1037, DOI:10.1021/acsanm.0c02542.
- X.-L. Ge, B. Huang, Z.-L. Zhang, X. Liu, M. He, Z. Yu, B. Hu, R. Cui, X.-J. Liang and D.-W. Pang, Glucose-functionalized near-infrared Ag2Se quantum dots with renal excretion ability for long-term in vivo tumor imaging, J. Mater. Chem. B, 2019, 7(38), 5782–5788, 10.1039/C9TB01112A.
- J. Wang, Y. Wang, J. Jia, C. Liu, D. Ni, L. Sun and Z. Guo, Dual-Modality Molecular Imaging of Tumor via Quantum Dots-Liposome–Microbubble Complexes, Pharmaceutics, 2022, 14(11), 2510 CrossRef CAS PubMed.
- Y. Kong, J. Chen, H. Fang, G. Heath, Y. Wo, W. Wang, Y. Li, Y. Guo, S. D. Evans and S. Chen, et al., Highly Fluorescent Ribonuclease-A-Encapsulated Lead Sulfide Quantum Dots for Ultrasensitive Fluorescence In Vivo Imaging in the Second Near-Infrared Window, Chem. Mater., 2016, 28(9), 3041–3050, DOI:10.1021/acs.chemmater.6b00208.
- H. Ren, Y. Yuan, A. Labidi, Q. Dong, K. Zhang, E. Lichtfouse, A. A. Allam, J. S. Ajarem and C. Wang, Green process of biomass waste derived fluorescent carbon quantum dots for biological imaging in vitro and in vivo, Chin. Chem. Lett., 2023, 34(6), 107998, DOI:10.1016/j.cclet.2022.107998.
- S. Feng, M. Chen, Y. Chen, L. Sai, S. Dong, H. Li, Y. Yang, J. Zhang, X. Yang and X. Xu, et al., Seeking and identifying time window of antibiotic treatment under in vivo guidance of PbS QDs clustered microspheres based NIR-II fluorescence imaging, Chem. Eng. J., 2023, 451, 138584, DOI:10.1016/j.cej.2022.138584.
- S. Wang, Y. Wen, Y. Wang, Y. Ma and Z. Liu, Pattern Recognition of Cells via Multiplexed Imaging with Monosaccharide-Imprinted Quantum Dots, Anal. Chem., 2017, 89(10), 5646–5652, DOI:10.1021/acs.analchem.7b00965.
- S. Xu, Z. Gu, H. Lu, P. Guan and Z. Liu, Leveraging Macrophage-Mediated Cancer Immunotherapy via a Cascading Effect Induced by a Molecularly Imprinted Nanocoordinator, ACS Appl. Mater. Interfaces, 2023, 15(23), 27658–27669, DOI:10.1021/acsami.3c03950.
- M. Panagiotopoulou, Y. Salinas, S. Beyazit, S. Kunath, L. Duma, E. Prost, A. G. Mayes, M. Resmini, B. Tse Sum Bui and K. Haupt, Molecularly Imprinted Polymer Coated Quantum Dots for Multiplexed Cell Targeting and Imaging, Angew. Chem., Int. Ed., 2016, 55(29), 8244–8248, DOI:10.1002/anie.201601122.
- S. Jeong, Y. Jung, S. Bok, Y.-M. Ryu, S. Lee, Y.-E. Kim, J. Song, M. Kim, S.-Y. Kim and G. O. Ahn, et al., Multiplexed In Vivo Imaging Using Size-Controlled Quantum Dots in the Second Near-Infrared Window, Adv. Healthcare Mater., 2018, 7(24), 1800695, DOI:10.1002/adhm.201800695.
- A. Zebibula, N. Alifu, L. Xia, C. Sun, X. Yu, D. Xue, L. Liu, G. Li and J. Qian, Ultrastable and Biocompatible NIR-II Quantum Dots for Functional Bioimaging, Adv. Funct. Mater., 2018, 28(9), 1703451, DOI:10.1002/adfm.201703451.
- Z. Sun, H. H. Xing, M. Qing, Y. Shi, Y. Ling, N. B. Li and H. Q. Luo, From the perspective of high-throughput recognition: Sulfur quantum dots-based multi-channel sensing platform for metal ions detection, Chem. Eng. J., 2023, 452, 139594, DOI:10.1016/j.cej.2022.139594.
- Y. Tang, K. Liu and H. Sun, Multi-channel Precision Bias Source for Experiments on Quantum dots, 2021, preprint, 2021070458, DOI:10.20944/preprints202107.0458.v2.
- V. K. Sagar, S. Dey, S. Bhattacharya, P. Lesani, Y. Ramaswamy, G. Singh, H. Zreiqat and P. B. Bisht, Probing heteroatoms co-doped graphene quantum dots for energy transfer and 2-photon assisted applications, J. Photochem. Photobiol., A, 2022, 423, 113618, DOI:10.1016/j.jphotochem.2021.113618.
- J. Chen, W. Han, Y. Zhang, X. Zhang, Y. Ge, Y. Guo and F. Yang, Bias-dependent hole transport through a multi-channel silicon nanowire transistor with single-acceptor-induced quantum dots, Nanoscale, 2022, 14(30), 11018–11027, 10.1039/D2NR02250H.
|
This journal is © The Royal Society of Chemistry 2024 |
Click here to see how this site uses Cookies. View our privacy policy here.