DOI:
10.1039/D4RA04441J
(Review Article)
RSC Adv., 2024,
14, 30201-30229
Metal–organic frameworks in drug delivery: engineering versatile platforms for therapeutic applications
Received
17th June 2024
, Accepted 30th August 2024
First published on 23rd September 2024
Abstract
Recently, metal–organic frameworks (MOFs) have attracted much attention as versatile materials for drug delivery and personalized medicine. MOFs are porous structures made up of metal ions coupled with organic ligands. This review highlights the synthesis techniques used to design MOFs with specific features such as surface area and pore size, and the drug encapsulation within MOFs not only improves their stability and solubility but also allows for controlled release kinetics, which improves therapeutic efficacy and minimizes adverse effects. Furthermore, it discusses the challenges and potential advantages of MOF-based drug delivery, such as MOF stability, biocompatibility, and scale-up production. With further advancements in MOF synthesis, functionalization techniques, and understanding of their interactions using biological systems, MOFs can have significant promise for expanding the area of personalized medicine and improving patient outcomes.
1. Introduction
The term drug delivery system refers to various formulations and processes used to provide drugs to patients.1 Controlling drug release was difficult until 1950, which resulted in rapid-release formulations in pills or capsules.2,3 The first generation was launched by releasing Spansule capsules, which carried hundreds of micropellets coated with a water-soluble wax of varying thicknesses, one for each micropellet.2,3 Thus, drug delivery systems, such as pH- or temperature-sensitive nanoparticles, were modified for use in targeted delivery applications in second-generation treatments, following a deeper understanding of first-generation drug delivery release mechanisms.2,3 The last ten years have primarily focused on4,5 developing targeted nanotechnology-based drug delivery systems (DDSs) for cancers and gene delivery using various biodegradable nanoparticles (NPs).2,3,6 The most recent era of controlled release technology is the third generation of drug delivery systems.4,5,7 To be effective and successful, it must overcome the physicochemical and biological challenges associated with prior drug delivery technologies.5
Metal–organic frameworks (MOFs), or porous coordination polymers, have been intensively explored for gas storage, catalysis, sensors, water treatment, and membranes, as shown in Fig. 1.8,9 MOFs have demonstrated effectiveness in the delivery of drugs by acting as transporters of nucleic acids, proteins, and tiny molecules. These hybrid materials are porous and tuneable, with an extensive surface area, a high degree of porosity, and remarkable chemical stability.10,11 MOFs have the unique capacity to modify their drug release profiles and interact with biological systems based on target-specific tissues or cells, making them essential to improving therapeutic results.11,12 The structure of MOFs enables surface modification for tailored administration, maximizing efficacy and minimizing undesirable effects.10,12,13
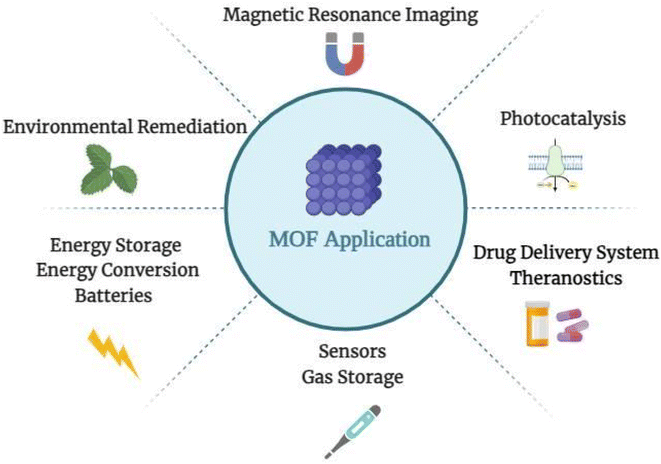 |
| Fig. 1 MOF-based materials as smart platforms for various applications. | |
The most extensively studied area in drug delivery research is MOFs, with most publications focusing on these materials. There has been a steady and rapid increase in interest, with the number of publications increasing from 11 in 2010 to 618 in 2023, as shown in Fig. 2.
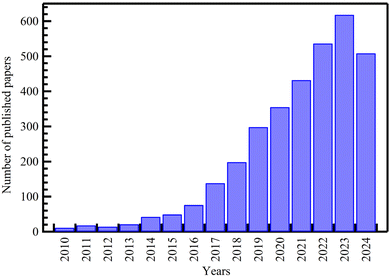 |
| Fig. 2 Distribution of the cumulative number of publications on MOFs as drug delivery systems per year based on the Scopus database (as of August 2024) using a combination of the keywords “metal–organic frameworks” and “drug delivery” in the title, abstract, or keyword fields. | |
Overall, MOFs have emerged as a smart approach to drug delivery systems due to their unique properties. These materials are at the forefront of pharmaceutical innovation because of their ability to encapsulate, protect, and precisely release medications, and their potential for surface functionalization and combination therapies. Despite these promising prospects, it is important to emphasize that the release kinetics of MOFs in targeted drug delivery are still under active investigation and require further study. This work focuses on the recent advances of MOFs as a potential smart nano-carrier for drug delivery system designs; furthermore, it addresses the current techniques for MOF functionalization, such as traditional methods, electrochemical synthesis, and anodic dissolution, illustrating how MOFs can revolutionize drug delivery systems by enhancing targeting therapy for various types of disease and improving patient outcomes.
2. Fundamentals of metal–organic frameworks
2.1 Structure, composition, and classification of MOFs
MOFs are crystalline, extremely porous materials that combine organic ligands with metal ions or clusters held together by coordinative bonding. This huge family is becoming increasingly attractive for drug delivery due to its easily functionalized surfaces, tunable porosity, chemical composition, size, and form. Designing tailored MOFs with regulated sizes for various biomedical purposes has gained increasing interest in recent decades. It is widely understood that forming porous MOFs requires metal ions as metal nodes and polydentate organic ligands as coordinating linkers. Moreover, porous MOFs offer significant advantages over traditional zeolites and carbon-based materials due to their exceptionally high surface area and diverse pore sizes. Metal ions or their coordination clusters, with well-defined coordination geometries, are promising candidates for creating highly porous MOFs and frameworks with unique flexibility.14 In metal complexes, the molecules are dissociated from one another. In contrast, MOFs consist of a structurally stable arrangement of repeating units that forms a supramolecular chain. Metal complexes are typically formed using ligands with a single bonding site such as –O or –N. However, MOFs are synthesized using chelating ligands with multiple bonding sites. As a kind of highly organized crystalline porous coordination polymer, MOFs show great promise. A large surface area, crystalline structure with a high density of active sites, and excellent stability can be achieved by designing, tailoring, and modifying the structural characteristics of MOFs, including metal clusters, organic linkers, and pore diameters.15 In the last ten years, MOFs have been more widely used for drug delivery, biocompatibility, and biodegradability due to their desirable characteristics, such as their harmless effects, guided and stimulus-based delivery systems, continuous release, and various drug-loaded properties. Scientists are always looking for new ways to learn more about MOFs and their remarkable properties.16 One of these is their ability to interact with biological systems in response to different stimuli. This includes improving the solubility of amorphous and poorly soluble drugs in a wide range of conditions, including changes in pH, temperature, light, magnetic fields, pressure, glucose levels, and response to multiple stimuli. Encapsulating macromolecular cargos (such as proteins and nucleic acids) in MOFs stabilizes their structures and protects them from degradation in biological settings. Investigations have also been conducted into even larger materials, such as cells and vaccinations.9 MOFs are a rapidly expanding class of porous materials that, owing to their many desirable properties, have piqued the curiosity of scientists globally. Porous materials are increasingly popular due to their high pore volume, customizable pore size, and large surface area. They are used in various fields, such as biomedicine, chemistry, insulation, sensors, and gas storage.17 The combination of MOF's large specific surface area, outstanding physical and chemical characteristics, and high porosity makes it an attractive and practical material for filtering polluted water from heavy metal ions.13 Two primary criteria for classifying porous materials are building framework composition and pore size. For materials, there are typically three categories of pore size: macropores (>50 nm; examples include sponge and cotton), mesopores (2–50 nm; examples include MCM-41, SBA-14, and mesoporous silica), and micropores (<2 nm; examples include zeolite and MOFs), as illustrated in Fig. 3. In contrast, there are organic polymers (POPs), hybrid organic, and pure inorganic materials (such as silica) that can be used to construct frameworks. MOFs, primarily used for drug delivery systems, are organized using various linkers and metals, resulting in unique polytropic structures and enhanced biodegradability. The balance between biodegradability and stability in biological mediums is crucial. MOFs can respond to drug delivery stimuli, pH changes, molecules, heat, and pressure. They are categorized based on specific formulation components and properties.18 Composition, structure, and applications are some of the factors that can be used to classify MOFs.
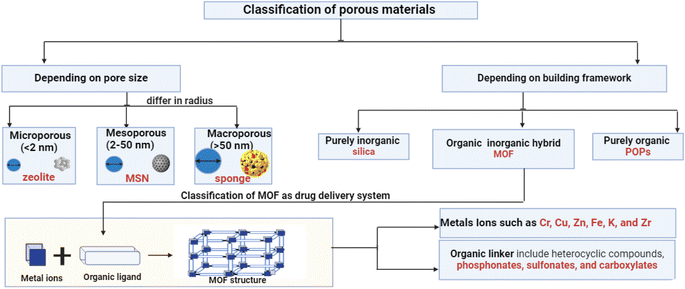 |
| Fig. 3 Classification of porous material and MOFs. | |
2.1.1 Classification of MOFs by metal ions. Metal ions and organic linkers have been used to create thousands of MOFs, which are crucial for their biocompatibility and toxicity in DDSs. The median lethal dose (LD50) is used to determine a metal's toxicity. Metals such as potassium, zinc, zirconium, and iron are suggested for use in DDSs with MOFs, with oral LD50 values of 0.215, 0.35, 4.1, and 0.45 g kg−1, respectively. MOFs have been used as anticancer, antibiotic, and antiviral medicines and can be co-loaded with medications for synergies. MOFs offer benefits, such as higher bioavailability, stability, targeting capabilities, and solubility.19
2.1.1.1 Cr-based MOFs. In 2006, two model systems, MIL-100 (Cr) and MIL-101 (Cr), were used to evaluate drug loading into MOFs. In MIL-101 (Cr), Cr ions form coordination bonds with either 1,4-benzenedicarboxylic acid (BDC) or terephthalic acid, while MIL-100 (Cr) binds Cr ions to 1,3,5-benzenetricarboxylic acid (BTC) or trimesic acid. Ibuprofen (IBU) showed a high drug loading capacity, with a loading concentration of 1.4 g per gram of dehydrated MIL-101 (Cr).19
2.1.1.2 Cu-based MOFs. Cu-MOFs based on copper offer strong binding sites for guest materials due to their easily accessible, coordinatively unsaturated metal sites. He et al. used a hydrothermal approach to manufacture mixed ligands, MOFs-2 and MOFs-3, which are harmless to normal human cells and HEK 293A cells. Multi-ligand MOFs have shown superior drug delivery capabilities compared to MOFs with only a single ligand.19 Hou et al. developed a smartphone-based method for the visual detection of alkaline phosphatase using amino-functionalized Cu-MOFs, which have fluorescence properties and could be used for alkaline phosphatase detection in serum samples.19,20 Cu-MOFs also have potential use in treating microorganisms, with their antibacterial properties against various bacterial types even at low concentrations.19
2.1.1.3 K-based MOFs. CD-MOFs made from cyclodextrin, ethanol, and potassium ions are edible and highly porous MOFs with a high surface area. They are widely used in the biomedical industry due to their porous nature, water-solubleness, and non-toxic properties. CD-MOFs have been loaded with pharmaceuticals using methods such as impregnation, grinding, and co-crystallization. For example, lansoprazole was loaded into CD-MOFs using an improved co-crystallization technique, achieving a drug loading of 23.2%. CD-MOFs can significantly improve the solubility and bioavailability of insoluble medicines, such as azilsartan, in Sprague-Dawley rats. They have a long history of use in pulmonary, intravenous, and oral DDSs.19,21
2.1.1.4 Fe-based MOFs. MIL-53 (Fe) iron-based MOFs (Fe-MOFs) were developed for biomedicine due to their low toxicity, design flexibility, and biodegradability. These nanosized MOFs were used for loading anti-tumor or retroviral medications, with the first nanosized Fe-MOFs showing degradability, biosafety, and imaging capabilities. Leng et al. used MIL-53 to load the anti-cancer medicine oridonin onto Fe-MOFs, with a drug loading capacity of 56.25 w/w and a sustained release period of over seven days.22 The MOFs also demonstrated exceptional magnetic resonance imaging (MRI) performance due to the presence of Fe(III) ions. Folic acid (FA) and fluorescent reagent modifications allowed for tailored medication delivery and fluorescence imaging. MIL-100 (Fe) was used to co-encapsulate azidothymidine triphosphate (AZT-TP) and lamivudine triphosphate, enhancing the effectiveness of anti-human immunodeficiency virus (HIV) treatments.23
2.1.1.5 Zr-based MOFs. Cavka et al. (2008) discovered that zirconium-based MOFs (Zr-MOFs), mainly Zr(IV) carboxylates, have exceptional stability, especially hydrothermal stability, due to their extreme oxidation state and strong coordination interactions.24 Zr-MOFs are considered an appropriate biomedical material due to their low toxicity in living organisms and widespread distribution in nature. A study found that cancer cells might be subjected to enhanced cytotoxicity in vitro when dichloroacetate and 5-fluorouracil (5-FU) were delivered synergistically from Zr-MOFs.19,25 Another study investigated variations in drug loading capacity and release behavior by introducing UiO-66 with –NH2 and –NO2 functional groups. Zr-fum, another Zr-MOF, incorporates an endogenous fumarate linker and is stable in water. It is more effective in transporting the drug imitator calcein into HeLa cells and has improved biocompatibility due to its endogenous fumarate linker.19,26
2.1.1.6 Zn-based MOFs. Rojas et al. (2016) developed zinc-based MOFs (Zn-MOFs) in the form of four zinc pyrazolate isoreticular MOFs, ZnBDP_X, for intravenous and oral administration.27 These MOFs have a particle size of less than 200 nm and exhibit strong structural and adhesive durability.28 Two types of anticancer drugs, mitoxantrone and Ru (p-cymene) Cl2 (1,3,5-triaza-7-phospaadamantane) (RAPTA-c), were encased in the pores of the ZnBDP_X compound family. Bag et al. improved the therapeutic activity and aqueous stability of Zn-MOFs by creating a strong bi-carboxylate ligand, 4,4′-(9-H carbazole-3,6-diyl) dibenzoic acid (H2CDDB).29 These porous MOFs demonstrated an outstanding loading ability of 53.3% (w/w) for 5-FU, exhibited biosafety after a 12 hours incubation period, and remained stable in water for up to three weeks. Zn-cpon-1, another Zn-MOF with a 3D topological framework, was created using ClO4− as a template and 5-(4′-carboxyphenoxy) nicotinic acid (H2cpon) as an organic linker. Its acid-responsive twofold stimulation activity makes it an excellent drug delivery vehicle, which can load up to 44.75 percent weight of 5-FU.19 Zeolitic imidazolate frameworks (ZIFs), a subfamily of Zn-MOFs linked by Zn(II) and imidazolate or its derivatives, have been used in numerous DDSs. Sun et al. used zeolitic imidazole framework-8 (ZIF-8) to pack the volatile and hydrophobic d-α-tocopherol succinate, achieving a drug loading ratio of 43.03% (w/w).19,30
2.1.2 Classification of MOFs by organic linker. MOFs are versatile materials with a wide range of organic linkers and constitutive metals, and they play a crucial role in their physicochemical characteristics and 3D supramolecular organization. Common linkers include heterocyclic compounds, phosphonates, sulfonates, and carboxylates, with approximately 50% of all materials produced being MOFs made up of carboxylate ligands. The choice of linker in MOFs significantly affects their stability, biodegradability, bioavailability, toxicity, and physical and chemical properties. The synthesis of MOFs can be customized by selecting the appropriate linkers, such as imidazole or polycarboxylic acid, due to their low toxicity and high polarity.19,31 Enzymatic synthesis of biocompatible iron carboxylates is also possible. Organic linkers and functional groups also influence drug payloads and release patterns in MOFs. BioMOFs, which are synthesized using active molecules as linkers, offer good biocompatibilities and high drug payloads due to their intrinsic self-assembly. Building blocks can be biomolecules, such as sugars, amino acids, or nucleobases. The biomedical uses of BioMOFs remain largely unexplored due to a lack of research into their stability in biological media. However, numerous studies have documented the use of active components in building BioMOFs, such as a substance with anti-lipid, vasodilatory, and pellagra-treating capabilities and therapeutically active vitamin B3.19 A hydrothermally produced 3D microporous MOF with a 4-connected two-nodal net has been employed to selectively absorb C2H2/CO2 mixes, displaying excellent reusability and great selectivity, even though these combinations have comparable physical properties.32
2.2 Synthesis techniques and structural tunability
MOFs may be designed with great stability, sensitivity, selectivity, and simplicity of assembly. They can also be made to exhibit adjustable colorimetric responses. The organic and inorganic building components of MOFs provide fresh insight into the development of porous materials. MOFs are highly recognized owing to their remarkable chemical stability, great thermal stability, ultrahigh porosity, and variable pore size, making them perfect for gas separation and storage. Because of the superior properties of MOFs over conventional semiconductors, MOFs are becoming increasingly acknowledged as photocatalysts. According to a study conducted by Wei et al., the photocatalytic reduction of 4-nitroaniline is strongly influenced by the spatial placement of CdS NPs in UiO-66 frameworks.33 For CdS NP encapsulation, microporous UiO-66 nanocages outperform their mesoporous and solid counterparts.34 Over the last twenty years, luminous MOFs have become more and more popular because of their huge payloads, adaptable surface chemistry, and configurable sizes. MOFs are combined with fluorescent medicines, fluorescent dyes, metal ions/clusters, photosensitizers, and luminescent NPs, such as quantum dots, persistent luminescent nanoparticles, and up-conversion NPs, to create these optical imaging agents.35
MOF nontoxic effects, drug-loaded properties, and continuous release have enriched its use in drug delivery, biocompatibility, and biodegradability. MOFs can interact with biological systems based on various stimuli, thereby improving drug release capacity and solubility under variable conditions. Scientists have continuously explored their properties.36 To create MOFs with the necessary size, surface topology, chemical content, and application area, synthesis procedures have been proposed. MOFs were originally synthesized using solvothermal synthesis, but new methods, such as mechanochemical, electrochemical, sonochemical, and microwave-assisted techniques, have been developed over the years.
2.2.1 Methods of traditional synthesis. MOFs are synthesized using traditional methods that can be solvothermal or non-solvothermal depending on the environment. Solvothermal synthesis involves heating solvents to their boiling point or higher in a sealed vessel. This can be performed using special closed chemical reactors or open flasks at atmospheric pressure. This results in milder thermal conditions and more homogeneous anemone structures. Non-solvent methods require metal precursors, organic linkers, and appropriate synthesis temperature. The latter is simple and does not require complicated reactions. The temperature used in these synthesis processes considerably affects their final output. A new MOF-199 coating was created in situ via solvothermal synthesis for the solid-phase microextraction of volatile organic molecules from air samples.37 The traditional synthesis of MOFs is well established. However, using alternative solvents instead of solvothermal solvents can impact the form and toxicity of the resulting materials. Optimizing the choice of solvent is crucial for maintaining synthesis quality. Additionally, polyvinylpyrrolidone modulators are used to control the sizes of the NPs.38
2.2.2 Synthesis of MOFs using microwaves. MW-assisted synthesis enhances MOF stability. MOF morphology varies based on synthesis method, reaction time, morphology, yield, and particle size. Covalent bonding affects MOF properties. Highly porous structures are important for adsorption, gas storage, catalysts, and electrical components. Future development of MOFs with augmented performance is possible.36 MW-assisted synthesis is a faster and more efficient method for synthesizing porous materials, such as MOFs. It reduces the time required for synthesis from days to minutes and avoids toxic solvents. The properties and functions of MOFs obtained by MW synthesis are highly improved. A solution can be heated under MW fields through conduction and polarization. The rotation of dipoles during polarization causes dielectric heating, while the collisions of atoms and molecules due to conduction generate ohmic heating. MW heating is widely used in synthetic organic and inorganic chemistry. The whole sample is uniformly heated, and the scaling-up procedure is less complicated. Cr-MIL-100 was the first MOF to be synthesized under MW. Amorphous products were formed under low reagent conditions, and crystalline products were obtained with modulators.36 Synthesizing MOFs using a microwave offers various advantages, such as enhanced efficiency, increased purity, and crystallinity; the ability to manage particle size and shape, reduced energy usage; and adherence to principles of green chemistry. Nevertheless, several drawbacks are associated with it, including problems with achieving uniform heating, limited capacity to scale up, compatibility challenges, high costs of equipment, and safety concerns. The dispersion of microwave radiation might result in localized overheating or uneven progress of a reaction. However, achieving consistent exposure across greater quantities can be difficult. Certain MOF precursors or initial substances may not be suitable for microwave synthesis conditions, and the expenses associated with equipment may be substantial. Safe operation requires adequate training and appropriate equipment.
2.2.3 Electrochemical synthesis of MOFs. Direct or indirect techniques can be used to synthesize and deposit MOFs on substrates. The necessary MOFs are produced by direct techniques, which entail electrochemical processes on the electrodes, such as reductive electrosynthesis and anodic dissolution. Certain techniques, such as electrophoretic deposition, self-templated synthesis, and galvanic displacement, are employed in indirect approaches. Direct approaches allow for real-time adjustment of electrochemical parameters and conditions. Real-time control over the synthesis process is the primary benefit.39 This review covers direct approaches.
2.2.3.1 Anodic dissolution. One prevalent electrochemical synthesis method for creating MOFs is anodic dissolution. It permits MOF properties to be independent of metal ion precursors. This is the most widely used technique for producing MOFs. The biggest advantage of anodic dissolution over other production techniques is that it frees the MOFs from the need for metal ion intermediates (e.g., Cu(NO3)2 vs. CuCl2).40 For instance, HKUST-1, a MOF with Cu2+-paddlewheel-type nodes, and BTC, produced by anodic dissolution, have double the surface area of the samples produced solvothermally. During the chemical production of MOFs, the counterions of the precursor block the pores. Four phases comprise the anodic electrosynthesis of MOFs: detachment, growth, intergrowth, and nucleation. At the electrode surface, metal ions are added to a solution that contains a linker. After that, the nuclei grow to form micrometric-sized crystals. Together, the nuclei grow to form crystal islands. During the detachment process, the electrode beneath the generated crystals dissolves even further. Important factors include the solvent, electrolyte content, applied voltage, electrodeposition duration, and spacing between the electrodes.41 Protic solvents are used in the anodic dissolution process to prevent metal ion electro-reduction at the cathode surface. According to Kumar et al., a high applied potential results in a high material synthesis yield.42
2.2.3.2 Reductive electrosynthesis. For the production of a probase agent at the cathode surface during the reductive electrosynthesis of MOFs, a solution including all starting ingredients, such as nitrate or perchlorate ions, is required.43 Reductive electrosynthesis can be used in conjunction with the anodic dissolution technique for MOFs containing readily reducible metals, as this approach is not appropriate for them. The procedure covers the electrode with a metal coating by applying cathodic potentials, reducing oxoanions to produce hydroxide ions, deprotonating the linker in an alkaline environment, and quickly interacting with metal ions. On the electrode surface, the MOF layer is mostly electrodeposited. Selecting the right probase agent is essential for preventing undesirable side effects and high applied potential values.To regulate the electrosynthesis conditions and prevent metal ion deposition on the substrate, triethylammonium, a less negative potential probase agent, was employed. For the reductive electrosynthesis of MOFs, other probase agents, such as zeolitic imidazolate structures, can be used. By choosing the appropriate reduction potentials, the electrochemical characteristics of the as-prepared MOF films could be readily adjusted. The Cu-BTC framework shape, electrocatalytic activity, electronic states of metal, and sensing capacity have all been effectively controlled by electrodeposited Cu-BTC thin films on fluorine-doped tin oxide at various cathodic potentials.44
A conductive item is deposited in a supporting electrolyte under a voltage gradient using the electrodeposition process known as bipolar electrosynthesis (BPES). Redox reactions can occur simultaneously using this approach, making it a practical technique for producing MOFs under control. Additionally, conductive organic single crystals may be used as bipolar electrodes owing to BPES. Unlike anodic dissolution and reductive electrosynthesis processes, MOF films are site-selectively produced on the substrate using the BPES approach.45
2.2.4 Mechanochemical synthesis. For mechanochemical processes, various commercially available containers and apparatus are utilized, such as drums, extruders, mixers, planetary balls, cry or mills, and mortar grinders and mills. A mortar and pestle are the most basic and affordable instruments, but because of the circumstances that can be repeated, it is not advisable to use them. Uniform fragmentation, dependable grinding procedures, and repeatable, contamination-free synthesis conditions are guaranteed by electronically controlled mortar grinders and mills. However, items made from materials used in mechanochemical reactors may include contaminants.46Over the past 20 years, mechanochemistry has seen tremendous change, with major advancements achieved in the fields of technique, apparatus, and processes. The primary emphasis has been on the synthesis of organic chemicals, resulting in the development of sophisticated nanomaterials with a wide range of uses. Nowadays, a vast variety of inorganic, organic, and hybrid compounds are included in the field of mechanochemistry. These include polymers, metal complexes, alloys, nanoparticles, carbonaceous materials, supramolecular complexes, and energy materials.47 One chemical technique that shows promise for creating MOFs with improved yield and high purity is mechanochemical synthesis. Coordination polymerization, which includes the reaction in the presence of metal ions, multisite ligands, and solutions, is the process used in this approach.47 Less solvent-intensive, solvent-free, or solid-state organic techniques without hazardous solvents are used in the mechanochemical production of MOFs. This method has the benefit of producing MOFs on a wide scale at room temperature with a shorter reaction time. The solid–solid interaction offers ease of handling and the ability to synthesize materials on a large scale. Although a solvent-based purification step is still necessary, mechanochemical synthesis is predicted to be a commercially interesting method for producing MOFs that are ecologically benign. The result of the liquid-assisted mechanochemical synthesis was MOFs-5, a 97% product yield of MOFs based on copper.47 The mechanochemical approach yields framework structures that are readily separated from the host molecules, allowing for reproducible free pore access for future applications.
2.2.5 Sonochemical synthesis. Chemical reactions are produced using ultrasound-induced cavitation in sonochemical synthesis.48 Pressure waves known as ultrasounds compress and expand gasses and particles in a reaction mixture, overstretching intermolecular forces and causing bubbles to form; this process is known as cavitation. These bubbles swiftly release stored energy when they burst, with accumulated energy of up to a threshold size. Extreme local pressure and temperature conditions are caused by these phenomena; hot spots can reach up to 1000 atm and 5300 °C, respectively.49 Ultrasounds do not directly interact with chemical species; instead, cavitation caused by ultrasounds provides the energy required for synthesis. Additionally, cavitation produces radicals, which are the starting point for chemical processes. Physical and mechanical processes, including microjet, shockwave, and heating, influence surface composition and morphologies, speed up mass transfer and produce nanostructures. Chemical syntheses depend on these processes because they enable the decomposition of layered materials into 2D-layered compounds.50 Ultrasonic baths, longitudinal horns, ultrasonic probes (horns), and numerous transducers are examples of sonochemical reactors that use water and reaction vessel walls to inject ultrasounds into a reacting system. Although ultrasonic probes deliver energy directly into the system, ultrasonic baths introduce energy through the walls of the reaction vessel and the water. For large-scale applications, longitudinal horns and transducers are preferred. A transducer is the fundamental component of sonochemical devices; it transforms mechanical or electrical energy into ultrasounds. Typical piezoelectric transducers may function in the entire ultrasonic frequency range; higher frequencies are appropriate for chemical effects, and lower frequencies are good for physical effects. These transducers are built using materials such as barium titanate or lead methaniobate. A few more crucial factors influencing sonochemical synthesis include solvent type, mass transfer, mixing time, and flow diagram. Solvents with low surface tension encourage bubble formation but lessen the degree of cavitation.51 A chemical reaction known as a mechanochemical reaction is introduced by the absorption of mechanical energy, usually by shearing, compression, ball- or pan-milling, grinding, or other similar processes. These processes result in surface alterations, flaws, and plastic and elastic deformations, which break chemical bonds and generate new ones. The physical and chemical effects of ultrasonically generated cavitation include localized high temperature and pressure, crystal deformation, shear stresses, accelerated diffusion processes, broken chemical bonds, and the production of very reactive radicals. Shock waves and other physical impacts produced by ultrasounds affect the response system mechanically.51
3. Design principles for drug delivery in MOFs
3.1 Surface modification and functionalization strategies
MOFs are three-dimensional polymeric structures made up of organic linkers and metal nodes coupled with one another.52 Highly ordered structures, large surface areas, chemical and thermal stability, sustained luminescence, tunable pore diameters, high crystallinity, and large pore volumes are just a few of the amazing qualities of MOFs.11,15,53,54 They can open channels, trap beneficial chemicals inside the framework, or absorb them on their exterior because of their large pore volume. Functional compounds can be added to MOFs by post-synthetic modification or one-pot synthesis forming covalent bonds.15 One common way to accomplish these functions is by attaching biocompatible polymeric structures or proteins.55 When used for drug delivery, the crystal surface of MOFs is functionalized to meet various requirements such as preventing agglomeration in the bloodstream or causing the delivery location (such as cancer cells) to be specifically recognized. This serves as an example of the significance of altering a crystal's external surface.56 The functionalization capacity of MOFs (post-synthesis or one-pot synthesis), particularly by adding biocompatible polymers or proteins, is essential for precise drug administration and minimizing clumping in the bloodstream. Nevertheless, it is crucial to solve the practical difficulties associated with attaining precise functionalization and guaranteeing long-term stability and biocompatibility to fully utilize the promise of MOFs in biomedical applications.
3.1.1 Surface absorption. Functional molecules are adsorbed on the surface of MOFs due to their high porosity and high surface area. By agitating the previously synthesized MOFs in a functional molecule solution, surface adsorption is accomplished. The forces involved in this process are hydrogen bonding, the π–π interaction, and the van der Waals interaction.15 Enzyme immobilization is a common use of surface adsorption.57 Furthermore, it is necessary to carefully examine the feasibility of surface adsorption in practical applications, considering possible concerns, such as the release or deterioration of adsorbed substances over time. Although surface adsorption offers a direct approach to functionalizing MOFs, guaranteeing the stability and longevity of the adsorbed molecules poses a notable obstacle that necessitates additional investigation and inventive remedies.
3.1.2 Pore encapsulation. A substantial number of drugs have poor aqueous solubility, which limits their systemic absorption and drug efficacy. A viable solution to this issue is to load drugs into porous materials in an amorphous form. This presents a challenge for the formulation development of orally administered dosage forms.58 Pore encapsulation via de novo synthesis is a flexible and effective method of adding functional molecules to MOFs. MOFs have high porosity and tunable pores that can be made from microporous to mesoporous, allowing for the accommodation of a broad range of functional molecules. The production and substrate encapsulation of MOFs occur simultaneously during the synthetic process. Therefore, this technique makes it possible to immobilize molecules larger than the pore sizes of MOFs inside the cavity of the MOFs. As a host material, MOFs prevent the loaded substrates from leaking and provide a barrier against external hazards.15 Nevertheless, the feasibility of this approach relies on the stability of the enclosed medications and their regulated release in physiological environments. Furthermore, it is essential to evaluate the scalability and cost-effectiveness of this technology to determine its suitability for wider use in drug formulation.
3.1.3 Covalent bond. Even though various functional molecules have been added to MOFs through pore encapsulation and surface adsorption, slow-leaching issues are frequently caused by comparatively weak contact forces between these molecules and MOFs. The surface of MOFs has various functional groups, including hydroxyl, carboxyl, and amino groups, which can be utilized to form covalent bonds with the reactive groups of the target.15 Covalent bonds are created between the functional molecules and the MOF surface, strengthening their contact, enhancing stability, and lowering leaching. Nevertheless, this technique could restrict the range of compounds that can be efficiently integrated and potentially complicate the synthesis procedure. Furthermore, it is imperative to conduct comprehensive research on the enduring stability and compatibility of these systems with the living organisms that are chemically bound together, especially when considering their use in real-world scenarios.
3.1.4 Functional molecules as building blocks. Creating functional molecules as the foundation is an additional strategy for functionalizing MOFs. Biomolecules usually contain several reactive chemical groups that can pair with inorganic metals.15 In bio-MOFs, metal ions are introduced as inorganic counterparts, and biomolecules, such as polysaccharides, peptides, amino acids, and nucleobases, are included as organic equivalents.59 Bio-MOFs typically exhibit unique biological functions and superior biocompatibility. However, it is difficult to employ most biomolecules directly to produce high-quality MOF crystals because of their low symmetry and great flexibility.15 The utilization of most biomolecules to produce high-quality MOF crystals is difficult because of their limited symmetry and significant flexibility. This emphasizes a notable constraint in this strategy, indicating a requirement for inventive techniques to stabilize biomolecules within MOFs while preserving their inherent characteristics. The practical challenges of synthesizing bio-MOFs must be considered when evaluating their potential advantages.
3.2 Loading and release mechanisms
3.2.1 Drug loading. The use of NPs in pharmaceutical treatment is crucial for improvement. When the medication loads onto a molecule, different chemistries rely on the surface of the nanoparticles.10 Post-loading, co-loading, and pre-loading are the three methods for drug loading.60 A versatile technique called post-synthetic modification (PSM) involves incorporating specific moieties into MOFs that have already been synthesized. This can be achieved by applying surface functionalization, coordination chemistry, or covalent attachment methods. PSM can precisely target ligands to ensure their availability for particular interactions while maintaining the structural integrity of MOFs.55 The loading depends on the synthesis because the stability, surface area, size, and porosity of the NPs vary. A diagrammatic model of drug loading in MOFs is now available. Due to the unique properties of MOFs, drugs can be ebbed using both one-step and two-step methods onto their surface or into their pores.10 The high loading capacity of the nanocarrier leads to a lower amount of matrix material required for administration. There are two methods for completing the synthesis: chemical and physical methods. The physical method entails the simple incubation of the nanocarrier with a concentrated drug solution to make it easier for drugs to absorb across nanoparticle surfaces. Covalent conjugation of the drug's functional group and the end group over the surface of NPs is a step in the chemical process. Because the medication may separate from the nanocarrier and be discharged into the non-targeted area, the direct attachment of drug molecules across the surface of NPs can result in major problems.61 Drugs may also be incorporated into MOFs as they form. In certain circumstances, the drug molecules may function as the constituent parts of the MOF structure, working in tandem with the metal ions. Metal-biomolecule frameworks (MBioFs) are another name for these MOFs.60 Drug stability in the synthesis solvent and the interaction between the drug and NPs can affect loading. Using UV visible or HPLC, spectrophotometry can be used to measure the loading capacity of the drugs over the nanoparticles. The loading capacity can be found using the following relationship:10 |
 | (1) |
Adding targeting moieties to MOF surfaces can boost their selectivity towards specific cells or regions. The MOF surface can be modified to include targeted ligands by grafting or layer-by-layer deposition. These ligands allow for customized administration or imaging applications by identifying and attaching to particular biomarkers or receptors on the target cells.10 The incorporation of pharmacological molecules into MBioFs as constituent components is a novel approach that requires meticulous attention to drug stability and interactions. In addition, the effectiveness of these techniques depends on achieving a high capacity for carrying substances and guaranteeing accurate targeting to optimize the effectiveness of treatment and minimize any negative effects.
3.2.2 Kinetics of drug release. The study of drug release qualities is crucial for assessing and creating nano-drug delivery systems. Many researchers use chromatographic techniques to measure the overall amount of drug delivered in animal or cell models.61 The factors that affect the drug release rate are drug solubility, drug diffusion through the nanoparticle matrix, drug absorption and desorption at the surface, and deterioration of the nanoparticle matrix. Methods other than chromatography include dialysis membrane, sample, and separation; however, each method has its advantages and disadvantages.10 Most studies employ the dialysis membrane approach, which is one of the best ways to examine the characteristics of drug release. It operates based on drug molecules being physically separated from the surface of NPs.61 The cumulative percentage for medication release can be computed as follows:10 |
 | (2) |
where Pm(t) is the drug's percentage release at a time ‘t’. Pm(t − 1) = drug release percentage prior to t.10
Drug released as a percentage = (concentration of drug released/total amount taken) × 100.61 |
The following list of kinetic models was created to depict the drug dissolution profiles over time.10
3.2.2.1 Zero-order model. The term zero model refers to the way that various coated medications, low-solubility matrix tablets, and altered release pharmacological dosages dissolve in the body. In MOF-based drug delivery systems, the zero-order model predicts a consistent release rate across time to create regulated drug release patterns.62 When MOFs exhibit complicated release mechanisms that are affected by factors, including the structure of MOFs, interactions between drugs and MOFs, or environmental factors, they may depart from zero-order release kinetics.63The following equation illustrates the dosage that does not divide and delivers the drug gradually upon drug breakdown:10
where
P0 is the starting drug concentration in the solution (usually
P0 = 0),
Pt is the amount of drug dissolved in time
t, and
P0 is the zero-order release constant, which is expressed in concentration/time units.
10
3.2.2.2 First-order model. One can apply the first-order model to various drug delivery methods, such as MOF-based ones. It provides flexibility in understanding and optimizing release kinetics in MOF-based drug delivery systems because it may reflect both immediate and prolonged drug release properties.64 It is employed to clarify the absorption and excretion of some medications; however, theoretical comprehension of this process is difficult.The following equation is an application of drug expression release:10
|
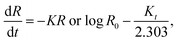 | (4) |
where
K is the first-order rate constant expressed in units of time
−1,
R0 is the initial concentration of the drug,
k is the first-order rate constant, and
t is the time.
10
3.2.2.3 Higuchi model. It offers a mechanistic understanding of drug release based on diffusion principles and is based on Fick's law of diffusion. To better understand and maximize drug release from MOF systems, it enables researchers to quantify the diffusion coefficient and release rate constant65 This model provides a mathematical analysis of the release of drugs from a matrix structure, both water soluble and low soluble. The primary presumptions of this model are as follows:10(1) Drug solubility in the matrix is lower than the original drug concentration.
(2) Drug diffusion primarily occurs in one dimension (with a negligible edge impact).
(3) The thickness of the system is greater than the size of the drug particles.
(4) There is less dissolution and matrix swelling.
(5) The drug is distributed uniformly.
(6) The release environment always meets optimal sink conditions.
The following equation provides the expression for this model:10
|
A = S[D − 2(k − ks)kst]1/2
| (5) |
where
A = the amount of drug released in time
t per unit area,
K is the initial concentration,
Ks is the drug solubility in water, and
D is the diffusivity of the drug molecule in the matrix.
The drug dissolution from different types of modified-release pharmaceutical dosage forms, such as some transdermal systems and matrix tablets containing water-soluble medications, is represented using this model.
3.2.2.4 Hixson–Crowell model. If the dimensions of pharmaceutical dosage forms, such as tablets, decrease appropriately and in a way that maintains their fundamental geometric shape over time, the disintegration of the tablets along planes perpendicular to the drug surface can be explained by this mode.10 The Hixson–Crowell model can be used in some MOF-based drug delivery systems and is helpful for drug release from solid matrices. Drug release in MOF matrices can be understood by taking its measurements as a function of the cube root of the remaining drug weight.66 However, the Hixson–Crowell model does not account for matrix material erosion or degradation, which may be significant in some MOF-based drug delivery systems. Time-dependent structural alterations, disintegration, or degradation may affect the release kinetics of MOF materials.67 Crowell proposed the cube root law, which asserts that the particle area is proportionate to its volume and describes the dissolving rate that is normalized for the decrease in the solid surface area as a function of time. Hixson and Crowell obtained the following equation10 for this drug powder with uniformly sized particles:10where K (kappa) is a constant that incorporates the surface-volume relation, l0 is the starting amount of medication in the pharmaceutical dosage form, and lt is the amount of drug left in the pharmaceutical dosage form at time t. The release from systems in which the diameter and surface area of particles or tablets fluctuate is described by the equation. The calculation accounts for release from systems in which the diameter and surface area of the tablets or particles fluctuate.10
3.2.2.5 Korsmeyer–Peppas model. The Korsmeyer–Peppas model allows for a more realistic representation of drug release kinetics by accounting for the non-Fickian release behavior commonly observed in MOF systems.68 Quantifying the release kinetics correctly when MOFs are present could be challenging. Because it assumes a homogenous matrix with uniform drug dispersion, the Korsmeyer–Peppas model may not adequately capture the complexity and diversity of MOF-based drug delivery systems.69 An equation for a polymeric system for drug release was created by Korsmeyer et al.68 The empirical formulas for analyzing drug release from swelling and non-swelling polymeric delivery systems are Fickian and non-Fickian, respectively. First, 60% of the drug release data were fitted into the Korsmeyer–Peppas model to determine the drug release mechanism:10 |
 | (7) |
where b is the release exponent, k is the release rate constant, and lt/linfinty is the percentage of medication released at time t. When describing the distinct release of matrices with a cylindrical shape, the b value is utilized.
3.2.2.6 Weibull model. The Weibull model explains many release characteristics observed in MOF-based drug delivery systems. Understanding the release kinetics and behavior of MOF systems is made possible by enabling the characterization of burst release, sustained release, or delayed release.70 Moreover, the shape and scale parameters of the Weibull model can be estimated and modified to fit the experimental data for MOF-based drug delivery systems. Understanding the release mechanism, assessing the formulation parameters, and creating MOF-based drug delivery systems can all be aided by optimizing these factors.71 The following equation, which has been described for several dissolution processes, is applied to drug dissolution release from dosage forms: |
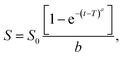 | (8) |
where S represents the drug's dissolution rate as a function of time t. The entire amount of medication released is denoted by S0. T considers the lag time that is determined as a consequence of the dissolving process, and b represents the scale parameter that governs the time dependency.10
3.3 Engineering MOFs for specific drug molecules and targets
The remarkable tunability in both the configuration and porosity of MOFs has generated significant interest in their potential use for selective drug delivery. It is necessary to appraise the MOF's toxicity after careful consideration of design parameters, such as optimal pore size, shape to accommodate particular drug molecules, and surface modification approaches to enhance drug loading and regulate release kinetics.72 After learning how metal ion selection affects medication encapsulation and stability, we can ensure that the designed MOFs are biocompatible. Then, we need to optimize the choices of organic linkers to boost MOF-drug interactions and overall performance. An aspect of this procedure is analyzing potential adverse impacts on living creatures. Research into MOF toxicity is gaining importance as we seek to create MOFs that attach to particular ligands to allow for selective drug delivery to particular cells or tissues, as well as MOFs that react to environmental cues to allow for controlled drug release under specific circumstances.72,73 This stage is crucial to guarantee that the proposed MOFs can deliver drugs properly and fulfill all the required safety criteria for biomedical applications. When MOFs, such as ZIF-8 (Zn), with PDA loaded with doxorubicin (DOX) are tested in U251 cells, they show a high level of toxicity. However, when exposed to Zn(II) biphosphonate loaded with cisplatin, CT26 cells do not exhibit any toxicity. When UiO-66 (Zr) with TPP loaded with dichloroacetate is used, U251 cells also show mild toxicity (+). However, MIL-88A (Fe) loaded with MC produces significantly less toxicity in HT-29 cells than MIL-101-NH2 with a silica shell and arginine–glycine–aspartate tri-amino acid sequence (RGD)fk loaded with cisplatin. The 293 T cells are unaffected by MC.BiTE's toxicity.72
By incorporating targeting moieties, such as aptamers, antibodies, or peptides, into MOFs, targeted drug delivery to cells or tissues becomes a reality. Consequently, there is a chance to improve therapeutic efficacy and reduce side effects, as illustrated in Fig. 4.73 Following the incorporation of ligands into the MOF synthesis process, the targeted moieties are incorporated into the MOFs during manufacturing. The pores of the MOFs can encapsulate the targeting molecules, or they might be embedded inside the MOF's structure. Imaging and tailored medication delivery are made possible by targeting the ability of moieties to selectively interact with complementary guest molecules, such as target receptors or biomarkers.72,74
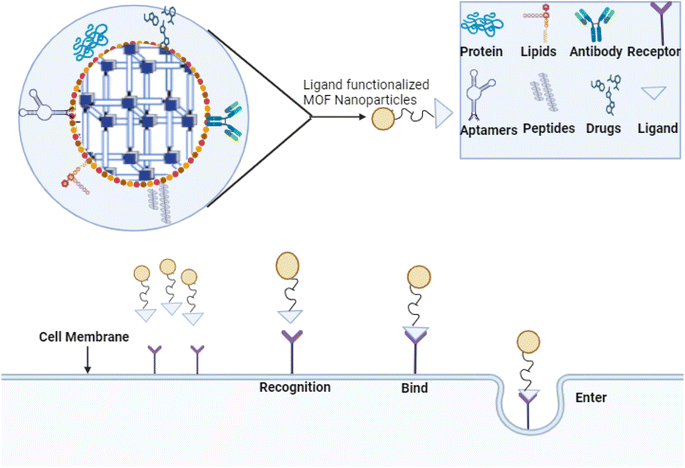 |
| Fig. 4 A schematic illustrating a drug delivery system that uses MOF nanocarriers functionalized with different ligands that bind strongly to receptors overexpressed on the surface of the target cells. | |
3.3.1 On target: MOFs and antibodies teaming up for effective drug delivery. Targeted medication delivery makes considerable use of proteins, which encompass a broad class of biological molecules. To target the distribution of drugs, researchers have used the advantage of several membrane-binding proteins, antibodies, and transferrin receptors.75 Biotechnology and biomedicine have recently demonstrated interest in MOFs in the form of nanocrystals. To improve biobanking and immunosensing, MOFs are coupled with Abs, either encapsulation or site-specific conjugation.76 Encapsulating Abs within ZIFs protected the protein against heat and solvents, as demonstrated by Qi et al. Abs were biotinylated on the surface of the COOH-rich MOFs to enhance biodetection. A generalized strategy for immobilizing Abs on MOFs was described by Qi et al. Their method relies on the simple derivatization of surface acid groups to supply peptide linkages.77 The ability to employ biomacromolecule surface chemistry for spatially selective MOF crystallization was demonstrated by Alt et al. Using Abs as a catalyst, a zinc-based MOF (ZIF-C) was grown on the Ab Fc area in a singular step. The Fab regions protruded from the MOF nanocrystal surface and targeted the designated antigen in a MOF biocomposite (ZIF-C*Ab) formed by the localized growth of ZIF-C on the Fc region. The effective incorporation of QDs into ZIF-C*Ab and the quantified targeting performance proved that this procedure might be used for biosensing.78 From antibody-based sensing to diagnostic and therapeutic applications, this simple synthetic technique could be taken to the next level. Personalized therapeutic development stands to benefit greatly from the creation of an immunotrapper system based on MOFs that integrates controlled drug delivery with tailored cell sequestration.77
3.3.2 Peptide-driven precision: engineering MOFs for tailored drug delivery and targeted therapeutics. Peptides are small chains of amino acids that play important biological roles and are frequently utilized as ligands to target tumors by recognizing overexpressed receptors.79 RGD is a popular peptide motif because of its capacity to target angiogenesis and its effect on cell adhesion. Implementing MOFs with RGD and its analogs has been performed for targeted applications. One application of cyclic RGDfK-functionalized MOFs as an MRI agent was performed in 2008. By enhancing target-specific absorption, the developed nanoplatform enhanced the transport of peptide-functionalized MOFs to cells afflicted with human colon cancer. A nanostructure metal–organic framework (nMOF) based on Gd was described for targeted in vivo MR imaging using dual-mode T1- and T2-weighting. To create NPs that respond to both pH and redox-sensitive stimuli, RGD peptide was also used to functionalize MIL-101 nMOFs.73,80 Using a post-synthetic modification procedure, the nMOF probe that had been synthesized was made functional, with methylene blue acting as a photosensitizer and a β-diketonate derivative as a two-photon-absorption (TPA) agent. The nMOFs that were created, called MB@THA-nMOFs-76@cRGD, could absorb 808 nm near-infrared light, transfer energy to Eu, and emit 615 nm light. Finally, peptides have demonstrated promise in several biological contexts, such as imaging, photodynamic treatment, tumor-targeting ligands, and drug/gene delivery.73 Additionally, various peptides were employed to direct the buildup of NPs in cancerous tissues actively.
3.3.3 MOFs and aptamers: crafting targeted elegance in drug delivery. Aptamers are oligonucleotides with one strand of DNA or RNA that have been randomly selected using the SELEX method. They can fold into three-dimensional structures and bind to target molecules with remarkable selectivity. They have been appealing targets for targeted therapy, particularly in cancer treatment, due to their small size, stability, rapid synthesis, low cost, and lack of immunogenicity. Additionally, aptamers can bind to metal ions, ATP, and proteins.73 Targeted distribution of DOX has been achieved using Zr-based MOF nanoparticles, with VEFG aptamers serving as locking units. Cancer cells can be targeted using the AS1411 aptamer sequence, which enables on-site medication release that is responsive to stimuli. For their usage as immunotrappers, hydrothermal methods were used to synthesize Zn-based MOFs, primarily in ZIF-8 NPs.73,81 A possible cancer marker called biotinylated anti-epithelial cell adhesion molecule (EpCAM) was attached to them, and streptavidin was used to cover them. The effectiveness of capture was higher in cells that were positive for EpCAM. Cell-selective surface glycan remodelling using aptamer-functionalized MOF NPs was also explored for potential utility in cancer treatment. Using TLS11a aptamers that selectively target HepG2 cell receptors, a galactose oxidase@ZIF-8@Apt system was created.73 A red blood cell membrane (RBCm)-inserted anti-lymphoma targeted molecular CD20 aptamer formed the shell of a smart anti-lymphoma nano drug delivery system that was suggested. The core of the system was an Ag MOF loaded with a tumour aerobic glycolysis inhibitor (PFK15). By facilitating mitochondrial apoptosis, the synthesized nanocomposite mediates mitochondrial outcomes. Active targeting, sufficient biocompatibility, reactivity to mild acids, real-time monitoring of drug release, and synergistic anticancer effects were among the many beneficial qualities displayed by the resultant multifunctional nanocarrier with aptamer.73,82Overall, the addition of targeting moieties to MOFs allows for the targeted delivery of medications to certain cells or tissues. These moieties can be antibodies, peptides, or aptamers. There may be fewer negative effects and an improved efficacy of therapy because of this.
4. Characterization techniques for MOF materials
The generated MOFs should be further characterized utilizing various physicochemical techniques to determine their characteristics.83 SEM and TEM are imaging the surface morphology of the manufactured MOFs. MOFs can have various morphologies based on several variables, including the molar ratio of doped metals.84 Because MOFs are crystalline materials, it is important to determine their crystal parameters and size. For this reason, the X-ray diffraction (XRD) technique should be utilized.84 A fundamental technique for verifying the phase purity and crystallographic characteristics of a substance is XRD.85 The NP size is determined using the Scherrer equation based on the XRD peak broadening in the powder diffraction pattern.84
Thermogravimetric analysis is a method used to assess the thermal stability of the prepared nanocomposites.86 The method mostly employed to measure a particle's hydrodynamic diameter is probably dynamic light scattering. The size of a hypothetical homogeneous hard sphere diffusing similarly to the particle under study is known as the hydrodynamic diameter.84
A proven vibrational method for molecular-level chemical analysis of materials is infrared absorption spectroscopy. As vibrating functional groups interact to absorb the incoming infrared light, they undergo changes in their electric dipole moments in the infrared active transitions.87
Because of their large surface areas, high pore volumes, flexible topologies, multifunctional characteristics, and inherently adjustable structures, MOFs have garnered much attention in the last few decades. Because of these qualities, MOFs have frequently been chosen materials for several applications, such as medication delivery, gas storage, separation, and catalysis. An essential comprehension of the crystallization mechanisms of this novel class of porous materials can be obtained through the manipulation of the primary structural elements, such as organic ligand molecules and metal ions. According to our current knowledge, MOFs as crystalline materials are made up of simple geometrical structures called secondary building units (SBUs), which are well-defined molecular clusters rather than single atoms. SBU is essentially a helpful tool that was discovered for the examination of intricate MOF structures. The current literature has thus far described MOF crystal structures in various ways depending on abstraction or deconstruction techniques. To control the size and morphology of MOF crystals in the micro/nano size ranges, various critical variables are chosen during the synthesis of MOFs, such as composition (choosing metal ions and organic linkers), process parameters, temperatures, additives, solvents, molar ratio of reactants, and reverse micro- or macroemulsions.88
There are two prevalent methods for measuring the surface areas of MOF materials.89 The isotherm-based method, which involves determining the monolayer coverage using the Brunauer–Emmett–Teller (BET) theory,90 and the geometry-based method employs a probe molecule to traverse individual atoms and calculate the accessible surface area. The BET study is conducted by examining the adsorption isotherms of inert gas molecules at various pressures that encompass the monolayer coverage of molecules. The isotherms acquired are converted into a linearized BET plot, allowing for the determination of the monolayer loading. It is important to mention that the method can also be applied using adsorption isotherms derived from molecular simulations.91–93 The surface areas of MOFs are often determined using the BET method, which relies on measuring the adsorption isotherms of nonreactive gases. Recently, there have been reports of differences in surface areas calculated using the BET approach and those obtained using geometric methods.89 However, a drawback of the BET approach is the selection of the linear zone from the linearized BET plot. In the BET analysis, the linear region is often selected within a relative pressure range of 0.05–0.30, which is commonly referred to as the BET standard pressure range.94–96 Nevertheless, in 2007, Rouquerol and colleagues proposed that the conventional range is not appropriate for microporous adsorbents because monolayer formation in these structures occurs at extremely low relative pressures (P/P0 < 0.05). Walton and Snurr have shown that the conventional BET pressure range is not suitable for microporous MOFs, as the pores become filled with adsorbate molecules at pressures significantly lower than the typical BET range.97,98
5. Applications of MOFs in drug delivery
5.1 Cancer therapy: targeted delivery of chemotherapeutic agents
Each year, millions of people die from cancer, a danger to public health. Radiotherapy, chemotherapy, and surgery are examples of conventional therapeutic techniques.99,100 Chemotherapy, however, has drawbacks, including poor drug transport and lack of selectivity, resulting in insufficient concentration in target areas and severe harm to healthy tissues.101,102 To decrease side effects and raise therapeutic indices, efforts have been made to create innovative drug delivery systems. The goal of this strategy is to increase the efficiency of radiation and chemotherapy in the treatment of cancer.
Understanding the processes governing cell regeneration and proliferation is essential for developing effective cancer therapy, as cancer is a complicated illness characterized by RNA damage. Targeted cancer therapy looks for effective noninvasive techniques to target the specific areas where cancer cells are growing and multiplying.103 It also addresses the causes and visualization of cancer cell growth and dispersion. Nanochemotherapeutics are used in nanomedicine, the application of NPs in medicine, to cure cancer. Since the beginning of the twenty-first century, this discipline has grown as the focus has shifted from disparate to clinical research.104 To detect and treat cancer, nMOFs are extensively employed as customizable theranostic platforms. These include monomodal medicines, such as photodynamic therapy (PDT), chemotherapy, radiography, and immunotherapy, as well as multimodal/combined imaging, thermal, and chemotropic treatments.105
MOFs are nanocarriers that allow for surface engineering and high mass fraction drug loading due to their ultrahigh specific surface area and abundance of functional groups. Because their chemical structure may be altered to provide the desired results in terms of size, shape, content, and surface functional groups, they are desirable candidates for active targeting in drug and cargo delivery.
To increase the therapeutic efficacy of nMOFs, significant attention has been paid to their modification and functionalization over the last ten years. Multifunctional nMOFs, whose surface alterations occur during self-assembly and post-synthetically, have been created for targeted cancer treatment. MOFs with polymer coatings or wraps have been created to increase their range of biological applications. Stability and dispersion are improved when reactive polymers, such as carbohydrate polymers, are attached to MOFs. Polymer-wrapper nMOFs are also developed using radical polymerization techniques and the GraftFast technology. MOFs coated with a molecularly imprinted polymer (MIP) have been created for oral delivery and selective species detection in biological settings.106
Stimuli-responsive MOFs hold significant promise for drug delivery and cancer therapy because they are based on the characteristics of tumour microenvironments, such as low pH. By both passive and active targeting, these nanocarriers build up at the tumour site, where they accelerate medication release while blocking its premature release in healthy cells and the bloodstream. Because of increased drug aggregation in tumour cells, this increases the rate of tumour inhibition.107
Both passive and active targeting can be used to accomplish tailored medication delivery in tumour microenvironments, as demonstrated in Fig. 5. Active targeting, also referred to as smart drug delivery, is the process of functionalizing drug-loaded nanocarriers with different ligands, such as peptides, proteins, antibodies, aptamers, and small molecules. Consequently, medications are more concentrated and bioavailable in the targeted tissues and organs, improving therapeutic effectiveness and lowering the cytotoxicity and adverse effects of chemotherapeutic treatments. Reduced cytotoxicity and adverse effects, as well as increased therapeutic efficacy, are linked to the efficiency of active drug targeting.108
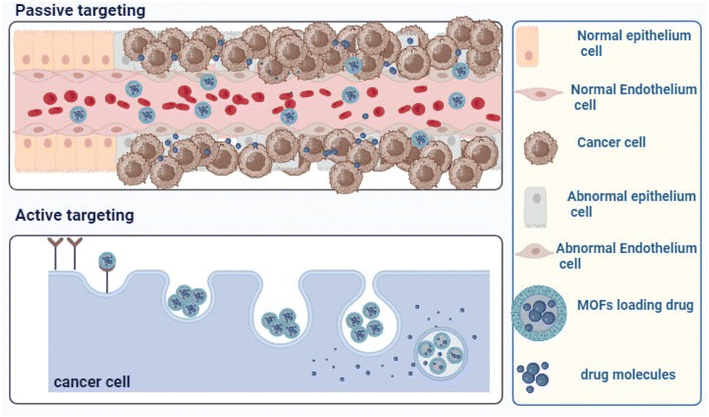 |
| Fig. 5 Diagram for passive and active targeting. MOF-loaded nanocarrier: it cannot diffuse through normal endothelium and can only partially cross it in both directions with small molecules of free drug. In the tumour area, gaps between endothelial cells appear, which allows NPs to propagate and accumulate, resulting in high local concentrations of the drug. MOFs are drug loaded and can identify specific cell surface receptors. Consequently, the medication is released from the carrier on the cell surface where it is still attached, or the drug can be absorbed to enter the target cells. | |
MOFs may be decorated with various ligands, including proteins, peptides, aptamers, antibodies, small molecules, and polysaccharides, and they are perfect for surface functionalization. These ligands increase selectivity and reduce multidrug resistance by binding to particular receptors on neoplastic cells, including transferrin, integrin, folate, and epidermal growth factor.109
Targeted drug delivery is a common application of proteins, and membrane-binding proteins, such as CD44 and FA receptors, are created for this purpose. To treat cancer, researchers have considered FA-modifying MOFs with improved cellular uptake.110
5.2 Infectious diseases: antibiotic and antiviral delivery
Instead of using conventional antibiotics, new approaches, such as MOFs, must be developed in response to the global rise in antiviral diseases and antimicrobial resistance. The overuse of antibiotics and self-medication has led to bacterial resistance, which is a global problem that has killed many people. With antimicrobial resistance currently ranking as the primary cause of death in 204 countries, mutated and resistant pathogenic viruses pose a serious threat to human health. Developing sophisticated nanomaterials and nanotechnology in bio- and nanomedicine provides an option to create intelligent nanosystems to fight these infections. MOFs are porous coordination polymers that exhibit favourable characteristics, including exceptional surface area, crystal structures, and structural diversity. They can be used in controlled drug delivery, ion exchange, heterogeneous catalysis, energetics, and synergy engineering in nano- and biomedicine. MOF-based sensing systems have demonstrated the ability to identify certain viruses.
With effects ranging from bacterial inactivation and death to the elimination of antibiotic-resistant genes, the suppression of biofilm development, and the complete eradication of biofilms, MOFs are crucial in antibacterial applications.111 These effects, which result in bacterial cell damage and functional loss, such as ion channel and cell membrane destruction, enzyme inactivation, protein denaturation, mitochondria, and DNA destruction, are attributed to their diverse antimicrobial actions, which include cation transport interruption, diffusion-directed lipid-oxidation, direct interaction, photogenerated ROS (reactive oxygen species) formation, and membrane depolarization.112 Because of their varied inorganic and organic components, tunable water/acid/base stability, and accelerated decomposition capabilities, MOFs can also be utilized as component-releasing antibacterial agents. When submerged in water, water-sensitive MOFs readily break down to liberate their constituent parts; the pace of this release is based on the water stability of the MOFs and may be regulated by modifying the coordination of MOFs in accordance with the Pearson hard/soft acid/base theory.113
Depending on the various antibacterial activities of the various components, MOFs with the right water stability must be chosen to provide the best and longest-lasting antimicrobial effects. Most MOFs break down in response to external stimuli, releasing their metal ions and organic ligands at predetermined times for focused, on-demand use.
The primary methods of photocatalytic antimicrobial action are photothermal lysis, photodynamic death, disinfection, and photogenerated ROS, which are created by semiconductors that are stimulated.114 When photons with energy equal to or greater than a MOF's HOMO–LUMO gap are absorbed, excited negative electrons (e−) can escape from the MOFs to the LUMO, and positive holes (h+) are left on the MOFs. This is possible when the MOFs have an appropriate band structure. In photocatalysis, hydrogen peroxide (H2O2), singlet oxygen (1 O2), O2−, and OH− are the four main photogenerated ROS.115
By making coordination metal ions in MOFs and bacterial cell membranes more compatible, chelation disinfection increases the permeability of bacterial cell membranes.116 Through chelation, MOFs containing numerous surface-active metal ions are expected to render a variety of bacteria inactive. Physical disinfection, which primarily uses unique morphology, nanosize, and functional surfaces with passive anti-adhesive and active contact-killing capabilities, is an effective and safe antibacterial technique that does not require the release of drugs or external chemicals.117 Although highly positively charged and nanowire-shaped MOFs are good for adhering, penetrating, and bursting bacterial membranes, hydrophobic, rough, and micro/nanostructured MOFs tend to reduce bacterial growth and adhesion. In particular, for films, membranes, and coatings, the combination of the two elements is more efficient in increasing their antibacterial effectiveness, antifouling performance, and antimicrobial durability. Because of their porosity, many exposed cations, tunable shape, ease of functionalization, and modifiable hydrophilic and hydrophobic characteristics,88 MOFs are good candidates for physical antibacterial agents.
In various applications, such as photocatalysts, chelation antibacterial materials, and component-releasing structures,118,119 MOF-based systems are effective antimicrobial agents. The potential of MOFs for antimicrobial photodynamic treatment has been investigated. Indocyanine green-loaded MOFs against E. faecalis showed an effective decrease in the number of pathogenic bacteria and esp gene expression. MOF-based nanocomposites, such as humic acid-encapsulated ZIF-8 nanocomposites, showed fast antibacterial activity against 99.59% of S. aureus and 99.37% of E. coli when exposed to near-infrared light.120 These nanocomposites offer light-responsive platforms that are reasonably biodegradable and reasonably priced for quick and sensitive sterilization procedures. Although MOFs have also been employed as photosensitized materials, the ultrashort diffusion distance of biocidal ROS limits their bactericidal effects. To overcome this issue, photosensitized porphyrin and bacterial-binding boronic acid ligands were added to the MOFs to enhance antibiotic potentials in a complementary manner. These highly biocompatible multivariate MOFs have demonstrated superior efficacy against germs that are resistant to many drugs while mitigating inflammatory reactions and promoting the healing of chronic wounds.121
The biomedical uses of MOFs are influenced by stability and degrading behaviour, which have great significance for the material's future usability.
For ages, viruses and humans have coexisted, resulting in yearly outbreaks and global health crises. When zoonotic viruses undergo genetic alterations to adapt to humans, a new wave of viruses infecting people is created. The growth of the global transport network makes it possible for infections and their vectors to spread more quickly and widely, which leads to pandemics of infectious diseases. MOFs are nanoporous materials that may be customized for certain uses to identify and manage viral infections.
Because of their special characteristics, MOFs are perfect for delivering vaccines. They boost long-term immunity and induce a favourable immunological response by effectively catabolizing antigens and adjuvants to immune cells. Because of their pH-responsive characteristics, which minimize off-target release and improve vaccination effectiveness, they can be delivered precisely to the right cells. Additionally, MOF carriers improve the stability of vaccine molecules by acting as armour around them, making it possible to administer certain vaccinations orally.122
Because MOFs are physiologically stable, they can be used to preserve vaccinations until cells absorb them. Because oral vaccinations are simple to administer and can elicit full immune responses, they are preferred. Direct GI administration, however, has drawbacks, including antigen degradation and challenges in encouraging high cellular absorption by microfold cells in the GI mucosal membrane. MOFs have demonstrated significant promise in the administration of vaccines by encapsulating and delivering adjuvants as well as antigens. It is now necessary to improve the protection and cellular absorption of mRNA, DNA, and proteins due to recent advancements in vaccination technology used to battle the coronavirus pandemic.123
Certain MOF NPs exhibit antiviral properties in addition to being employed for the delivery of antiviral drugs. Several metals, including copper, zinc, and silver, have demonstrated some degree of antiviral activity, making metal–organism frameworks based on the potential of these ions to counter viral threats. Cu@ZIF-8 nanowires (NWs), for instance, are an antiviral MOF core–shell nanocomposite created by developing a ZIF-8 layer on the surface of Cu NWs coated with pluronic acid.124 ZIF-8 was applied to the NWs to coat them, slow down the release of copper ions, and preserve the antiviral activity while lowering the possibility of copper-induced toxicity. Following SARS-CoV-2 infection of VeroE6 kidney epithelial cells and in vitro incubation with Cu@ZIF-8 NWs, qRT-PCR was run on viral RNA isolated from the supernatant in the cured cells.125 Research indicates that copper functions as a potent antiviral agent; SARS-CoV-2 was rendered inactive on copper surfaces for more than 4 hours. It has been demonstrated that Cu@ZIF-8 NPs had very little cytotoxicity; 48 h after exposure, 99% of the host kidney epithelial cells were still alive. Over time, the cytotoxicity rises due to the prolonged release of copper ions. MOFs can also function as superior filters, with filtration efficiencies of 70–80% for particles smaller than 0.3 μm. MOF systems can be made more effective by surface functionalization with additional antiviral drugs, such as carotenoids or folic acid. MOFs may be surface-functionalized with FA, nystatin (Nys), or tenofovir (Teno) and bind to viral capsid proteins, immobilizing viruses and halting reproduction, according to proof-of-concept research by Desai et al.126
5.3 Chronic conditions: sustained release formulations and implants
Because they require less frequent medication administration than standard formulations, extended-release formulations provide several advantages. This is because medications are released gradually and smoothly, maintaining drug concentration within the range of effective blood concentrations, assuring the duration of therapeutic activity, lowering dosages, and improving patient compliance. Innovative porous hybrid functional materials called MOFs have several benefits over conventional mesoporous materials. High therapeutic drug loading, biodegradability, and the capacity to investigate host–guest interactions are among their benefits. As a result of MOF optimization for drug loading and sustained release from nanoparticulate drug formulations or coatings, the traditional definition of biomedical MOF applications has mostly focused on drug delivery or diagnostic uses. MOFs are perfect for medication delivery because of their precise control.66
MOFs are highly organized materials with a large pore capacity, high specific surface area, and easy modification, making them perfect for prolonged drug release. They also degrade readily in vivo. Drug release occurs in many phases, beginning with surface breakdown and progressing through progressive diffusion, as the concentration gradient changes within the material.127 The collapsing structure releases the medication enclosed in the material cavity. There could be some affinity between the medication and substance, which would cause the drug to release slowly over time. MOFs have been reported to deliver various chemotherapeutic medicines and can accomplish gradual or continuous drug release. Consequently, they are a great medication delivery carrier material. When compared to pure PAN nanofiber mats, Fe-MOF scaffolds showed improved adhesion, proliferation, and spreading of human umbilical vein endothelial cells (HUVEC). They did not trigger inflammatory reactions in vivo and were cytotoxic in small doses. Fe-MOFs at an ideal concentration strike a compromise between cytotoxicity and scaffold breakdown. However, it has a detrimental effect on cell activity but a beneficial effect on pH levels at the biointerface.128 About 4% of people in developed countries have coronary artery disease (CAD), which calls for medical attention. Traditionally, patients have received drug-eluting stents in severe situations, small molecular weight nitric oxide donors, and antithrombotic medications. Recently, anti-thrombotic coatings for cardio-vascular implants have been developed using MOFs based on copper.129
The use of copper-based microfluidics in antithrombotic coatings for cardiovascular implants has grown in popularity. In vitro, blood-borne s-nitroso-cysteine can be converted by Cu-BTC into cysteine and nitric oxide, whereas more sophisticated Cu-MOFs can catalyze s-nitrosoglutathions. Because of their nitric oxide release characteristics, MOF/polymer composite materials are promising as lead materials for innovative implants. The direct growth of Cu-BTC on stent surfaces resulted in good hemocompatibility in vitro. For cardiovascular implants, the MIL-101 (Fe) polycaprolactone composite was assessed as a viable non-copper-based MOF.130 It has been discovered that blood-borne s-nitroso-cysteine may be effectively catalyzed to produce cysteine and nitric oxide using Cu-BTC. To catalyze the conversion of s-ntirosoglutathione, more sophisticated Cu-MOFs have been studied. This has resulted in the creation of MOF/polymer composite materials for nitric oxide release as possible implant materials.131
In vivo Cu-BTC has been demonstrated to be useful in altering the polydopamine coating on cardiovascular stent surfaces, which decreases platelet adhesion, thrombus formation, and protein absorption and increases vasodilation. Injecting nitric oxide donors has a very positive impact. A 5-(1H-tetrazol-5-yl) isophthalic acid-based MOF was employed in a different investigation to maintain a rat model free of arrhythmias. Subsequent studies must assess the safety and efficacy of MOFs in comparison to clinically used materials.132
Most studies on MOFs in TERM applications concentrate on enhancing structural bone implants although a sizeable portion of failures is introduced by unfavorable wound conditions, which may be made worse by bacterial infection. The biological impact that comes from outside the MOFs, acquired bioactivity by drug loading, allows MOFs to improve bone regeneration. Orthopaedic implant coatings are drawn to MOF-based drug carriers because of their great capacity for drug encapsulation and low rate of premature release.133
To heal critical-sized bony defects, guided bone biomaterials have been created for bone tissue engineering. During the healing process, osteoblasts can specifically repopulate bone defects owing to GBR, especially in the maxillofacial area. Two primary varieties of GBR membranes are non-resorbable and resorbable materials.134 Because collagen (Col) membranes are biocompatible and biodegradable, they are useful for biological applications; nevertheless, their mechanical qualities are inadequate, and they break down quickly.135 Microorganism-MOFs possess a noteworthy pore structure, a substantial surface area, and the capacity to be functionalized in several ways, making them promising biological platforms. Enzyme-MOFs can immobilize on porous and flexible membranes, and MOF layers have been effectively employed to support both in vivo and in vitro cellular behavior. A faster rate of design and synthesis is observed for multifunctional MOF-based biomaterials. ZIF-8 has good chemical and thermal stability, sensitivity to pH changes, and little cytotoxicity, making it a great option for use in bone regeneration procedures.136
MOFs have unique sustained release abilities for small molecular weight drugs, such as dexamethasone, which was successfully released for 4 weeks in cellulose-hydroxyapatite nanocomposites for load-bearing orthopedic applications.137 MOFs can facilitate the osteointegration of bone implants and improve clinical outcomes. Examples of bone diseases treated with MOFs include naringin, antitubercular drug delivery for osteoarticular tuberculosis, and vancomycin for osteomyelitis. MOF-based drug carriers allow for the design of elaborate release mechanisms tailored to specific triggers. However, only one study has described the pH and Ca2+ ion-dependent release of drugs into the bone environment using Zr-MOFs capped with CP5-based pseudorotaxanes.138 An in vitro investigation of implant materials modified with ZIF-8 particles loaded with levofloxacin revealed enhanced osteoblast adhesion, proliferation, and differentiation. The study concentrated on pH-triggered release in acidic settings introduced by bacterial infections and inflammation. In a rat model of an infected femur, the material's osseointegration and antibacterial qualities were verified.139 Nerve tissue loss results from glial scarring and defective regeneration introduced by traumatic nervous system injury. Both neurosensory or cerebral damage and physical paralysis may result from this. To improve these materials, biomaterials for the regeneration of nerve tissue, including MOFs, are being developed. In neurobiological applications, MOF-based drug delivery systems, such as cationic MOF-74-Fe(III) and MIL-88A, have demonstrated flexibility.140 These systems can deliver IBU passively, which is a medication known to lessen neuroinflammation, or they can use magnetophoretics to induce the release of dopamine.141 Zr4+ ions and amino-triphenyldicarboxylic acid can also be used as the basis of MOFs to induce ATP-responsive drug release. MOF particles must be able to permeate the blood–brain barrier for systemic injection to be used in studies such as ZIF-8. Microfluidic vascular transport models for pericyte-based NPs might be useful in future research to better simulate the blood–brain barrier and investigate the biological response of various cell types in inflammation and repair.142 A Cu(II) MOF was used in a mixed linker method with 5-methylisophthalic acid and 1,3-bis(5,6-dimethylbenzimidazol-1-yl)propane in vivo investigations. This resulted in the inhibition of dopaminergic neurons, decreased release of dopamine, decreased apoptosis, and enhanced activation of Wnt1-Nkx2.2. Up to 5 mg kg−1 body weight, the biological effects were shown in a dose-dependent manner; however, there was no comparison between the MOFs and a solution that contained just the ligand.143 The evidence demonstrates that MOFs have a robust biological response to successful healing, resulting in a range of tissues and applications. To comprehend the dose-dependent response of distinct cell types and tissues to various MOFs, additional work is necessary. Additionally, before MOF-based biomaterials may enhance healing results in the treatment of human patients, questions about the efficacy of alternative linkers and the safety of organic linkers for clinical applications must be addressed.
6. Overcoming challenges in MOF-based drug delivery
6.1 Stability in physiological environments
The stability of MOFs in physiological environments is a critical factor to consider for their effective use in biological applications, given the complex nature of body fluids, cells, and tissues.144 When nMOFs are explored for various bio-applications, their structural stability in aqueous environments is a concern. Factors such as metal–ligand bond strength, ligand basicity, coordination number, and metal center oxidation state affect the stability. The presence of high ionic strength, biomolecules, and slightly acidic to neutral pH in biological systems can potentially disrupt the coordination bonds and non-covalent interactions within bio-MOF assemblies, leading to structural disintegration or distortion over time.145 There are some ways to overcome the problem of stability in the body fluids. Strategies such as catenation and interpenetration, as well as using high pKa value linkers can improve stability. For bio-applications, MOFs need chemical stability to reach target sites and degradability to release cargo in response to pH and fluid composition changes.146 To enhance stability, biocompatible coatings, such as lipid bilayers, silica, cell membranes, proteins, chitosan, polymers, or antibodies, can be applied to externally protect bio-MOFs from harsh immunological secretions and conditions.145 Layering techniques can be used to create encapsulating nanocoatings by alternating layers of oppositely charged polymers through electrostatic interactions, thus preventing structural collapse.144 Covalent grafting of polymers onto the external surface of bio-MOFs via reactions with functional groups can chemically attach protective polymer chains. Sol–gel synthesis using TMOS-like gel shells can also provide stability. Artificial intelligence can help to promote stability. For example, computational modeling through molecular dynamics simulations can offer atomic-level insights into the vulnerability of bio-MOFs to dissociation or denaturation.147 Overall, researchers still require further studies in full culture media or simulated body fluids to better understand and improve the stability of MOFs in biomedical applications.
6.2 Biodegradability and biocompatibility concerns
Biocompatibility is a crucial aspect when creating and synthesizing MOFs for biomedical applications. To ensure safety for living tissue, non-toxic alternatives such as iron, zinc, and zirconium are often substituted for metals such as chromium. The water stability and biocompatibility of MOFs are important considerations due to their potential for degradation, metal leaching, and toxicity.148 However, MOFs can be functionalized to enhance their physiological features, such as improved colloidal stability, reduced cytotoxicity, and enhanced cellular absorption. This makes them superior to traditional nanocarriers in biological applications, especially for drug delivery systems.149 The biocompatible components, high loading capacity, and bio-preservation qualities of MOFs make them ideal for encapsulating complex chemicals and transporting larger molecules, such as hormones.27 Although some MOFs have shown in vivo compatibility, further evaluations of their toxicity, long-term biocompatibility, and specific parameters, such as degradation routes, metabolites, target specificity, and side effects, are necessary.150 The biocompatibility of MOFs is determined by various factors, such as degradation kinetics, biodistribution, accumulation in tissues and organs, excretion from the body, and the balance between risks and benefits.151
The fatal dosage and daily dose of metals are used to determine which cations are most suitable for MOF synthesis. The acceptable metals for MOF construction include Ca, Mg, Zn, Fe, Ti, and Zr; however, the daily dosage and chemical formulation affect these amounts. In certain uses, including cosmetics, metals, such as Zr and Ti, are not deemed harmful because they are not well absorbed by the body.152 To create biocompatible MOFs, liquid ligands can be categorized into exogenous and endogenous groups. Exogenous ligands are artificial linkers that are not present in the body naturally and that, once applied in vivo, need to be eliminated or broken down.153 Functionalizing exogenous ligands with polar and apolar functional groups can modulate their absorption, distribution, metabolism, and excretion behaviors, as well as improve the absorption and delivery of cargo biomolecules. Examples of functionalized MOFs include UiO-66(Zr), MIL-125(Ti), MIL-53(Fe), and MIL-88B(Fe).151
The biocompatibility and biodegradability of MOFs play a crucial role in ensuring their safety for biomarker sensing in the human body.154 However, the current research primarily focuses on improving sensing performance without paying sufficient attention to biocompatibility.154 Therefore, it is critical to address the possible risks of MOFs to human health.154 The biocompatibility of MOFs is affected by elements, such as the type of metal and ligand utilized in its structure, as well as physicochemical properties, including particle size, structure, and hydrophobicity.155 Oxidative agents and pH conditions can also influence the degradation path of MOFs under oxidative conditions, and the hydrolysis of the coordination bond between the ligand and metal can induce degradation. The type of metal ion in the MOF structure is critical, as it can be released upon degradation and cause toxicity. The nature of the released metal ions can either produce ROS that damage cells or help neutralize ROS.156 Furthermore, the toxicity of MOFs is dose dependent, with different concentrations affecting biocompatibility.156 Consequently, only molecules with specific functional groups or opposite charges, such as DOX, CRU, QT, and CPT, have been successfully encapsulated. The challenge lies in encapsulating small molecules or drugs without specific functional groups, as they tend to leak from the MOF's pores. Although a few studies have proposed strategies to address this issue, research in this area is still in its early stages.157 To overcome these limitations and enhance biocompatibility, future studies should concentrate on developing new MOFs with improved biocompatibility and targeting capabilities.158 This can be achieved by integrating sustainable precursors, such as biomass-derived or waste-derived materials, and developing green and sustainable synthesis methods that produce biodegradable MOFs to enhance the sustainability, biosafety, and biocompatibility of MOF synthesis in biomedical applications.159 Furthermore, synthesizing MOFs with biodegradable linkers or designing MOFs that can resorb in response to specific triggers.158 The kinds of metal ions, organic linkers, dimensions, surface chemistry, and colloidal biostability can all affect the biocompatibility and biodegradability of MOFs. Manipulating the biodistribution of MOFs in vivo can assist in managing their pharmacokinetics, toxicity, and associated immune responses, resulting in increased biosafety.160,161 The size, structure, and many other physicochemical properties of MOFs can be adjusted by selecting the synthesis method and controlling the synthesis parameters. MOFs synthesized using a suitable strategy with desired properties have led to different biomedical applications.156 The N, O, and S heteroatoms of drug molecules can work with non-harmful nodes to create porous MOFs, such as medical MOFs made of bioactive curcumin. In addition to guaranteeing strong biocompatibility, it can offer a workable solution for the simultaneous delivery of two drugs.162 Therefore, future studies should continue to explore the relationship between the characteristics of MOFs and their biocompatibility, aiming to develop more effective and safer MOFs for biomedical applications.
6.3 Regulatory hurdles and clinical translation
Regulatory hurdles present significant challenges for the clinical translation of metallic nanoparticles, including nanoparticle-based systems such as MOFs. Regulatory agencies, such as the FDA and EMA, impose additional scrutiny on novel therapies, requiring extensive safety, efficacy, and quality data before approval for clinical use. This entails conducting rigorous preclinical and clinical trials, which can be time-consuming and costly.163,164 Furthermore, pharmaceutical companies and drug manufacturers often prioritize modifications to current technology that already had FDA approval rather than investing in novel therapies, highlighting the need for a change in mindset.165 Another obstacle is the need to create efficient synthesis methods for a broader selection of clinically significant medications, as current research has focused on only a few drugs. Moreover, few studies have investigated the drug release kinetics of MOFs to ensure their capability to successfully release the drugs they carry.166 Addressing these challenges is crucial for the progress of MOFs such as DDS. Efforts should focus on identifying targeting biomarkers, precise conjugation, and developing stable and low-toxicity MOF carriers. To address the barriers to clinical trials, functionalized MOFs with bioactive substances to reduce toxic effects are used.167 Although the practical application of MOFs as commercial materials is restricted by various factors, such as production yield and cost, certain uniquely functional MOFs still have competitive advantages in specific fields. Hence, reducing the production cost of MOFs and optimizing the manufacturing process are necessary for broader-scale applications.168 Extensive research is needed to achieve successful drug delivery by employing various stimuli, as well as a complete understanding of MOF degradation processes and routes in vivo. The pharmaceutical industry can utilize MOFs in the future.169
7. Recent advances and innovative strategies
7.1 Stimuli-responsive drug release systems
Smart compounds, such as stimuli-responsive MOFs, appear to be promising candidates for nanomedicine. In biomedical applications, MOFs and other participant materials must withstand different conditions, such as temperature, pH, light irradiation, magnetic fields, ionic strength, and the presence of redox reagents, serving as valuable stimulants, as illustrated in Fig. 6.170 When stimuli-responsive MOFs are triggered, either externally or intrinsically, they undergo specific transformations in their physical structure or chemical composition, leading to significant changes in their physicochemical properties.171
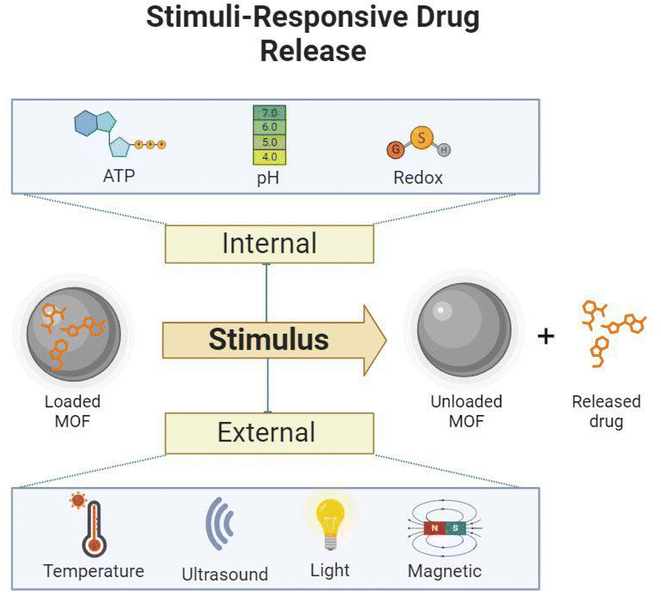 |
| Fig. 6 Two main categories of stimuli. | |
Internal stimuli include factors such as ATP levels, pH, and redox potential within the body. However, external stimuli encompass factors from the organism's external environment, such as temperature, ultrasound waves, light, and magnetic fields. External triggers, such as thermal, magnetic, electronic, ultrasound, and light stimuli, can influence the behavior of nanocarriers in biological systems. They enable enhanced accumulation in specific regions (e.g., through magnetic fields), controlled release, intracellular drug delivery, and activated imaging and therapy. However, externally directed triggers may not be practical for accessing and treating metastatic lesions when their location is uncertain.172
Intrinsic stimuli occur within the target microenvironment, which has distinguishing characteristics, such as specific temperature and pH. These intrinsic stimuli were used to develop internal stimuli-responsive mediums for MOFs, including pH, redox, and ATP responsiveness.171 In general, externally stimulated nMOFs may exhibit better performance than internally stimulated ones. Designing multi-responsive MOFs may be a more effective way to solve the limitations of efficient medication delivery.
MOF-based stimuli-responsive drug delivery entails adding stimuli-responsive groups or materials to the MOF structure, such as imidazole groups (pH-responsive), disulfide bonds (GSH-responsive), porphyrins (light-responsive), and temperature-sensitive components.173 These smart materials undergo molecular structural changes, protonation, or hydrolytic cleavage when stimulated.174 Consequently, the physicochemical properties of the stimuli-responsive MOFs change under specific conditions, resulting in the release of guest molecules.173
7.1.1 ATP response. To control the growth of cancerous cells, ATP-responsive MOFs have been developed, taking advantage of the higher ATP levels in these cells compared to normal ones.175 The coordination potential between metal ions in MOFs and the lone-pair electron-rich components of ATP, such as the benzene ring, amino group, and imidazole ring, enables effective coordination interactions.176 Furthermore, ATP's ability to complex with certain metals due to the lone-pair electrons of its nitrogen atoms enhances its affinity to bind to the metal sites of MOFs, resulting in the cleavage of the MOF structure and the desired response.177 One notable example involves the creation of ATP-responsive MOFs, including RhI-DOX@ZIF-90 and RhI-DOX-GOD@ZIF-90. These MOFs encapsulate cancer treatments, such as DOX, and employ gates that respond to stimuli to release the drug payload when exposed to ATP.178,179 Another approach involves using ATP-aptamer complexes, where functionalizing MOFs with ATP-sensitive DNAs allows the unlocking of gated caps through ATP-aptamer coordination.180 This strategy provides an effective means to cap and release therapeutic agents loaded in MOFs when exposed to ATP-concentrated media.181 Nevertheless, the efficacy of the technique largely depends on the precise distinction of ATP levels between malignant and normal cells, which can vary considerably among persons and types of cancer. Furthermore, the essential difficulty lies in guaranteeing the stability and controlled release of therapeutic substances in various biological settings.
7.1.2 pH response. Creating pH-sensitive MOFs involves incorporating proton-donating/accepting groups into the coordination structure, which is particularly useful in drug delivery systems targeting acidic tumor environments. For example, a Zn-based MOF self-assembles into a 3D supermolecular structure through intermolecular hydrogen bonds and N–S interactions, while a Cd-based MOF forms undulated 2D layers with a topological network that stacks into a 3D structure via interlayer N–S interactions.182 The Zn-based MOFs exhibit fluorescent emission under UV irradiation, with quenching in acidic environments and recovery in neutral environments due to the protonation-enhanced electron-withdrawing nature of the tetrazole group. In contrast, the Cd-based MOFs display self-referencing fluorescence responses in alkaline environments due to the different proton-withdrawing capabilities of the coordinated tetrazole group.182 This technique demonstrates MOF adaptability and improves its design for actual biomedical uses, offering potential progress in targeted therapy and customized medicine.
7.1.3 Redox response. Redox-responsive MOFs are highly valuable for drug delivery systems that target different redox concentrations in tumor cells and normal tissues. GSH, a reducing agent found in higher concentrations in cancer cells, provides an interesting receptor site for designing redox-responsive drug delivery systems.183 For instance, GSH-sensitive MOFs composed of Mn2+ ions and disulfide-containing ligands were developed, where the disulfide linkage within the structure of MOFs can be cleaved in the presence of GSH, resulting in the release of the encapsulated drug. Another study focused on redox-responsive MOFs with Zr, Fe, and Al as metal nodes and a ligand containing disulfide bonds. The cleavage of disulfide bonds in tumor cells was faster compared to normal cells when exposed to GSH. Moreover, redox-responsive and tumor-targeted MOFs were constructed by utilizing functional disulfide anhydride and FA as organic links, enabling the release of drugs in response to overexpressed GSH in cancer cells.184 Additional efforts can enhance the performance of these systems for use in clinical settings by overcoming problems related to stability and scalability, thereby maximizing their therapeutic benefits.
7.1.4 Temperature response. The distinct architectures of temperature-responsive 2D MOFs have garnered substantial interest, as they contain open metal sites and specific ligands. At varying temperatures, these MOFs display various thermochromic, thermoelectric, and thermomagnetic properties.185 For example, three 2D MOFs (marked as 1·NH3, 1·NH2CH3 and 2) showed diverse thermochromic behaviors by altering chemically inactive sites in the MOF lattices, resulting in phase transitions and changes in the supramolecular microenvironments.186 Additionally, some 2D MOFs display thermoelectric and thermomagnetic behaviors. Ni3(HIB)2 and Cu3(HIB)2 MOFs exhibited metallic behavior with different electrical conductivities depending on the temperature, while FeTHT MOFs demonstrated temperature-dependent resistivities.187 The intricate relationship between the structure of MOFs and their temperature–responsive properties is highlighted. This understanding could pave the way for custom designs with the potential to revolutionize materials science and device engineering. Further studies are crucial to optimizing these MOFs for specific applications and to thoroughly understand their underlying mechanisms, enabling the full utilization of their technological capabilities.
7.1.5 Ultrasound response. Apart from light and magnetic field stimuli, ultrasound has emerged as an external stimulus for controlling drug delivery. US-responsive nano-assemblies composed of US-responsive agents and various delivery carriers have been investigated.178 Upon exposure to ultrasound waves, these nano-assemblies can be activated through cavitation, acoustic fluid streaming, pressure variation, or local hyperthermia, leading to the release of drugs. The Fe-NDC nanorods effectively encapsulated calcein and DOX, resulting in notable improvements in their release when exposed to ultrasound. The release of calcein reached up to 95.2%, while DOX achieved a release of 80% when ultrasound was applied.188 Additional studies are essential to enhance these systems for clinical use, addressing issues such as reproducibility, scalability, and compatibility, with various medication formulations and administration methods. In conclusion, ultrasound-responsive drug delivery can improve treatment outcomes through controlled release mechanisms tailored to specific physiological conditions and disease states.
7.1.6 Light response. MOFs can be designed to exhibit light–responsive properties by introducing photosensitive functional groups, such as azobenzene, into their framework. Two approaches are commonly used: filling the MOF pores with photosensitive guests or decorating the bridging ligands with photosensitive functional groups. Azobenzene is a representative photosensitive molecule that undergoes conformational changes from the trans- to the cis state under UV light irradiation.189 For example, the introduction of azobenzene molecules into the pores of flexible MOFs can induce phase transitions and modify the crystal structures and pore environments upon light stimulation.190 Photosensitive groups can be incorporated into the MOF structure, leading to changes in the MOF's properties upon light irradiation. For instance, a light-responsive MOF was developed by decorating the MOF structure with azobenzene-functionalized ligands. The reversible photoisomerization of the azobenzene groups resulted in changes in the MOF's porosity and guest uptake/release properties.191 Furthermore, these MOFs can be engineered with a selenium-polymer shell that is responsive to redox reactions. This allows for precise control over the release of drugs when exposed to laser light. Upon irradiation, the MOF core produces ROS, which results in the breaking of the polymer chain and the subsequent release of the encapsulated treatment, such as DOX. This enables a combination of chemotherapy and photodynamic therapy.192 The attainment of reliable and predictable light-responsive behavior in MOFs shows potential for the creation of advanced smart materials with customized functions for a wide range of industrial and medicinal uses.
7.1.7 Magnetic response. Magnetic-responsive MOFs exhibit the ability to respond to an external magnetic field, offering a synergistic effect for controlled drug release and targeted particle accumulation. This therapeutic approach, called magnetophoretic therapy, holds promise in DDS. Additionally, these systems can be employed in MRI to enhance the contrast in T2*-weighted images.193 Combining magnetic responsiveness with other stimuli-responsive features has been explored in certain systems. In this study, researchers developed magnetic nanocomposites that integrate magnetic hyperthermia and chemotherapy treatments. The design involved a core–shell structure comprising a Fe3O4@PDA core and a ZIF-90 nMOF shell, with a size of 200 nm. Upon cellular uptake, the DOX-loaded Fe3O4@PDA@ZIF-90 nanocomposites demonstrated remarkable efficacy in eliminating tumor cells due to the synergistic effect of magnetic hyperthermia and pH-triggered drug release.194 Magnetic-responsive MOFs have also been utilized in environmental applications. For instance, the researchers synthesized an adsorbent by immobilizing the tripeptide glutathione on the surface of magnetic MOFs (Fe3O4-ZrMOF@GSH) to remove Hg(II), Cd(II), and Pb(II) from wastewater.195 Emerging stimuli-responsive MOF materials have demonstrated suitability for various applications, such as ion adsorbents, CO2 capture materials, drug delivery systems, and ion-selective separation membranes. Various synthetic methods, such as sol–gel, MOF seed induction, electrospinning, core–shell, porous carrier-functional group, nanofiber polymerization, and in situ polymerization, have been developed to achieve the desired response properties of stimuli-responsive materials. MOF materials responsive to different structural stimuli exhibit distinct properties, enabling more efficient and rapid responses to specific external and internal triggers.196Overall, MOFs may be constructed to respond to a wide range of stimuli, including ATP, pH, redox conditions, temperature, ultrasound, light, and magnetic fields. MOFs are interesting prospects for drug delivery systems due to their sensitive features, which allow for the regulated release of encapsulated drugs or therapeutic agents. The design and mechanism of MOFs for each stimulus might differ depending on the application and target environment.
7.2 Multifunctional MOF nanocarriers for imaging and therapy
With the increase in personalized cancer treatments, the development of advanced imaging modalities for cancer diagnosis is crucial. The integration of diagnosis and treatment has become a clinical trend, and phototheranostic nanomedicine is a promising approach to bridging this gap.197 Various non-invasive in vivo imaging techniques, including MRI, SPECT, CT, PET, and optical imaging, have been developed to visualize the progression of abnormal cells by targeting specific sites.198
7.2.1 MOF nanocarriers for imaging. In bioimaging, MOF-based nanocomposites have gained significant attention due to their facile functionalization, diverse structures and compositions, and large porosities. These nanocomposites are widely used in fluorescence imaging (FL), CT, MRI, and PET imaging by incorporating fluorescent small molecules and imaging contrast agents.199 MOFs can serve as matrices for the attachment of specific materials, enhancing optical, chemical, or electrical signals for bioimaging applications.200 Additionally, certain metal ions can confer imaging functions to nMOFs, such as MRI and CT, while the porous structures and active sites on the surface enable the loading of fluorescent molecules for high-sensitivity imaging. Organic ligands with fluorescence properties can also be directly assembled with metal ions to achieve multiple imaging functions in single nMOFs.201MOFs have shown promise in imaging techniques via many studies that have highlighted their flexibility and convenience. Jia et al. used Gd/DTPA/MOF-808/PANI to perform magnetic resonance bioimaging of 4T1 breast cancer cells. They achieved an R1 elongation of 30.1 mM−1 s−1 (0.5 T).202 Zhu et al. conducted a separate investigation in which they created Fe-DOX@Gd-MOFs-ICG using Gd3+ as a contrast agent. This allowed for the effective implementation of photoacoustic and photothermal imaging techniques.200 These examples demonstrate the use of MOFs in photoacoustic and photothermal imaging treatment, which helps advance the development of MOF-based nano-hybrid materials in MRI.203
Furthermore, recent findings have demonstrated that MOFs have potential as contrast agents, providing a high level of intensity while minimizing disadvantages. Bao et al. produced Hf/Mn-TCPP-MOF, which exhibited a significant ability to absorb X-rays and showed promise for use in highly sensitive CT scanning.204 In addition, Ma et al. produced NMOF545@Pt by demonstrating a CT value of 110 HU. The increased concentration of Pt in these MOFs may amplify signals in CT and emphasize its potential in MRI, CT, and PAI imaging modalities.205
Zhang et al. developed MOF@ICG@DOX, which was created from Zn2+ and 2-methylimidazole. ICG was loaded into the pores of the MOFs for photoacoustic and FL. Nevertheless, their research did not address the methods for removing MOFs containing ICG from the body, maybe via the excretion of urine.206 In addition, Yuan et al. introduced a fluorescent probe called DOX-Gd-TCPP-MOF, which can diagnose 4T1 cells. The study also showed its potential for anti-tumor therapy and fluorescence treatment in FL imaging.207 Overall, MOFs demonstrate great flexibility and provide substantial potential for future testing and diagnostic engineering projects.
7.2.2 MOF nanocarriers for therapy. In terms of therapeutic applications, nMOFs can be built similarly to those of imaging applications. Certain metal ions possess therapeutic effects, such as those used in radiotherapy, while organic ligands, such as porphyrins and their derivatives, have PDT capabilities. By selecting appropriate metal ions and ligands, functional nMOF-based systems can be constructed, integrating diagnosis and treatment.201 Moreover, MOFs exhibit synergistic effects when combined with various therapeutic agents, enabling combination therapies, such as chemodynamic therapy (CDT) and gene therapy activation. Some highly potent MOFs can even deliver specific therapeutic effects individually, such as microwave therapy and phototherapy.208 An example of MOFs used in bioimaging and therapy include UiO-66, a zirconium-based MOF that offers drug-loading capabilities and functional group conjugation. Additionally, MOFs-5, a zinc-based MOF, possesses unique properties and can be substituted with other metal ions to modulate immune stimulation signals and improve cancer immunotherapy efficacy. Moreover, MOF-74, an MN-based MOF, can be modified with metal NPs to amplify electrochemical sensing signals for sensitive cancer biomarkers.209Although nMOFs show promise in bioimaging, their use can be limited by the toxicity associated with certain metal ions. For example, the toxicity of Gd3+ and Mn2+ has restricted their widespread clinical use in MRI due to their irreversible binding to serum proteins, and Gd3+ may lead to brain deposits.210 In addition, it becomes challenging to separate the diagnostic and therapeutic properties because the same stimulus used for diagnosis can produce multiple signals and simultaneously exhibit a therapeutic effect.211 Additionally, although nMOFs are commonly studied in phototherapy, other therapies, such as microwave therapy and ultrasound therapy, are still in their early stages due to the uncertainty surrounding their mechanisms of action.201
7.3 Combining MOFs with other nanomaterials for enhanced efficacy
MOFs have been combined with various other nanomaterials to improve their characteristics and efficacy in a wide range of applications. For example, Kim et al. created a photoactive MOF-derived cobalt-silver bimetallic nanocomposite (Ag@Co-MOFs) with significant antibacterial properties and a photothermal transformation effect.212 Mo et al. developed MnO2@NH2-MIL101(Fe)@Ce6-F127 nanoparticles, which incorporated the Fenton-like effects of Fe-based MOFs with MnO2 tumor microenvironment-responsive features to improve CDT and PDT.213 Multifunctional cancer theranostic drugs can be manufactured by mixing MOFs with other nanoparticles, including molybdenum disulfide. These nanoplatforms have suitable photothermal characteristics, pH/near-infrared (NIR) laser-triggered drug release, and tumor cell targeting ability.214 Furthermore, the use of MOFs and magnetic NPs has led to the development of hierarchically porous nano-objects that have significant colloidal stability, biodegradability, and drug-loading capacity. These nanoobjects have demonstrated promise as drug delivery platforms for cancer therapy and as effective contrast materials for MRI.215 These findings show the potential for combining MOFs with other nanomaterials to improve efficacy in various diagnostic and therapeutic applications. Table 1 illustrates a comparison of various MOF materials for different therapeutic agents. Table 2 lists the synthesis methods, structural and morphological properties of different MOFs, and their modes of application in biomedical research.
Table 1 Comparison of various MOF materials for different therapeutic agents
MOFs |
Organic linker |
Therapeutic agents |
Advantages |
Ref. |
CS/Bio-MOFs-13-Co |
— |
DOX |
Bonds cleavable at specific pH |
216 |
CNTs |
— |
DOX |
Selective drug accumulation |
217 |
Hollow MSNs |
— |
DOX |
Anti-proliferative |
218 |
MIL-88A (Fe) |
Fumaric acid |
Ibuprofen and cidofovir |
Good relaxivities and biodegradable |
219 |
FA-PEG/CQ@ZIF-8 |
Methoxy poly(ethylene glycol)-folate (FA-PEG) |
PHY |
Anti-microbial effect |
220 |
Autophagy inhibitor |
Cu-MOFs |
1,3,5-Benzenetricarboxylic acid |
Ibuprofen |
High thermal stability |
221 |
Catalytic activity |
DOX@ZIF-8 NTs |
— |
DOX |
High level of drug loading |
222 |
BSA/Cu/NQ NP |
— |
— |
Active targeting therapy |
223 |
UiO-66-NH2/NO2 |
1,4-Benzenedicarboxylic acid |
Ketoprofen |
— |
224 |
CoFe2O4@PDA@ZIF-8 |
2-Methylimidazole (2-MIM) |
DOX + CPT |
Promoted cellular uptake |
225 |
Greater growth inhibition against cancer cells |
ZIF-8 |
2-Methylimidazole (2-MIM) |
DOX |
pH-responsive release |
226 |
ZIF-8 |
2-Methylimidazole (2-MIM) |
DOX + BSA |
Anti-cancer effect |
227 |
CD-MOF |
γ-Cyclodextrin |
Ibuprofen and lansoprazo |
Sustained drug release |
228 |
Table 2 Synthesis methods, structural and morphological properties of different MOFs and their mode of application in biomedical research
MOFs |
Synthesis method |
Structural and morphological properties |
Drug delivery applications |
Ref. |
Cu-BDC MOFs |
Solvothermal |
• Irregularly shaped flakes |
• Carrier of antibacterial agents |
229 |
• Monoclinic crystal system |
• Negative surface charges |
• Size 25 nm |
MOF-74-M |
Mechanochemical |
• High density of open metal sites |
• Drug delivery host in cancer treatment, which exhibits a very high drug-loading capacity of ibuprofen anions |
230 and 231 |
• Hexagonal channels along the c-axis |
• High porosity |
• Relatively large pore sizes |
Fe-MOF-5-NH2 |
Hydrothermal |
• Hollow octahedral |
• Strong green fluorescence, pH-controlled 5-FU release, and used in cancer diagnostic and therapy |
232 and 233 |
• Nanostructures with a particle size of ∼200 nm |
Zn MOF-74 |
Solvothermal |
• Crystalline structure |
• Delivery system of ibuprofen and used as drug delivery in cancer therapy |
234 and 235 |
• Pore range diameters of 3, 8.5, and 13 nm |
Zr-MOF UiO-66 |
Solvothermal |
• Size of 50–80 nm |
• Delivery of DOX for hepatocellular carcinoma |
236 and 237 |
• Zeta potential of +40 ± 1 mV |
• Cuboctahedra structure |
• High surface area |
• Thermal stability |
• Resistant to outside pressures |
PCN-222 MOF |
One-pot microwave-assisted synthesis |
• Uniform ricelike nanorods of 301 ± 17 nm in length and 96 ± 9 nm in diameter |
• DNA-mediated nanoscale PCN-222 immunoassay for prostate-specific antigen detection |
236 and 238 |
• Absorbs light and concentrates oxygen and dopamine molecules around active TCPP ligands |
MOF-808 |
Solvothermal |
• Octahedral morphology |
• Dual drug delivery of floxuridine and carboplatin loaded in MOF-808 and highly selective DDS in cancer cells and enhance the therapeutic efficacy of chemotherapy |
124 |
• Surface area of 722 m2 g−1 |
• Average particle size of 113.7 ± 25.9 |
MOFs-53(Fe) |
Solvothermal |
• Octahedral structure |
• Carrier for antibacterial drug (vancomycin) |
239 |
• Surface area of MOFs-53(Fe) and MOFs-53(Fe)@Van were 83.76 and 10.338 m2 g−1, respectively |
• The pore size of the MOFs-53(Fe) = 1.688 nm |
MTD@MOF5 |
Deprotonation regulation synthesis |
• Thermal behavior and properties in the range of 25–600 °C |
• Delivery vehicle for an antibiotic drug |
240 |
• The size of MOFs-5 and MTD@MOF5 nanocomposite was found of about 40–90 nm and up to 100 nm, respectively |
• TEM micrograph of MOFs-5 shows black dots |
8. Future perspectives and emerging trends
By integrating medical, genetic, and epigenetic data, personalized medicine (PM) seeks to customize treatment to meet the unique health requirements of each patient. Improving health and longevity while decreasing healthcare expenditures is an extension of the One-Size-Fits-All approach. It predicts which medical treatments will be safe and effective for certain people.241 The use of existing biomarkers in conjunction with early genome and epigenomic processes allows for the early detection of PM, which includes carcinogenesis. With PM's focus on preventive medicine, we can reduce the financial burden and improve quality of life by warding off potential issues before they even start.241 Drugs delivered via NPs, such as MOFs, allow for targeted or tissue-selective treatment, and they can also serve as imaging agents for diagnostic purposes. Nanomaterials with two distinct but complementary uses can transport drugs and diagnose medical conditions. By facilitating the tracking of medication release, biodistribution, dosage modification, and illness progression, this integrated method has significant prospects for the advancement of personalized medicine.242 Bioinspired design approaches show significant promise for developing the field of next-generation MOFs. These approaches, inspired by nature's impressive flexibility and efficiency, seek to incorporate crucial characteristics and principles found in biological systems into the design and synthesis of MOFs. Researchers can improve the effectiveness and versatility of MOFs for a wide range of applications by mimicking the structural and functional characteristics of biological materials, such as enzymes, proteins, and DNA. Bioinspired approaches for designing various techniques, including templating, self-assembly, and hierarchical structure, to produce MOFs with customized features, such as enhanced stability, selectivity, and catalytic activity, using the inherent benefits associated with biological systems. Furthermore, MOFs have demonstrated significant promise in the fields of precision medicine and theranostic platforms for cancer detection and therapy. MOFs have versatile physicochemical features, such as particular cellular targeting, customizable pore and particle size, high surface area, and drug-loading capacity, making them appropriate for both targeted imaging and therapy. MOFs are excellent drug carriers. However, at present, research on the biomedical performance of MOFs has been limited to small-scale production and laboratory experiments. MOFs can be combined with diagnostic and therapeutic features, resulting in the development of theragnostic platforms. In the future, these systems will enable continuous evaluation of treatment response and the adjustment of therapy in real time. Future efforts will involve the advancement of MOFs that possess integrated theragnostic characteristics designed for personalized medicine.
When MOFs are produced in large quantities, it can be challenging to control their quality, which can cause fluctuations in the pore size and material size. Additionally impacted are the drug loading capacity and release rate. Therefore, a major difficult issue is the construction of stable and manageable MOFs. Overall, significant advances from fabrication to quality monitoring focus on quantitative quality control strategies for MOF contents, and in vivo long-term studies are required to develop appropriate MOFs as drug carriers for clinical applications. These developments are expected to be achieved in the future.
9. Conclusion
In conclusion, this review article focused on the potential of MOFs as smart drug delivery systems in the treatment of various types of diseases, such as cancers. In addition, MOFs play an important role in fighting the worldwide threat of antiviral diseases and antibiotic resistance. Furthermore, MOFs can serve as agents for the controlled release of anti-bacterial components. It has been obvious that MOFs have unique characteristics that make them attractive for targeted drug delivery applications. For instance, the unique structural properties of MOFs, such as their high porosity and large surface area, enable the efficient encapsulation, storage, and regulated release of therapeutic compounds. This ensures that drugs are administered in a precise and controlled manner, maximizing efficacy while limiting adverse effects. Then, the flexible nature of MOFs also provided the incorporation of functional groups, resulting in versatile drug carriers for various types of drugs. By developing the surface properties of MOFs, researchers can improve drug stability, solubility, and bioavailability, and thus enhance therapeutic effects. Furthermore, MOFs can respond to external stimuli, such as pH, temperature, light, and electrical fields, allowing for the controlled release of drugs at specific target areas in the body. This responsive reaction improves the precision and selectivity of drug administration, lowering off-target effects and systemic toxicity. Overall, MOFs have shown significant potential as smart drug delivery systems for the treatment of a wide range of diseases. However, more studies are needed to address these challenges, including scaling up manufacturing, long-term stability, and clinical translation. With continuous advances in MOF synthesis, characterization techniques, and an understanding of their interactions with biological systems, MOFs are expected to play a crucial role in enhancing the administration of drugs and improving patient outcomes in the future.
Data availability
No primary research results, software or code are included and no new data were generated or analysed as part of this review.
Conflicts of interest
There are no conflicts to declare.
References
- H. Park, A. Otte and K. Park, J. Controlled Release, 2022, 342, 53–65 CrossRef CAS PubMed.
- H. Reza Rezaie, M. Esnaashary, A. Aref Arjmand and A. Öchsner, in A Review of Biomaterials and Their Applications in Drug Delivery, Springer Singapore, Singapore, 2018, pp. 1–8 Search PubMed.
- W. Zhang, Q. Zhao, J. Deng, Y. Hu, Y. Wang and D. Ouyang, Drug Discovery Today, 2017, 22, 1201–1208 CrossRef CAS PubMed.
- A. A. Ghawanmeh, L. L. Tan, G. A. M. Ali, M. A. Assiri and K. F. Chong, J. Mol. Liq., 2024, 393, 123631 CrossRef CAS.
- T. C. Ezike, U. S. Okpala, U. L. Onoja, C. P. Nwike, E. C. Ezeako, O. J. Okpara, C. C. Okoroafor, S. C. Eze, O. L. Kalu, E. C. Odoh, U. G. Nwadike, J. O. Ogbodo, B. U. Umeh, E. C. Ossai and B. C. Nwanguma, Heliyon, 2023, 9, e17488 CrossRef CAS PubMed.
- M. P. B. Abundo, Ultrasound Controlled Drug Delivery by Acoustically Switchable Hydrogels, PhD Dissertation, California Institute of Technology, 2023.
- A. A. Ghawanmeh, G. A. M. Ali, H. Algarni, S. M. Sarkar and K. F. Chong, Nano Res., 2019, 12, 973–990 CrossRef CAS.
- D. Elfadil, G. S. El-Sayyad and G. A. M. Ali, in Handbook of Nanosensors, ed. G. A. M. Ali, K. F. Chong and A. S. H. Makhlouf, Springer Nature Switzerland, Cham, 2024, pp. 1–36 Search PubMed.
- H. D. Lawson, S. P. Walton and C. Chan, ACS Appl. Mater. Interfaces, 2021, 13, 7004–7020 CrossRef CAS.
- S. Gautam, I. Lakhanpal, L. Sonowal and N. Goyal, Nanotechnol, 2023, 3–4, 100027 Search PubMed.
- S. Mallakpour, E. Nikkhoo and C. M. Hussain, Coord. Chem. Rev., 2022, 451, 214262 CrossRef CAS.
- M. Fytory, K. K. Arafa, W. M. A. El Rouby, A. A. Farghali, M. Abdel-Hafiez and I. M. El-Sherbiny, Sci. Rep., 2021, 11, 19808 CrossRef CAS.
- H. I. Adil, M. R. Thalji, S. A. Yasin, I. A. Saeed, M. A. Assiri, K. F. Chong and G. A. M. Ali, RSC Adv., 2022, 12, 1433–1450 RSC.
- S. Kumar, C. Chauhan, R. Kumar, N. Kalra, A. Saini, S. Sharma and A. Singh, Results Chem., 2023, 6, 101206 CrossRef CAS.
- Y. Sun, L. Zheng, Y. Yang, X. Qian, T. Fu, X. Li, Z. Yang, H. Yan, C. Cui and W. Tan, Nano-Micro Lett., 2020, 12, 103 CrossRef CAS PubMed.
- B. Maranescu and A. Visa, Int. J. Mol. Sci., 2022, 23, 4458 CrossRef CAS PubMed.
- V. F. Yusuf, N. I. Malek and S. K. Kailasa, ACS Omega, 2022, 7, 44507–44531 CrossRef CAS.
- T. D. Bennett, F.-X. Coudert, S. L. James and A. I. Cooper, Nat. Mater., 2021, 20, 1179–1187 CrossRef CAS PubMed.
- S. He, L. Wu, X. Li, H. Sun, T. Xiong, J. Liu, C. Huang, H. Xu, H. Sun, W. Chen, R. Gref and J. Zhang, Acta Pharm. Sin. B, 2021, 11, 2362–2395 CrossRef CAS PubMed.
- L. Hou, Y. Qin, J. Li, S. Qin, Y. Huang, T. Lin, L. Guo, F. Ye and S. Zhao, Biosens. Bioelectron., 2019, 143, 111605 CrossRef CAS.
- Y. He, W. Zhang, T. Guo, G. Zhang, W. Qin, L. Zhang, C. Wang, W. Zhu, M. Yang, X. Hu, V. Singh, L. Wu, R. Gref and J. Zhang, Acta Pharm. Sin. B, 2019, 9, 97–106 CrossRef.
- X. Leng, X. Dong, W. Wang, N. Sai, C. Yang, L. You, H. Huang, X. Yin and J. Ni, Molecules, 2018, 23(10), 2490 CrossRef PubMed.
- X. Liu, T. Liang, R. Zhang, Q. Ding, S. Wu, C. Li, Y. Lin, Y. Ye, Z. Zhong and M. Zhou, ACS Appl. Mater. Interfaces, 2021, 13, 9643–9655 CrossRef CAS.
- J. H. Cavka, S. Jakobsen, U. Olsbye, N. Guillou, C. Lamberti, S. Bordiga and K. P. Lillerud, J. Am. Chem. Soc., 2008, 130, 13850–13851 CrossRef PubMed.
- D. B. N. Lee, M. Roberts, C. G. Bluchel and R. A. Odell, ASAIO J., 2010, 56, 550–556 CrossRef CAS PubMed.
- Z. Li, S. Zhao, H. Wang, Y. Peng, Z. Tan and B. Tang, Colloids Surf., B, 2019, 178, 1–7 CrossRef CAS PubMed.
- S. Rojas, F.J. Carmona, C.R. Maldonado, P. Horcajada, T. Hidalgo, C. Serre, J.A.R. Navarro and E. Barea, Inorg. Chem., 2016, 55(5), 2650–2663 CrossRef CAS PubMed.
- H. Shayegan, G. A. M. Ali and V. Safarifard, J. Inorg. Organomet. Polym. Mater., 2020, 30, 3170–3178 CrossRef CAS.
- P.P. Bag, D. Wang, Z. Chen and R. Cao, Chem. Commun., 2016, 52, 3669–3672 RSC.
- Q. Sun, H. Bi, Z. Wang, C. Li, X. Wang, J. Xu, H. Zhu, R. Zhao, F. He, S. Gai and P. Yang, Biomaterials, 2019, 223, 119473 CrossRef CAS PubMed.
- P. Horcajada, R. Gref, T. Baati, P. K. Allan, G. Maurin, P. Couvreur, G. Férey, R. E. Morris and C. Serre, Chem. Rev., 2012, 112, 1232–1268 CrossRef CAS PubMed.
- F. Liang, D. Ma, L. Qin, Q. Yu, J. Chen, R. Liang, C. Zhong, H. Liao and Z. Peng, Dalton Trans., 2024, 53, 10070–10074 RSC.
- J. Wei, Y. Chen, H. Zhang, Z. Zhuang and Y. Yu, Chin. J. Catal., 2021, 42, 78–86 CrossRef CAS.
- J. Martí-Rujas, Dalton Trans., 2020, 49, 13897–13916 RSC.
- S. Torresi, A. Famulari and J. Martí-Rujas, J. Am. Chem. Soc., 2020, 142, 9537–9543 CrossRef CAS.
- P. T. Phan, J. Hong, N. Tran and T. H. Le, Nanomaterials, 2023, 13, 352 CrossRef CAS PubMed.
- Elizbit, U. Liaqat, Z. Hussain, M. M. Baig, M. A. Khan and D. Arif, J. Korean Ceram. Soc., 2021, 58, 598–605 CrossRef CAS.
- T. T. T. Nguyen, L. H. T. Nguyen, N. X. D. Mai, H. K. T. Ta, T. L. T. Nguyen, U.-C. N. Le, B. T. Phan, N. N. Doan and T. L. H. Doan, J. Drug Delivery Sci. Technol., 2021, 61, 102135 CrossRef CAS.
- A. Ghoorchian, A. Afkhami, T. Madrakian and M. Ahmadi, in Metal-Organic Frameworks for Biomedical Applications, Elsevier, 2020, pp. 177–195 Search PubMed.
- W.-J. Li, M. Tu, R. Cao and R. A. Fischer, J. Mater. Chem. A, 2016, 4, 12356–12369 RSC.
- M. G. Goesten, P. C. M. M. Magusin, E. A. Pidko, B. Mezari, E. J. M. Hensen, F. Kapteijn and J. Gascon, Inorg. Chem., 2014, 53, 882–887 CrossRef CAS.
- A. Kumar, A. Sharma, Y. Chen, M. M. Jones, S. T. Vanyo, C. Li, M. B. Visser, S. D. Mahajan, R. K. Sharma and M. T. Swihart, Adv. Funct. Mater., 2021, 31, 2008054 CrossRef CAS.
- H. Liu, H. Wang, T. Chu, M. Yu and Y. Yang, J. Mater. Chem. C, 2014, 2, 8683–8690 RSC.
- Q. Zhang, Z. Wu, Y. Lv, Y. Li, Y. Zhao, R. Zhang, Y. Xiao, X. Shi, D. Zhang, R. Hua, J. Yao, J. Guo, R. Huang, Y. Cui, Z. Kang, S. Goswami, L. Robison, K. Song, X. Li, Y. Han, L. Chi, O. K. Farha and G. Lu, Angew. Chem., Int. Ed., 2019, 58, 1123–1128 CrossRef CAS PubMed.
- I. Malytska, C. Mézière, M. Kielar, L. Hirsch, G. Wantz, N. Avarvari, A. Kuhn and L. Bouffier, J. Phys. Chem. C, 2017, 121, 12921–12927 CrossRef CAS.
- M. Leonardi, M. Villacampa and J. C. Menéndez, Chem. Sci., 2018, 9, 2042–2064 RSC.
- Z. Nadizadeh, M. R. Naimi-Jamal and L. Panahi, J. Solid State Chem., 2018, 259, 35–42 CrossRef CAS.
- Z. Li, J. Dong, H. Zhang, Y. Zhang, H. Wang, X. Cui and Z. Wang, Nanoscale Adv., 2021, 3, 41–72 RSC.
- Z. Li, J. Dong, L. Wang, Y. Zhang, T. Zhuang, H. Wang, X. Cui and Z. Wang, Nanoscale Adv., 2021, 3, 2423–2447 RSC.
- S. A. Hwangbo, M. Kwak, J. Kim and T. G. Lee, Nanomaterials, 2021, 11, 427 CrossRef CAS PubMed.
- S. Głowniak, B. Szczęśniak, J. Choma and M. Jaroniec, Molecules, 2023, 28, 2639 CrossRef PubMed.
- F. Parsa, M. Setoodehkhah and S. M. Atyabi, Inorg. Chem. Commun., 2023, 155, 111056 CrossRef CAS.
- Z. S. Rozveh, S. Kazemi, M. Karimi, G. A. M. Ali and V. Safarifard, Polyhedron, 2020, 183, 114514 CrossRef CAS.
- H. Shayegan, G. A. M. Ali and V. Safarifard, ChemistrySelect, 2020, 5, 124–146 CrossRef CAS.
- T. Chen and D. Zhao, Coord. Chem. Rev., 2023, 491, 215259 CrossRef CAS.
- L. Figueroa-Quintero, D. Villalgordo-Hernández, J. J. Delgado-Marín, J. Narciso, V. K. Velisoju, P. Castaño, J. Gascón and E. V. Ramos-Fernández, Small Methods, 2023, 7, 2201413 CrossRef CAS.
- J. Mehta, N. Bhardwaj, S. K. Bhardwaj, K.-H. Kim and A. Deep, Coord. Chem. Rev., 2016, 322, 30–40 CrossRef CAS.
- S. Wang, X. Yang, W. Lu, N. Jiang, G. Zhang, Z. Cheng and W. Liu, J. Drug Delivery Sci. Technol., 2021, 64, 102593 CrossRef CAS.
- L. B. Vaidya, S. S. Nadar and V. K. Rathod, Colloids Surf., B, 2020, 193, 111052 CrossRef CAS PubMed.
- Y. Liu, G. Yang, S. Jin, L. Xu and C. Zhao, ChemPlusChem, 2020, 85, 2143–2157 CrossRef CAS.
- S. Bharti, G. Kaur, S. Jain, S. Gupta and S. K. Tripathi, J. Drug Targeting, 2019, 27, 813–829 CrossRef CAS PubMed.
- M.-L. Laracuente, M. H. Yu and K. J. McHugh, J. Controlled Release, 2020, 327, 834–856 CrossRef CAS.
- S. D. Taherzade, S. Rojas, J. Soleimannejad and P. Horcajada, Nanomaterials, 2020, 10, 2296 CrossRef CAS.
- R. Gouda, H. Baishya and Z. Qing, J. Dev. Drugs, 2017, 6, 171–178 Search PubMed.
- S. Bhattacharjee, Ther. Delivery, 2021, 12, 21–36 CrossRef CAS.
- Y. Wang, J. Yan, N. Wen, H. Xiong, S. Cai, Q. He, Y. Hu, D. Peng, Z. Liu and Y. Liu, Biomaterials, 2020, 230, 119619 CrossRef CAS PubMed.
- A. Parmar and S. Sharma, TrAC, Trends Anal. Chem., 2018, 100, 15–35 CrossRef CAS.
- W. Korsmeyer, R. Gurny, E. Doelker, P. Buri and N.A. Peppas, Int. J. Pharm., 1983, 15(1), 25–35 CrossRef.
- M. Pourmadadi, Z. Omrani, Z. Forootan, M. S. Ebadi and F. Yazdian, J. Drug Delivery Sci. Technol., 2023, 86, 104690 CrossRef CAS.
- Y. Zhang, A. R. Khan, X. Yang, M. Fu, R. Wang, L. Chi and G. Zhai, J. Drug Delivery Sci. Technol., 2021, 61, 102266 CrossRef CAS.
- M. Shoaib, I. Siddiqui, Y. M. Amir and S. U. Rehman, Renewable Sustainable Energy Rev., 2017, 70, 1343–1351 CrossRef.
- S. Gautam, I. Lakhanpal, L. Sonowal and N. Goyal, Nanotechnol, 2023, 3–4, 100027 Search PubMed.
- R. Masoudifar, N. Pouyanfar, D. Liu, M. Ahmadi, B. Landi, M. Akbari, S. Moayeri-Jolandan, F. Ghorbani-Bidkorpeh, E. Asadian and M.-A. Shahbazi, Appl. Mater. Today, 2022, 29, 101646 CrossRef.
- F. Afshariazar and A. Morsali, J. Mater. Chem. C, 2021, 9, 12849–12858 RSC.
- F. Salahpour Anarjan, Nano-Struct. Nano-Objects, 2019, 19, 100370 CrossRef CAS.
- Q. Cheng, F. Gao, W. Yu, M. Zou, X. Ding, M. Li, S. Cheng and X. Zhang, Adv. Funct. Mater., 2020, 30, 2000335 CrossRef CAS.
- X. Qi, Z. Chang, D. Zhang, K. J. Binder, S. Shen, Y. Y. S. Huang, Y. Bai, A. E. H. Wheatley and H. Liu, Chem. Mater., 2017, 29, 8052–8056 CrossRef CAS.
- K. Alt, F. Carraro, E. Jap, M. Linares-Moreau, R. Riccò, M. Righetto, M. Bogar, H. Amenitsch, R. A. Hashad, C. Doonan, C. E. Hagemeyer and P. Falcaro, Adv. Mater., 2022, 34, 2106607 CrossRef CAS.
- Z. Jiang, J. Guan, J. Qian and C. Zhan, Biomater. Sci., 2019, 7, 461–471 RSC.
- H. N. Abdelhamid, M. Dowaidar, M. Hällbrink and Ü. Langel, Microporous Mesoporous Mater., 2020, 300, 110173 CrossRef CAS.
- W.-H. Chen, S. Yang Sung, M. Fadeev, A. Cecconello, R. Nechushtai and I. Willner, Nanoscale, 2018, 10, 4650–4657 RSC.
- Q. Zhao, J. Li, B. Wu, Y. Shang, X. Huang, H. Dong, H. Liu, W. Chen, R. Gui and X. Nie, ACS Appl. Mater. Interfaces, 2020, 12, 22687–22701 CrossRef CAS.
- O. K. Akeremale, O. T. Ore, A. A. Bayode, H. Badamasi, J. Adedeji Olusola and S. S. Durodola, Results Chem., 2023, 5, 100866 CrossRef CAS.
- P. Hirschle, T. Preiß, F. Auras, A. Pick, J. Völkner, D. Valdepérez, G. Witte, W. J. Parak, J. O. Rädler and S. Wuttke, CrystEngComm, 2016, 18, 4359–4368 RSC.
- P. Behera, S. Subudhi, S. P. Tripathy and K. Parida, Coord. Chem. Rev., 2022, 456, 214392 CrossRef CAS.
- M. Jouyandeh, F. Tikhani, M. Shabanian, F. Movahedi, S. Moghari, V. Akbari, X. Gabrion, P. Laheurte, H. Vahabi and M. R. Saeb, J. Alloys Compd., 2020, 829, 154547 CrossRef CAS.
- T. Petit and L. Puskar, Diamond Relat. Mater., 2018, 89, 52–66 CrossRef CAS.
- B. Liu, K. Vellingiri, S.-H. Jo, P. Kumar, Y. S. Ok and K.-H. Kim, Nano Res., 2018, 11, 4441–4467 CrossRef CAS.
- P. Sinha, A. Datar, C. Jeong, X. Deng, Y. G. Chung and L.-C. Lin, J. Phys. Chem. C, 2019, 123, 20195–20209 CrossRef CAS.
- M. Naderi, in Progress in Filtration and Separation, Elsevier, 2015, pp. 585–608 Search PubMed.
- M. R. Bhambhani, P. A. Cutting, K. S. W. Sing and D. H. Turk, J. Colloid Interface Sci., 1972, 38, 109–117 CrossRef CAS.
- D. Dollimore, P. Spooner and A. Turner, Surf. Technol., 1976, 4, 121–160 CrossRef CAS.
- M. Naderi, in Progress in Filtration and Separation, Elsevier, 2015, pp. 585–608 Search PubMed.
- S. Brunauer, P. H. Emmett and E. Teller, J. Am. Chem. Soc., 1938, 60, 309–319 CrossRef CAS.
- K. S. W. Sing, in Adsorption by Powders and Porous Solids, Elsevier, 2014, pp. 237–268 Search PubMed.
- F. Ambroz, T. J. Macdonald, V. Martis and I. P. Parkin, Small Methods, 2018, 2, 1800173 CrossRef.
- J. Rouquerol, P. Llewellyn and F. Rouquerol, in Studies in Surface Science and Catalysis, Elsevier, 2007, vol. 160, pp. 49–56 Search PubMed.
- K. S. Walton and R. Q. Snurr, J. Am. Chem. Soc., 2007, 129, 8552–8556 CrossRef CAS.
- D. S. R. Khafaga, A. M. El-Khawaga, R. A. Elfattah Mohammed and H. K. Abdelhakim, Mol. Biol. Rep., 2023, 50, 10365 CrossRef CAS.
- D. S. R. Khafaga, M. M. Eid, M. H. Mohamed, M. D. E. Abdelmaksoud, M. Afify, A. M. El-Khawaga and H. K. Abdelhakim, Sci. Rep., 2024, 14, 15538 CrossRef CAS.
- D. S. Rashwan, M. M. Abd El Hamed, M. D. El-deen, M. M. Afify, M. H. Mohamed, A. E. R. F. Mohamed, R. A. Nagy and H. K. A. Elhakim, Egypt. J. Chem., 2022, 65, 197–207 Search PubMed.
- R. A. A. Nagy, H. K. A. Elhakim, M. H. Mohamed, M. Afify, M. D. E. Abd El-Maksoud, A. E. R. F. Mohamed, D. S. R. Khafaga and M. M. Eid, Egypt. J. Chem., 2022, 65, 1477–1487 Search PubMed.
- M. R. Saeb, N. Rabiee, F. Seidi, B. Farasati Far, M. Bagherzadeh, E. C. Lima and M. Rabiee, J. Bioresour. Bioprod., 2021, 6, 215–222 CrossRef CAS.
- D. S. R. Khafaga and E. F. Ewies, Egypt. J. Chem., 2023, 66, 269–279 Search PubMed.
- J. Yang and Y. Yang, VIEW, 2020, 1, e20 CrossRef.
- L. Zhang, C. Mo, Y. Huang and Z. Liu, Part. Part. Syst. Charact., 2019, 36, 1800355 CrossRef.
- D. Li, X. Yang and D. Yan, ACS Appl. Mater. Interfaces, 2018, 10, 34377–34384 CrossRef CAS.
- J. Yoo, C. Park, G. Yi, D. Lee and H. Koo, Cancers, 2019, 11, 640 CrossRef CAS.
- G. Gocheva and A. Ivanova, Mol. Pharm., 2019, 16, 3293–3321 CrossRef CAS.
- T. Yan, J. He, R. Liu, Z. Liu and J. Cheng, Carbohydr. Polym., 2020, 231, 115706 CrossRef CAS PubMed.
- D. F. Sava Gallis, K. S. Butler, J. O. Agola, C. J. Pearce and A. A. McBride, ACS Appl. Mater. Interfaces, 2019, 11, 7782–7791 CrossRef CAS PubMed.
- J. Yang and Y. Yang, Small, 2020, 16, 1906846 CrossRef CAS PubMed.
- S. Yuan, L. Feng, K. Wang, J. Pang, M. Bosch, C. Lollar, Y. Sun, J. Qin, X. Yang, P. Zhang, Q. Wang, L. Zou, Y. Zhang, L. Zhang, Y. Fang, J. Li and H. Zhou, Adv. Mater., 2018, 30, 1704303 CrossRef.
- Y. Wu, X. He, X. Wang, J. Xv, M. Muddassir, I. A. Ansari and A. Zhong, Inorg. Chim. Acta, 2024, 568, 122115 CrossRef CAS.
- Y. Li, Y. Fang, Z. Cao, N. Li, D. Chen, Q. Xu and J. Lu, Appl. Catal., B, 2019, 250, 150–162 CrossRef CAS.
- C.-B. Liu, Y.-N. Gong, Y. Chen and H.-L. Wen, Inorg. Chim. Acta, 2012, 383, 277–286 CrossRef CAS.
- D. H. Kim, J. C. Park, G. E. Jeon, C. S. Kim and J. H. Seo, Biotechnol. Bioprocess Eng., 2017, 22, 210–217 CrossRef CAS.
- D. S. R. Khafaga, M. G. Radwan, G. Muteeb, M. Aatif and M. Farhan, Catalysts, 2023, 13, 1448 CrossRef CAS.
- D. S. R. Khafaga, G. Muteeb, A. Elgarawany, M. Aatif, M. Farhan, S. Allam, B. A. Almatar and M. G. Radwan, PeerJ, 2024, 12, e17589 CrossRef.
- A. Golmohamadpour, B. Bahramian, M. Khoobi, M. Pourhajibagher, H. R. Barikani and A. Bahador, Photodiagn. Photodyn. Ther., 2018, 23, 331–338 CrossRef CAS.
- M. Chen, J. Zhang, J. Qi, R. Dong, H. Liu, D. Wu, H. Shao and X. Jiang, ACS Nano, 2022, 16, 7732–7744 CrossRef CAS PubMed.
- Y. Miao, W. Pan, K. Chen, H. Wei, F. Mi, M. Lu, Y. Chang and H. Sung, Adv. Funct. Mater., 2019, 29, 1904828 CrossRef CAS.
- N. O. Mishra, A. S. Quon, A. Nguyen, E. K. Papazyan, Y. Hao and Y. Liu, ACS Appl. Bio Mater., 2023, 6, 3052–3065 CrossRef CAS PubMed.
- F. N. Azad, M. Ghaedi, K. Dashtian, S. Hajati and V. Pezeshkpour, Ultrason. Sonochem., 2016, 31, 383–393 CrossRef CAS PubMed.
- A. Kumar, A. Sharma, Y. Chen, M. M. Jones, S. T. Vanyo, C. Li, M. B. Visser, S. D. Mahajan, R. K. Sharma and M. T. Swihart, Adv. Funct. Mater., 2021, 31, 2008054 CrossRef CAS PubMed.
- A. V. Desai, S. M. Vornholt, L. L. Major, R. Ettlinger, C. Jansen, D. N. Rainer, R. De Rome, V. So, P. S. Wheatley, A. K. Edward, C. G. Elliott, A. Pramanik, A. Karmakar, A. R. Armstrong, C. Janiak, T. K. Smith and R. E. Morris, ACS Appl. Mater. Interfaces, 2023, 15, 9058–9065 CrossRef CAS.
- K. Wang, R. Zhang, X. Zhao, Y. Ma, L. Ren, Y. Ren, G. Chen, D. Ye, J. Wu, X. Hu, Y. Guo, R. Xi, M. Meng, Q. Yao, P. Li, Q. Chen and T. D. James, J. Am. Chem. Soc., 2023, 145(15), 8408–8416 CrossRef CAS PubMed.
- S. A. Hashemi, S. Bahrani, S. M. Mousavi, N. Omidifar, M. Arjmand, K. B. Lankarani and S. Ramakrishna, Eur. Polym. J., 2022, 162, 110926 CrossRef CAS.
- F. Sanchis-Gomar, C. Perez-Quilis, R. Leischik and A. Lucia, Ann. Transl. Med., 2016, 4, 256 CrossRef PubMed.
- M. Y. Kalashgrani, F. F. Nejad and V. Rahmanian, Adv. Appl. NanoBio-Technol., 2022, 3(2), 38–42 CAS.
- Y. Zang, T. R. Roberts, A. I. Batchinsky and M. M. Reynolds, ACS Appl. Bio Mater., 2020, 3, 3535–3543 CrossRef CAS PubMed.
- C. Ding, W. Zhang, Z. Xu, H. Zhang and J. Zheng, J. Exp. Nanosci., 2020, 15, 87–96 CrossRef CAS.
- L.-L. Tan, N. Song, S. X.-A. Zhang, H. Li, B. Wang and Y.-W. Yang, J. Mater. Chem. B, 2016, 4, 135–140 RSC.
- F. Ejeian, A. Razmjou, M. H. Nasr-Esfahani, M. Mohammad, F. Karamali, M. Ebrahimi Warkiani, M. Asadnia and V. Chen, Int. J. Nanomed., 2020, 15, 10029–10043 CrossRef CAS.
- S. M. Mousavi, S. A. Hashemi, S. Bahrani, A. Sadrmousavi-Dizaj, O. Arjmand, N. Omidifar, C. W. Lai, W.-H. Chiang and A. Gholami, Bioinorg. Chem. Appl., 2022, 2022, 1–12 Search PubMed.
- S. M. Mousavi, S. A. Hashemi, Y. Ghahramani, R. Azhdari, K. Yousefi, A. Gholami, F. Fallahi Nezhad, N. Vijayakameswara Rao, N. Omidifar and W.-H. Chiang, Pharmaceuticals, 2022, 15, 1137 CrossRef CAS.
- C. Sarkar, A. R. Chowdhuri, S. Garai, J. Chakraborty and S. K. Sahu, Cellulose, 2019, 26, 7253–7269 CrossRef CAS.
- A. Karakeçili, B. Topuz, S. Korpayev and M. Erdek, Mater. Sci. Eng. C, 2019, 105, 110098 CrossRef.
- B. Tao, W. Zhao, C. Lin, Z. Yuan, Y. He, L. Lu, M. Chen, Y. Ding, Y. Yang, Z. Xia and K. Cai, Chem. Eng. J., 2020, 390, 124621 CrossRef CAS.
- J. A. Wixey, K. R. Sukumar, R. Pretorius, K. M. Lee, P. B. Colditz, S. T. Bjorkman and K. K. Chand, Front. Physiol., 2019, 10, 541 CrossRef.
- A. Pinna, R. Ricco’, R. Migheli, G. Rocchitta, P. A. Serra, P. Falcaro, L. Malfatti and P. Innocenzi, RSC Adv., 2018, 8, 25664–25672 RSC.
- M. Shyngys, J. Ren, X. Liang, J. Miao, A. Blocki and S. Beyer, Front. Bioeng. Biotechnol., 2021, 9, 603608 CrossRef PubMed.
- S. Tian and D. Tian, Supramol. Chem., 2020, 32, 365–372 CrossRef CAS.
- U. Shashikumar, S. Joshi, A. Srivastava, P.-C. Tsai, K. D. S. Shree, M. Suresh, B. Ravindran, C. M. Hussain, S. Chawla, L.-Y. Ke and V. K. Ponnusamy, Int. J. Biol. Macromol., 2023, 253, 127120 CrossRef CAS.
- M. D. J. Velásquez-Hernández, M. Linares-Moreau, E. Astria, F. Carraro, M. Z. Alyami, N. M. Khashab, C. J. Sumby, C. J. Doonan and P. Falcaro, Coord. Chem. Rev., 2021, 429, 213651 CrossRef.
- N. Singh, S. Qutub and N. M. Khashab, J. Mater. Chem. B, 2021, 9, 5925–5934 RSC.
- Y. Sun, L. Zheng, Y. Yang, X. Qian, T. Fu, X. Li, Z. Yang, H. Yan, C. Cui and W. Tan, Nano-Micro Lett., 2020, 12, 103 CrossRef CAS PubMed.
- I. Tibbetts and G. Kostakis, Molecules, 2020, 25, 1291 CrossRef CAS.
- N. Singh, S. Qutub and N. M. Khashab, J. Mater. Chem. B, 2021, 9, 5925–5934 RSC.
- J. Yao, Y. Liu, J. Wang, Q. Jiang, D. She, H. Guo, N. Sun, Z. Pang, C. Deng, W. Yang and S. Shen, Biomaterials, 2019, 195, 51–62 CrossRef CAS.
- Y. Lin, Y. Zhong, Y. Chen, L. Li, G. Chen, J. Zhang, P. Li, C. Zhou, Y. Sun, Y. Ma, Z. Xie and Q. Liao, Mol. Pharm., 2020, 17, 3328–3341 CrossRef CAS.
- S.-Z. Ren, D. Zhu, X.-H. Zhu, B. Wang, Y.-S. Yang, W.-X. Sun, X.-M. Wang, P.-C. Lv, Z.-C. Wang and H.-L. Zhu, ACS Appl. Mater. Interfaces, 2019, 11, 20678–20688 CrossRef CAS.
- C. Tamames-Tabar, D. Cunha, E. Imbuluzqueta, F. Ragon, C. Serre, M. J. Blanco-Prieto and P. Horcajada, J. Mater. Chem. B, 2014, 2, 262–271 RSC.
- X. Guo, L. Zhou, X. Liu, G. Tan, F. Yuan, A. Nezamzadeh-Ejhieh, N. Qi, J. Liu and Y. Peng, Colloids Surf., B, 2023, 229, 113455 CrossRef CAS.
- A. Wagner, Q. Liu, O. L. Rose, A. Eden, A. Vijay, Y. Rojanasakul and C. Z. Dinu, Int. J. Nanomed., 2019, 14, 7583–7591 CrossRef CAS PubMed.
- M. Ahmadi, S. M. Ayyoubzadeh, F. Ghorbani-Bidkorbeh, S. Shahhosseini, S. Dadashzadeh, E. Asadian, M. Mosayebnia and S. Siavashy, Heliyon, 2021, 7, e06914 CrossRef CAS PubMed.
- Y. Zhang, A. R. Khan, X. Yang, M. Fu, R. Wang, L. Chi and G. Zhai, J. Drug Delivery Sci. Technol., 2021, 61, 102266 CrossRef CAS.
- P. Wiśniewska, J. Haponiuk, M. R. Saeb, N. Rabiee and S. A. Bencherif, Chem. Eng. J., 2023, 471, 144400 CrossRef PubMed.
- N. Rabiee, Mater. Today Commun., 2023, 35, 106244 CrossRef CAS.
- S. Khan, M. Falahati, W. C. Cho, Y. Vahdani, R. Siddique, M. Sharifi, L. A. Jaragh-Alhadad, S. Haghighat, X. Zhang, T. L. M. Ten Hagen and Q. Bai, Adv. Colloid Interface Sci., 2023, 321, 103007 CrossRef CAS.
- N. Singh, S. Qutub and N. M. Khashab, J. Mater. Chem. B, 2021, 9, 5925–5934 RSC.
- Z. Zong, G. Tian, J. Wang, C. Fan, F. Yang and F. Guo, Pharmaceutics, 2022, 14, 2790 CrossRef CAS.
- A. Abdelkawi, A. Slim, Z. Zinoune and Y. Pathak, Coatings, 2023, 13, 1660 CrossRef CAS.
- S. Khan, J. K. Monteiro, A. Prasad, C. D. M. Filipe, Y. Li and T. F. Didar, Adv. Mater., 2024, 36, 2300875 CrossRef CAS PubMed.
- C. Carrillo-Carrión, Anal. Bioanal. Chem., 2020, 412, 37–54 CrossRef.
- X. Liu, T. Liang, R. Zhang, Q. Ding, S. Wu, C. Li, Y. Lin, Y. Ye, Z. Zhong and M. Zhou, ACS Appl. Mater. Interfaces, 2021, 13, 9643–9655 CrossRef CAS PubMed.
- B. Nirosha Yalamandala, W. Shen, S. Min, W. Chiang, S. Chang and S. Hu, Adv. NanoBiomed Res., 2021, 1, 2100014 CrossRef CAS.
- K. Song, Y.-T. Pan, J. Zhang, P. Song, J. He, D.-Y. Wang and R. Yang, Chem. Eng. J., 2023, 468, 143653 CrossRef CAS.
- S. F. Fatima, R. Sabouni, R. Garg and H. Gomaa, Colloids Surf., B, 2023, 225, 113266 CrossRef CAS PubMed.
- M. Bellusci, P. Guglielmi, A. Masi, F. Padella, G. Singh, N. Yaacoub, D. Peddis and D. Secci, Inorg. Chem., 2018, 57, 1806–1814 CrossRef CAS.
- S. Karimzadeh, S. Javanbakht, B. Baradaran, M.-A. Shahbazi, M. Hashemzaei, A. Mokhtarzadeh and H. A. Santos, Chem. Eng. J., 2021, 408, 127233 CrossRef CAS.
- P. Mi, Theranostics, 2020, 10, 4557–4588 CrossRef CAS PubMed.
- C. Zhao, R. Jing, S. Wang and X. Tang, J. Beijing Inst. Technol., 2022, 31, 225–237 Search PubMed.
- Y. Wang, J. Yan, N. Wen, H. Xiong, S. Cai, Q. He, Y. Hu, D. Peng, Z. Liu and Y. Liu, Biomaterials, 2020, 230, 119619 CrossRef CAS PubMed.
- X. Yang, Q. Tang, Y. Jiang, M. Zhang, M. Wang and L. Mao, J. Am. Chem. Soc., 2019, 141, 3782–3786 CrossRef CAS PubMed.
- Y. Liu, C. S. Gong, L. Lin, Z. Zhou, Y. Liu, Z. Yang, Z. Shen, G. Yu, Z. Wang, S. Wang, Y. Ma, W. Fan, L. He, G. Niu, Y. Dai and X. Chen, Theranostics, 2019, 9, 2791–2799 CrossRef CAS PubMed.
- F. Oroojalian, S. Karimzadeh, S. Javanbakht, M. Hejazi, B. Baradaran, T. J. Webster, A. Mokhtarzadeh, R. S. Varma, P. Kesharwani and A. Sahebkar, Mater. Today, 2022, 57, 192–224 CrossRef CAS.
- F. Li, Y. Qin, J. Lee, H. Liao, N. Wang, T. P. Davis, R. Qiao and D. Ling, J. Controlled Release, 2020, 322, 566–592 CrossRef CAS PubMed.
- X.-X. Chen, M.-J. Hou, W.-X. Wang, M. Tan, Z.-K. Tan, G.-J. Mao, B. Yang, Y. Li and C.-Y. Li, Nanoscale, 2022, 14, 3808–3817 RSC.
- W.-H. Chen, G.-F. Luo, M. Vázquez-González, R. Cazelles, Y. S. Sohn, R. Nechushtai, Y. Mandel and I. Willner, ACS Nano, 2018, 12, 7538–7545 CrossRef CAS.
- R. Chen, J. Zhang, J. Chelora, Y. Xiong, S. V. Kershaw, K. F. Li, P.-K. Lo, K. W. Cheah, A. L. Rogach, J. A. Zapien and C.-S. Lee, ACS Appl. Mater. Interfaces, 2017, 9, 5699–5708 CrossRef CAS.
- M. Wei, Y. Wan and X. Zhang, J. Compos. Sci., 2021, 5, 101 CrossRef CAS.
- L. Ruirui, P. Feifei, C. Jia, Y. Dandan and Z. Peng, Asian J. Pharm. Sci., 2020, 15(3), 311–325 CrossRef.
- C. Liu, X. Xu, J. Zhou, J. Yan, D. Wang and H. Zhang, BMC Mater, 2020, 2, 7 CrossRef.
- C. Zhang, Y. Li, H. Xu, J. Ma and H. Zheng, Chem.–Eur. J., 2018, 24, 327–331 CrossRef CAS PubMed.
- C. Li, K. Wang, J. Li and Q. Zhang, ACS Mater. Lett., 2020, 2, 779–797 CrossRef CAS.
- C. Yang, R. Dong, M. Wang, P. St. Petkov, Z. Zhang, M. Wang, P. Han, M. Ballabio, S. A. Bräuninger, Z. Liao, J. Zhang, F. Schwotzer, E. Zschech, H.-H. Klauss, E. Cánovas, S. Kaskel, M. Bonn, S. Zhou, T. Heine and X. Feng, Nat. Commun., 2019, 10, 3260 CrossRef PubMed.
- M. Ibrahim, R. Sabouni, G. A. Husseini, A. Karami, R. G. Bai and D. Mukhopadhyay, J. Biomed. Nanotechnol., 2020, 16, 1359–1369 CrossRef CAS PubMed.
- Z. Liu, L. Zhang and D. Sun, Chem. Commun., 2020, 56, 9416–9432 RSC.
- N. Yanai, T. Uemura, M. Inoue, R. Matsuda, T. Fukushima, M. Tsujimoto, S. Isoda and S. Kitagawa, J. Am. Chem. Soc., 2012, 134, 4501–4504 CrossRef CAS.
- R. Lyndon, K. Konstas, B. P. Ladewig, P. D. Southon, P. C. J. Kepert and M. R. Hill, Angew. Chem., Int. Ed., 2013, 52, 3695–3698 CrossRef CAS.
- Z. Luo, L. Jiang, S. Yang, Z. Li, W. M. W. Soh, L. Zheng, X. J. Loh and Y. Wu, Adv. Healthcare Mater., 2019, 8, 1900406 CrossRef.
- J. W. M. Osterrieth and D. Fairen-Jimenez, Biotechnol. J., 2021, 16, 2000005 CrossRef CAS PubMed.
- M. Cedrún-Morales, M. Ceballos, E. Polo, P. Del Pino and B. Pelaz, Chem. Commun., 2023, 59, 2869–2887 RSC.
- H. Musarurwa and N. T. Tavengwa, Mater. Today Commun., 2022, 33, 104823 CrossRef CAS.
- Q. Guan, Y. Fang, X. Wu, R. Ou, X. Zhang, H. Xie, M. Tang and G. Zeng, Mater. Today, 2023, 64, 138–164 CrossRef.
- D. Gao, X. Guo, X. Zhang, S. Chen, Y. Wang, T. Chen, G. Huang, Y. Gao, Z. Tian and Z. Yang, Mater. Today Bio, 2020, 5, 100035 CrossRef CAS PubMed.
- R. S. Chouhan, M. Horvat, J. Ahmed, N. Alhokbany, S. M. Alshehri and S. Gandhi, Cancers, 2021, 13, 2213 CrossRef CAS.
- J. Yang and Y. Yang, Small, 2020, 16, 1906846 CrossRef CAS PubMed.
- Y. Zhu, N. Xin, Z. Qiao, S. Chen, L. Zeng, Y. Zhang, D. Wei, J. Sun and H. Fan, Advanced Healthcare Materials, 2020, 9(14), 2000205 CrossRef CAS.
- S. Li, L. Tan and X. Meng, Adv. Funct. Mater., 2020, 30, 1908924 CrossRef CAS.
- M. Jia, X. Yang, Y. Chen, M. He, W. Zhou, J. Lin, L. An and S. Yang, J. Mater. Chem. B, 2021, 9, 8631–8638 RSC.
- N. T. Thao Nguyen, T. T. Thanh Nguyen, S. Ge, R. Keey Liew, D. T. Cam Nguyen and T. Van Tran, Nanoscale Adv., 2024, 6, 1800–1821 RSC.
- J. Bao, X. Zu, X. Wang, J. Li, D. Fan, Y. Shi, Q. Xia and J. Cheng, Int. J. Nanomed., 2020, 15, 7687–7702 CrossRef CAS.
- Y. Ma, J. Mao, H. Qin, P. Liang, W. Huang, C. Liu and J. Gao, Front. Bioeng. Biotechnol., 2022, 10, 927461 CrossRef.
- X. Zhang, Y. Lu, D. Jia, W. Qiu, X. Ma, X. Zhang, Z. Xu and F. Wen, J. Nanobiotechnol., 2021, 19, 455 CrossRef CAS.
- C. Yuan, Y. Li, Z. Xu, C. Wang, J. Zhang, Y. Jin, Z. Chen, H. Sun, F. Wu, Q. Zhang, Y. Tang and S. Wang, ACS Appl. Nano Mater., 2022, 5, 15318–15327 CrossRef CAS.
- D. Ma, G. Wang, J. Lu, X. Zeng, Y. Cheng, Z. Zhang, N. Lin and Q. Chen, Eur. J. Med. Chem., 2023, 261, 115884 CrossRef CAS PubMed.
- Y. Gao, K. Wang, J. Zhang, X. Duan, Q. Sun and K. Men, MedComm, 2023, 4, e187 CrossRef CAS PubMed.
- Y. Liu, T. Jiang and Z. Liu, Nanotheranostics, 2022, 6, 143–160 CrossRef PubMed.
- A. Mavridi-Printezi, M. Guernelli, A. Menichetti and M. Montalti, Nanomaterials, 2020, 10, 2276 CrossRef CAS.
- D. Kim, K. W. Park, J. T. Park and I. Choi, ACS Appl. Mater. Interfaces, 2023, 15, 22903–22914 CrossRef CAS.
- Z. Mo, X. Pan, X. Pan, L. Ye, H. Hu, Q. Xu, X. Hu, Z. Xu, J. Xiong, G. Liao and S. Yang, Journal of Materials Chemistry B, 2022, 10(42), 8760 RSC.
- H. Zhao, S. Sene, A. M. Mielcarek, S. Miraux, N. Menguy, D. Ihiawakrim, O. Ersen, C. Péchoux, N. Guillou, J. Scola, J.-M. Grenèche, F. Nouar, S. Mura, F. Carn, F. Gazeau, E. Dumas, C. Serre and N. Steunou, J. Mater. Chem. B, 2023, 11, 3195–3211 RSC.
- J. Yang, D. Dai, X. Zhang, L. Teng, L. Ma and Y.-W. Yang, Theranostics, 2023, 13, 295–323 CrossRef CAS PubMed.
- R. Abazari, A. R. Mahjoub, F. Ataei, A. Morsali, C. L. Carpenter-Warren, K. Mehdizadeh and A. M. Z. Slawin, Inorg. Chem., 2018, 57, 13364–13379 CrossRef CAS.
- S. R. Datir, M. Das, R. P. Singh and S. Jain, Bioconjugate Chem., 2012, 23, 2201–2213 CrossRef CAS PubMed.
- S. Wu, X. Huang and X. Du, J. Mater. Chem. B, 2015, 3, 1426–1432 RSC.
- P. Horcajada, T. Chalati, C. Serre, B. Gillet, C. Sebrie, T. Baati, J. F. Eubank, D. Heurtaux, P. Clayette, C. Kreuz, J.-S. Chang, Y. K. Hwang, V. Marsaud, P.-N. Bories, L. Cynober, S. Gil, G. Férey, P. Couvreur and R. Gref, Nat. Mater., 2010, 9, 172–178 CrossRef CAS.
- Z. Shi, X. Chen, L. Zhang, S. Ding, X. Wang, Q. Lei and W. Fang, Biomater. Sci., 2018, 6, 2582–2590 RSC.
- K. Sun, L. Li, X. Yu, L. Liu, Q. Meng, F. Wang and R. Zhang, J. Colloid Interface Sci., 2017, 486, 128–135 CrossRef CAS.
- H. Yu, X. Qiu, P. Neelakanda, L. Deng, N. M. Khashab, S. P. Nunes and K.-V. Peinemann, Sci. Rep., 2015, 5, 15275 CrossRef CAS.
- D. Hu, H. Xu, B. Xiao, D. Li, Z. Zhou, X. Liu, J. Tang and Y. Shen, ACS Appl. Mater. Interfaces, 2018, 10, 34974–34982 CrossRef CAS PubMed.
- Z. Li, R. Guo, Z. Gu, X. Wang, Y. Wang, H. Xu, C. Wang and X. Liu, Gene, 2019, 699, 1–7 CrossRef CAS.
- Y. Li, Y. Zheng, X. Lai, Y. Chu and Y. Chen, RSC Adv., 2018, 8, 23623–23628 RSC.
- H. Zheng, Y. Zhang, L. Liu, W. Wan, P. Guo, A. M. Nyström and X. Zou, J. Am. Chem. Soc., 2016, 138, 962–968 CrossRef CAS PubMed.
- Z. Liang, Z. Yang, H. Yuan, C. Wang, J. Qi, K. Liu, R. Cao and H. Zheng, Dalton Trans., 2018, 47, 10223–10228 RSC.
- H. Li, N. Lv, X. Li, B. Liu, J. Feng, X. Ren, T. Guo, D. Chen, J. Fraser Stoddart, R. Gref and J. Zhang, Nanoscale, 2017, 9, 7454–7463 RSC.
- G. Gumilar, Y. V. Kaneti, J. Henzie, S. Chatterjee, J. Na, B. Yuliarto, N. Nugraha, A. Patah, A. Bhaumik and Y. Yamauchi, Chem. Sci., 2020, 11, 3644–3655 RSC.
- J. Hu, Y. Chen, H. Zhang and Z. Chen, J. Solid State Chem., 2021, 294, 121853 CrossRef CAS.
- H. Kim and C. S. Hong, CrystEngComm, 2021, 23, 1377–1387 RSC.
- Z. Zong, G. Tian, J. Wang, C. Fan, F. Yang and F. Guo, Pharmaceutics, 2022, 14, 2790 CrossRef CAS PubMed.
- R. Liang, N. Liu and F. Li, ChemMedChem, 2022, 17, e202200480 CrossRef CAS PubMed.
- S. Lawson, K. Newport, K. Schueddig, A. A. Rownaghi and F. Rezaei, Mater. Sci. Eng. C, 2020, 117, 111336 CrossRef CAS PubMed.
- M.-X. Wu and Y.-W. Yang, Adv. Mater., 2017, 29, 1606134 CrossRef.
- K. Chattopadhyay, M. Mandal and D. K. Maiti, Mater. Adv., 2024, 5, 51–67 RSC.
- D. B. Trushina, A. Yu. Sapach, O. A. Burachevskaia, P. V. Medvedev, D. N. Khmelenin, T. N. Borodina, M. A. Soldatov and V. V. Butova, Pharmaceutics, 2022, 14, 1325 CrossRef CAS PubMed.
- B. L. Bonnett, E. D. Smith, M. De La Garza, M. Cai, J. V. Haag, J. M. Serrano, H. D. Cornell, B. Gibbons, S. M. Martin and A. J. Morris, ACS Appl. Mater. Interfaces, 2020, 12, 15765–15773 CrossRef CAS.
- S. Lin, X. Liu, L. Tan, Z. Cui, X. Yang, K. W. K. Yeung, H. Pan and S. Wu, ACS Appl. Mater. Interfaces, 2017, 9, 19248–19257 CrossRef CAS PubMed.
- G. Kumar, A. Kant, M. Kumar and D. T. Masram, Inorg. Chim. Acta, 2019, 496, 119036 CrossRef CAS.
- A. K. Singh, R. Malviya and S. Verma, Curr. Drug Res. Rev., 2023, 15, 101–104 CrossRef.
- G. Wyszogrodzka, P. Dorożyński, B. Gil, W. J. Roth, M. Strzempek, B. Marszałek, W. P. Węglarz, E. Menaszek, W. Strzempek and P. Kulinowski, Pharm. Res., 2018, 35, 144 CrossRef PubMed.
|
This journal is © The Royal Society of Chemistry 2024 |
Click here to see how this site uses Cookies. View our privacy policy here.