DOI:
10.1039/D4RA04695A
(Paper)
RSC Adv., 2024,
14, 23037-23047
Effects of mixed enzymolysis alone or combined with acetylation or carboxymethylation on the role of jujube kernel fibre as a biosorbent for wastewater treatment
Received
28th June 2024
, Accepted 4th July 2024
First published on 22nd July 2024
Abstract
Jujube kernel fibre (JKF) could serve as a renewable, abundant, low-cost, and environmentally friendly adsorbent for wastewater if its adsorption capacities are improved. However, data on the modification of JKF, especially on the combination of biological and chemical modifications, are scarce. Therefore, for the first time, we studied the effect of mixed enzymolysis alone or combined with acetylation or carboxymethylation on the structure and adsorption capacities of JKF. After these modifications, the microstructure of JKF became more porous, and its soluble fibre and extractable polyphenol contents, surface area and adsorption capacities for nitrite, copper, and lead ions were all significantly improved (P < 0.05). Meanwhile, mixed enzymatic hydrolysis and acetylation treated JKF showed the highest surface hydrophobicity (43.57) and oil-adsorption ability (4.47 g g−1), while mixed enzymatic hydrolysis and carboxymethylation treated JKF exhibited the highest water adsorption ability (10.66 g g−1), water expansion ability (8.50 mL g−1), and lead and copper ion chelating abilities. Additionally, mixed enzymatic hydrolyzed JKF had the highest nitrite-ion-adsorption ability (10.57 μmol g−1). It can be concluded that mixed enzymolysis combined with carboxymethylation is an optimal way to increase the hydration properties and heavy-metal-adsorption capacity of JKF, while mixed enzymolysis combined with acetylation is an effective approach to enhance the oil-adsorption capacity of JKF.
1 Introduction
Water pollution is one of the biggest problems threatening human survival and security. Pathogenic microorganisms, parasites, heavy metals, grease, detergent and phytonutrients (such as nitrogen, phosphorus and sulfur) in contaminated water can deteriorate water quality, reduce or eliminate crop yields, contaminate food and cause and spread various diseases.1 Industrial wastewater, gas and residues, pesticide and veterinary drug residues, and non-compliant packaging materials are the main sources of heavy metal pollution in water.2 Most heavy metals, such as lead(II) (Pb2+), cadmium (Cd+) and mercury (Hg+) ions, are hazardous because they accumulate in living organisms and cause fatal diseases,1 while excessive essential heavy metals in water, such as copper, iron, and manganese ions, can cause serious damage to the liver, kidney, and other organs of humans.3 Moreover, excessive phytonutrients, such as nitrite, phosphorus, and sulfur, in water can cause eutrophication, water-quality degradation, bloom, and even the death of fish and other organisms.4 Additionally, oil and sugar in domestic and industrial sewage can breed a large number of pathogenic bacteria and microorganisms, thus depleting the oxygen in water and gradually resulting in the death of animals and plants in the water.1,5 Therefore, technologies, such as adsorption, reverse osmosis, and ion exchange, that can remove heavy metals, phytonutrients, and other toxic substances from wastewater have attracted increasing attention. Among these, the use of natural biopolymers, especially fibre, starch, and chitosan, as adsorbents to treat wastewater is popular for their high availability, low cost, and renewability.4,6
Compared to active carbon and starch, adsorbents derived from plant fibres have remarkable advantages, including availability, low cost, reasonable strength and stiffness, and low density.5 In recent decades, the adsorption properties of heavy metals, phytonutrients, oil and other toxic substances in wastewater on various agro-based waste fibres, such as rice bran, coconut shell, millet bran, and sugarcane bagasse, have been studied.6–8 Although insoluble fibre also plays a role in the wastewater treatment ability of fibre adsorbents, a large number of studies have shown that the wastewater treatment ability of fibre is mainly dependent on surface microstructure, soluble fibre content, hydrophobicity and hydrophilicity.1,5 However, many natural fibre adsorbents show relatively poor adsorption capacities for toxic and harmful substances in wastewater due to their low hydration properties, small specific surface area, and few functional groups.4 Thus, various physical, biological, and chemical modifications have been explored to improve the adsorption capacity of fibres.6,9 Among these methods, enzyme hydrolysis is a biological modification for increasing the hydration and adsorption properties of fibres, with the advantages of environmental friendliness, low-energy consumption, selectivity, and mild operation.10–12 Cellulase and lignin enzyme hydrolysis can cause a rupture of the glycoside bonds of fibres, exposing more hydrophilic groups and increasing the contact area of fibres with adsorbed substances.7,13 Moreover, hydroxypropylation, acetylation, and carboxymethylation can induce the introduction of hydroxypropyl, ester and carboxymethyl groups into fibres, respectively. These introduced groups can improve the polarity of fibres, thereby improving their specific area, hydration properties, and adsorption capacities.14–16 However, the composite influence of these biological and chemical modifications on the adsorption capacity of fibres is small.
Red jujube (Ziziphus jujuba Mill.) is one of the main fruits in the north of China, with a cultivation history of around 4000 years.17 Jujube kernel, a potential dietary fibre resource, is rich in fibre, of which cellulose and lignin account for around 49 and 24 g 100 g−1, respectively.18 Every year, approximately 200
000 tons of jujube kernels are produced in China, but only a small part is used as medicine for nourishing the liver, and calming the heart and mind, while the majority are discarded directly, causing serious environmental pollution problems.19,20 Although jujube kernel fibre (JKF) has a relatively high water sorption ability, its soluble fibre content (6.32 g 100 g−1) is low and its sorption abilities for oil and heavy metal ions are poor, which is not conducive for its applications as a wastewater adsorbent.21 Moreover, alkaline treatment has been shown to be effective at reducing the cellulose content of date-pit fibres,22 albeit there is scant data referring to the modifications of JKF, especially the synergistic effects of physical, chemical, and biological modifications for wastewater treatment. Thus, to improve the wastewater treatment capacity of JKF, mixed enzymatic hydrolysis (cellulase, hemicellulase, and laccase hydrolysis), mixed enzymatic hydrolysis assisted with acetylation or carboxymethylation were used to modify JKF. Changes in the structure, hydration properties, and the ability of purifying wastewater by these composite modification methods were investigated with the purpose of expanding the usage of JKF as a low-cost, biodegradable and renewable wastewater adsorbent.
2 Experimental
2.1. Materials
Jujube kernels were purchased from Zaohua Red Jujube Processing Factory, Taigu, China. Laccase (75 U g−1, where one unit corresponds to the amount of enzymes that can convert 1 μmol catechol per hour at pH 5.0 and 25 °C), hemicellulase (from Aspergillus niger, 2.0 × 105 U g−1, where one unit can liberate 1.0 μmol of D-galactose from hemicellulose per hour at pH 5.5 at 45 °C), cellulase (from Trichoderma Vride G, 3.0 × 105 U g−1, where one unit corresponds to the amount of enzymes that can convert 1 μmol substrate to produce glucose per hour at pH 4.8 and 50 °C), amyloglucosidase (Aspergillus niger, 1.0 × 105 U g−1, where one unit corresponds to the amount of enzymes that can convert 1 μmol soluble starch per minute at pH 4.6 and 40 °C), and α-amylase (Bacillus licheniformis, 1.0 × 105 U g−1, where one unit corresponds to the amount of enzymes that can convert 1 μmol starch to produce glucose per hour at pH 6.9 and 25 °C) were all purchased from Peisun Enzyme Company (Nanning, China). Monochloroacetic acid, isopropenyl acrylate, and other analytical reagents were obtained from Maoyuan Chemical Reagent Company (Tianjin, China).
2.2. JKF preparation
Jujube kernel was dried at 45 °C for 12 h, and then ground and sieved using a XL-30C grinder (Xulang Machinery, Guangzhou, China) and a sieve (aperture: 150 μm) (Yushang Sieve Instrument, Shaoxing, China) in sequence. Next, 80 g of the ground powder, phosphate buffer (100 μmol L−1, 1 L), and α-amylase (2.4 g) were added and mixed in a triangular glass bottle.15 The mixture in the bottle was shaken with an HEZ-004C shaker (Shangxu Instrument Factory, Shangyu, China) at 225 rpm and 90 °C, and the pH value of the mixture was maintained at 5.0 through adjusting the pH with NaOH (100 mmol L−1) or HCl (100 mmol L−1) every 30 min. Then, 90 min later, the mixture was adjusted to pH 7.0 and papain (1.2 g) was added. The mixture in the bottle was continuously shaken at 225 rpm (50 °C, 120 min). Afterward the pH value of the mixture was lowered to 4.0, and then glucoamylase (1.2 g) was added. The bottle was shaken in the HEZ-004C shaker at 225 rpm and 60 °C for 120 min, and then heated in boiling water for 10 min. Finally, the reaction mixture was filtered with a filter paper (Whatman 1004-047), and the residue on the paper was dried in a GHZ-43B blast drying oven (45 °C, 6 h). The dried residue was ground and passed through a sieve (aperture: 150 μm), and JKF was obtained.
2.3. Mixed enzymatic hydrolysis of JKF
In a glass bottle, JKF (20 g), 400 mL of buffer (pH 5.0, 0.1 mol L−1), 0.15 g of cellulase, and 0.05 g of hemicellulase were mixed. The suspension in the bottle was shaken in the EZH-004C shaker (48 °C and 225 rpm) for 2 h. NaOH (0.1 mol L−1) was used for adjusting the pH value of the suspension to 6.5 ± 0.1 and the reaction temperature was adjusted to 35.0 °C, and then laccase (25 U g−1 JKF) was added. The reaction suspension was shaken at 225 rpm for 2 h, and then heated in boiling water for 10 min. Afterward, the suspension was filtered with filter paper (Whatman1004-047), and the residue on the paper was dried in the GHZ-43B blast drying oven (52 °C, 6 h). The dried residue was ground and passed through a sieve (aperture: 150 μm), and the mixed enzymatic hydrolyzed jujube kernel fibre (JKF-E) was obtained.
2.4. Acetylation of JKF-E
Here, 20 g of JKF-E was placed in a triangular flask, and dimethyl sulfoxide (270 mL) was slowly added to the inside of the flask.15 The suspension in the bottle was shaken at 70 °C in the HEZ-004C shaker with a stirring speed of 45 rpm. Then, 3 h later, the temperature was lowered to 40 °C, and then sodium carbonate (0.3 g) and 7.4 mol L−1 isopropenyl acetate (16 mL) were added. The mixture was continuously shaken at 45 rpm and 40 °C in the oscillator for 70 h, and then filtered with filter paper (Whatman1004-047). Isopropanol (60 mL) was used to purify the residue on the filter paper in triplicate. Then the residue was dried at 48 °C for 7 h and the jujube kernel fibres modified by mixed enzymatic hydrolysis and acetylation (JKF-EA) was obtained.
2.5. Carboxymethylation of JKF-E
The carboxymethylation of OPKEF-GE was performed by slight modification of the procedure of Zhang et al.23 First, 20 g of OPKEF-GE was alkalized using 70 mmol L−1 of NaOH (dissolved in 85% ethanol, v/v) at 35 °C for 70 min. Afterward, chloroacetic acid (3.5 mmol L−1, dissolved in 85% ethanol solution) was added. The reaction mixture was shaken in the EZH-004C shaker at a temperature of 35 °C and shaking rate of 115 rpm. Then, 75 min later, the reaction mixture was slowly mixed with 45 mL of NaOH solution (3.4 mol L−1), and then continuously shaken at 53 °C and 115 rpm. Then, 4 h later, the mixture was slowly neutralized with acetic acid (8.75 mol L−1), and then centrifuged at 4100×g for 0.5 h. The obtained precipitate was purified through washing with anhydrous ethanol (15 mL each time), and dried at 50 °C for 7 h. JKF treated by mixed enzymatic hydrolysis and carboxymethylation (JKF-EC) was finally obtained. The substitution degree was defined as the increment in carboxymethyl group content of JKF-E after carboxymethylation.24
2.6. Determination of the chemical constituent
The methods AOAC.955.04, AOAC.92.05, AOAC.924.05, and AOAC.920.39 were used to determine the contents of protein, fat, ash, and moisture of the jujube kernel fibres, respectively.25 The cellulose, lignin, and hemicellulose contents were measured according to the method of Zhu et al.9 Moreover, the AOAC.991.43 method was employed to determine the insoluble and total fibre contents, and the soluble fibre content is the difference between the total fibre content and the insoluble fibre content.25 Moreover, phenolic content determination was conducted with the Folin–Ciocalteu method.26
2.7. Determination of the particle size
JKFs were dispersed in distilled water (1
:
25 g mL−1) and then analysed with a WNINER 336B nanometre size analysis system (Xinna Particle Instrument Factory, Chengdu, China).15 The particle size was represented by the Sauter mean diameter (D3,2, μm) and the refractive index was 1.33. Moreover, the specific surface area (m2 kg−1) of the samples was read.
2.8. Changes in the structure of JKF after modification
2.8.1 Changes in the microstructure. The effects of different modification methods on the microstructure of JKF were determined using a scanning electron microscopy system (SEM, SJM-JEOL-7200E, Electronics Co., Ltd, Showima city, Japan). The dried samples were fixed on the sample table using a conductive adhesive. After spraying 10 nm gold, the samples on the table were photographed at a magnification of 5000.
2.8.2 Fourier-transform infrared spectroscopy (FT-IR). The infrared spectra of the JKFs were recorded with an HS-FT-725 Fourier-transform infrared (FT-IR) spectrometer (Precision Scientific Instrument Company, Shanghai, China). Dried KBr (0.2 g) was blended thoroughly with 4 mg of JKFs. Next, the mixed powder was pressed into a transparent sheet, and then analysed using the spectrometer in the scanning range of 4000–400 cm−1 at a resolution of 4 cm−1.12
2.9. Water absorption and expansion capacities
In a graduated centrifugal tube, dried JKFs were dispersed in distilled water with a mass to volume ratio of 1
:
15. The dispersion was placed at 25 °C for 230 min, and then centrifuged at 1500 g for 25 min.27 The upper water was removed, and the wet JFKs were weighed. The water absorption capacity (WAC) was calculated as follows: |
WAC (g g−1) = (Ww − Wd)/Wd
| (1) |
where Ww and Wd are representative of the wet and dry weights of the JKFs, respectively.
Moreover, 3 g of dry JKFs was placed in a glass cylinder with a plug and the volume was read (Vd, mL). Next, 54 mL distilled water was added and gently mixed for 3 min, and then the cylinder was placed at 25 °C for 20 h. Afterward the volume of the wet JKFs was read (Vw, mL), and the water expansion ability (WEA) was calculated as follows:
|
WEA (mL g−1) = (Vw − Vd)/Wd
| (2) |
where
Wd is the dry weight of the JKFs.
2.10. Surface hydrophobicity
As per the method of He et al.,28 JKF and the modified JKFs (JKF-E, JKF-EA, and JKF-EC) were dispersed in 0.1 mol L−1 of phosphate buffer (pH 7.4) for formulating solutions of different concentrations (20–500 μg mL−1). Next, 2.5 mL of the JKFs solution was reacted with 25 μL of 1-anilino-8-naphthalenesulfonate (10 mmol mL−1) at 25 °C for 2 min. Then the fluorescence intensity of the reaction solution was investigated using a DOLOBF93 spectrophotometer (Lengguang Technology and Science Co. Ltd, Shanghai, China) with an emission wavelength of 470 nm and an excitation wavelength of 390 nm. The regression curve between the fluorescent intensity of the JKFs' solution and their concentration was drawn, and the initial slope of the curve was defined as the surface hydrophobicity.
2.11. Adsorption properties
2.11.1 Oil-adsorption ability. The oil-adsorption ability (OAA) was determined referring to the a modified procedure from Asadpour et al.29 First, crude oil (25 mL) was added into 250 mL sodium chloride (3.5 g 100 mL−1, artificial seawater, 267.5 g), and then 1 g dry JKFs was added, thoroughly mixed, and then stirred in the HEZ-004C shaker at 165 rpm and at 25 °C for 10, 20, 30, 40, and 50 min, respectively. After filtration with a nylon mesh filter, the residual wet JKFs were collected and weighted (W2), and then used for determination of the water amount (W3) using the method AOAC.920.39. The OAA of the JKFs in artificial seawater was defined as follows: |
OAA (g g−1) = (W2 − W1 − W3)/W1
| (3) |
where W1 represents the weight of the dry JKFs.
2.11.2 Adsorbing capacity of nitrite ions on the JKFs. Referring to the procedures from Zheng et al.,15 0.2 mg 100 mL−1 of NaNO2 (25 mL, 26.75 g wastewater) was added into a glass beaker and pre-incubated at 25 °C for 5 min. Next, JKFs (0.5 g) was added and mixed thoroughly using the DTM-2500 vortex oscillator for 5 s. Afterward the beaker was placed in the HEZ-004C shaker (215 rpm and 35 °C). Then, 2 h later, the dispersion was filtered. The original nitrite content in the NaNO2 solution and the final nitrite content in the filtrate solution were both measured using the N-1-naphthylethylenediamine dihydrochloride method.30 The adsorbing capacity of nitrite ions (ACNI) on the JKFs was defined as the reduced nitrite content per weight of the JKFs (μmol g−1).
2.11.3 Adsorption capacity of lead ions(II) on the JKFs. The adsorption capacity of lead ions(II) (ACLI) on the JKFs was investigated according to a modified procedure from Rani et al.14 Briefly, 70 mg L−1 of lead nitrate (220 mL, 235.4 g wastewater) was added into a glass beaker and pre-incubated at 25 °C for 5 min. Next, 0.5 g of JKFs was added and mixed thoroughly using the DTM-2500 vortex oscillator for 5 s. Afterward the dispersions in the beaker were placed in the HEZ-004C shaker (215 rpm and 35 °C) for 5, 20, 35, 50, 65, 80, 95, and 110 min, respectively, and then were all filtered by nylon cloth with apertures of 8.3 mm. The flame atomic absorption spectrometry method was used to measure the original lead ion content in the cupric sulfate (Co) and the final lead ion content in the filtrate solution (Cf).5 The ACLI was defined according to the following equation: |
ACLI (mg g−1) = (C0 − CS) × 220/W
| (4) |
where 220 is the volume of lead nitrate (mL), and W represents the dry JKFs' weight.
2.11.4 Adsorption capacity of copper ions(II) on the JKFs. The isothermal adsorption kinetics of copper ions on the JKFs was investigated according to a modified procedure from Zheng et al.15 Briefly, 325 μg mL−1 of cupric sulfate (125 mL, 133.75 g wastewater) was added into a glass beaker and pre-incubated at 25 °C for 5 min. Next, 0.5 g of JKFs was added and mixed thoroughly using the DTM-2500 vortex oscillator for 5 s. Afterward the dispersions in the beaker were placed in the HEZ-004C shaker (215 rpm and 35 °C) for 10, 20, 40, 60, 80, and 100 min, respectively, and then were all filtered using nylon cloth with aperture of 8.3 s mm. The KI-soluble starch titration method was used to measure the original copper content in the cupric sulfate (Co) and the final copper content in the filtrate solution (Cf).3 The adsorption capacity of copper ions(II) (ACCI) on the JKFs was calculated using the following equation: |
ACCI (mg g−1) = (Co − Cf) × 125/W
| (5) |
where 125 is the volume of cupric sulfate (mL), and W represents the dry JKFs' weight.
2.12. Statistics analysis
The mean and standard deviation for all the data were obtained by duplicating all the experiments at least three times. Statistical differences were analysed by analysis of the variance (ANOVA) and Duncan test with the SPSS Mas program (SPSS Inc., Chicago, America). The threshold for significant difference (p < 0.05) was 95%.
3 Results and discussion
3.1. Effects of different modifications on the chemical components of the JKFs
The carboxymethylated degree of JKF-EC was 4.73% ± 0.22%, suggesting that a part of the hydrogen bonds in JKF had been replaced by carboxymethyl groups. Compared with hydrogen bonds, carboxymethyl groups have higher hydrophilicity, which could probably improve the water solubility of JKF. The acetylated degree of JKF-EA was 1.87 ± 0.13%, indicating that a part of the hydrogen bonds in JKF had been replaced by acyl ester bonds, which was conducive to the hydrophobicity of DFs.31 Moreover, the composite modification methods used in this study (mixed enzymatic hydrolysis, enzymatic hydrolysis assisted with acetylation or carboxymethylation) had no obvious impact on the fat, protein, or moisture contents (p > 0.05), because the difference between JKF, JKF-E, JKF-EA, and JKF-EC was not significant (p > 0.05, Table 1). By contrast, these modification methods all showed remarkably enhanced soluble fibre content of JKF (p < 0.05). Cellulase and laccase can cause a degradation of the glycosidic bonds, which can release more hydrophilic groups and increase the soluble fibre content of JKE.27
Table 1 Proximate compositions of jujube kernel fibre (JKF), JKF modified via mixed enzymes (cellulase, hemicellulase and laccase) hydrolysis (JKF-E), JKF treated with mixed enzymatic hydrolysis combined with carboxymethylation (JKF-EC), and JKF modified via mixed enzymatic hydrolysis combined with acetylation (JKF-EA)a
Constituent |
Jujube kernel |
JKF |
JKF-E |
JKF-EC |
JKF-EA |
Different small letters (a–e) in the same line indicate significant difference (p < 0.05). |
Moisture (g 100 g−1) |
5.25 ± 0.47a |
6.38 ± 0.11a |
5.37 ± 0.09a |
4.29 ± 0.20a |
5.12 ± 0.15a |
Fat (g 100 g−1) |
2.74 ± 0.25a |
1.93 ± 0.27b |
1.24 ± 0.05b |
0.97 ± 0.05b |
1.01 ± 0.06b |
Ash (g 100 g−1) |
0.81 ± 0.11b |
0.95 ± 0.04b |
1.51 ± 0.06a |
1.28 ± 0.09a |
1.41 ± 0.15a |
Protein (g 100 g−1) |
0.87 ± 0.09a |
0.38 ± 0.06a |
0.45 ± 0.03a |
0.39 ± 0.03a |
0.62 ± 0.06a |
Total fiber (g 100 g−1) |
87.36 ± 2.74b |
89.76 ± 2.56a |
91.36 ± 2.69a |
92.19 ± 4.52a |
90.64 ± 3.73a |
Insoluble fiber (g 100 g−1) |
81.87 ± 1.65a |
83.44 ± 3.43a |
77.72 ± 3.48b |
73.87 ± 1.19a |
76.64 ± 2.57b |
Soluble fiber (g 100 g−1) |
5.49 ± 0.24c |
6.32 ± 0.33c |
13.64 ± 1.42b |
18.32 ± 0.82a |
14.00 ± 1.22b |
Extractable polyphenols (mg g−1) |
3.46 ± 0.22d |
5.82 ± 0.37c |
12.65 ± 0.16a |
9.56 ± 0.37b |
10.07 ± 0.45b |
Cellulose (g 100 g−1) |
40.83 ± 1.64a |
41.82 ± 1.43a |
34.27 ± 1.25b |
35.45 ± 0.08b |
31.91 ± 1.02b |
Hemicellulose (g 100 g−1) |
24.25 ± 0.39a |
24.67 ± 0.78a |
17.58 ± 0.50b |
17.49 ± 0.32b |
18.05 ± 0.67b |
Lignin (g 100 g−1) |
24.37 ± 3.14a |
26.17 ± 3.27a |
14.34 ± 0.78b |
9.43 ± 0.37c |
8.39 ± 0.47c |
Moreover, the soluble fibre content of JKF-EC (18.32 ± 0.82 g 100 g−1) was much higher than that of JKF-E (p < 0.05), indicating that carboxymethylation significantly enhanced the effects of the mixed enzymatic hydrolysis to improve the polarity of JKF. The introduced carboxymethyl group had a relatively high polarity.16 In comparison, the soluble content of JKE-SGEA was not different from that of JKE-SGE (p > 0.05). Previous studies showed that acyl ester bonds could increase the hydrophobicity of fibres,15,16,32 therefore acetylation reduced the effect of mixed enzymatic hydrolysis on the polarity of JKF.
In addition, the cellulose, hemicellulose, and lignin contents of JKF were all decreased after the three modifications (p < 0.05), corresponding to their lower insoluble fibre content (Table 1). Cellulase, hemicellulase, and laccase can cause a degradation of cellulose, hemicellulose, and lignin,33 resulting in a decreased insoluble fibre content and increased soluble fibre content. Furthermore, the soluble fibre contents of JKF, JKF-EC, and JKF-EA (13.64–18.32 g 100 g−1) were much higher than those of rice bran fibre (3.03 g 100 g−1),7 coconut mesocarp fibre (4.01 g 100 g−1),27 soybean hulls fibre (1.76 g 100 g−1),9 and sugarcane bagasse fibre (3.12 g 100 g−1),6 highlighting their relatively high hydration properties.
Apart from that, the extractable polyphenol content of JKF was 3.46 ± 0.22 mg g−1, which was in accordance with the result of Ji et al.21 The extractable polyphenol contents of JKF-E, JKF-EA, and JKF-EC were all higher than that of JKF (p < 0.05), probably due to laccase hydrolysis. Laccase causes a degradation of lignin (polyphenol polymers) and thus significantly increases the extractable phenols.12
3.2. Effects of different modifications on particle size of JKF
The results in Table 2 show that mixed enzymatic hydrolysis, and mixed enzymatic hydrolysis combined with acetylation or carboxymethylation increased the surface area of the JKF and reduced its particle size (p < 0.05). Mixed enzymatic hydrolysis can cause the breakdown of the fibre cell well and degradation of the polysaccharide chains,11 resulting in a reduction of JKF's particle size. The surface area of JKF-EA (146.37 ± 3.72 m2 kg−1) was higher than that of the other JKFs, and its particle size (63.77 ± 1.53 μm) was lower than that of the other JKFs (p < 0.05). An increase in surface area can improve the contact chance between JKF and oil or heavy metal ions, leading to an improvement of its sorption capacity.30
Table 2 Particle-size distribution and adsorption properties of JKF, JKF-E, JKF-EC, and JKF-EAa
Properties |
JKF |
JKF-E |
JKF-EC |
JKF-EA |
Different small letters (a–d) in the same line indicate significant difference (p < 0.05). |
D3,2 (μm) |
132.94 ± 3.62a |
73.35 ± 4.27c |
87.99 ± 3.39b |
63.77 ± 1.53d |
Surface area (m2 kg−1) |
51.46 ± 1.28d |
129.22 ± 5.68b |
114.95 ± 1.73c |
146.37 ± 3.72a |
Water-absorption capacity (g g−1) |
4.46 ± 0.30c |
7.73 ± 0.28b |
10.66 ± 0.45a |
6.47 ± 0.49b |
Water-expansion ability (mL g−1) |
4.20 ± 0.17c |
6.42 ± 0.16b |
8.50 ± 0.20a |
5.95 ± 0.20b |
Viscosity (cp) |
9.83 ± 0.04c |
14.34 ± 0.12b |
17.93 ± 0.84a |
16.71 ± 0.22a |
Surface hydrophobicity |
36.50 ± 3.44b |
28.65 ± 2.59c |
22.42 ± 1.62d |
43.57 ± 3.36a |
Oil-adsorption ability (g g−1) |
1.56 ± 0.21c |
1.32 ± 0.07c |
2.62 ± 0.12b |
4.47 ± 0.34a |
Adsorbing capacity of nitrite ions (μmol g−1) |
3.37 ± 0.17c |
10.57 ± 0.34a |
9.69 ± 0.32a |
7.24 ± 0.33b |
Equilibrium sorption amount of Pb2+ (mg g−1) |
12.43 ± 0.35d |
17.22 ± 1.52c |
29.85 ± 1.64a |
25.61 ± 1.44b |
Equilibrium sorption amount of Cu2+ (mg g−1) |
11.44 ± 1.08d |
15.97 ± 0.98c |
23.47 ± 1.33b |
19.69 ± 1.99a |
3.3. Effects of different modifications on the structure of JKF
3.3.1 Changes in the microstructure. As shown in Fig. 1A, JKF offered a relatively smooth microstructure surface with some fragments. By contrast, JKF-E, JKF-EA, and JKF-EC all showed irregular and fragmented microstructures with a large amount of pores (Fig. 1B–D). Mixed enzymatic hydrolysis and acetylation or carboxymethylation can all break down the cell wall of JKF or crack its polysaccharide chains, causing a loss of fat, cellulose, and lignin, while also increasing fragmentation and the pores in the microstructure of JKF.7,34 The fragmented and porous microstructures of JKF-E, JKF-EA, and JKF-EC were in accordance with their larger surface area and smaller particle sizes (Table 2). Moreover, fibres with a porous microstructure always exhibit higher sorption capacities for oil, sugar, and heavy metals.5,16
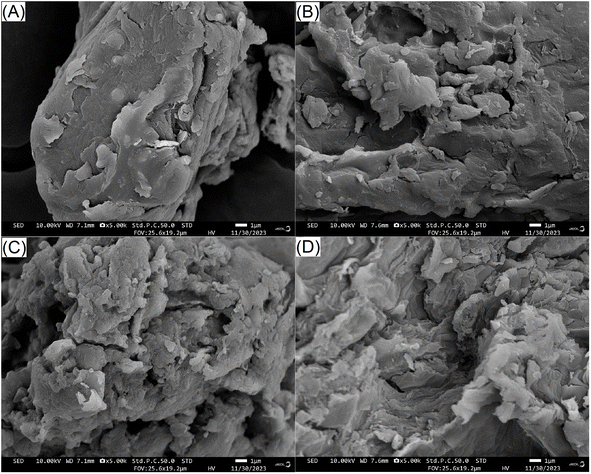 |
| Fig. 1 Scanning electron microscopy images of JKF (A), JKF-E (B), JKF-EA (C), and JKF-EC (D) with a magnification of 5000× at 1 μm. JKF, jujube kernel fibre; JKF-E, JKF modified via mixed enzymatic hydrolysis; JKF-EA, JKF treated via mixed enzymatic hydrolysis and acetylation; and JKF-EC, JKF treated via mixed enzymatic hydrolysis combined with carboxymethylation. | |
3.3.2 Fourier-transformed infrared spectroscopy. Fig. 2 shows the FT-IR spectra of JKF, JKF-E, JKF-EA, and JKF-EH, where visible differences can be seen among them. Compared with the spectrum of JKF, a new peak at around 860 cm−1 appeared in the spectra of JKF-E, JKF-EA, and JKF-EH, evidencing the degradation of the β-glycosidic bond caused by the mixed enzymolysis.30 An obvious red-shift occurred for the peak located at the wavenumber of around 3300 cm−1 in the spectra of JKF-E, JKF-EA, and JKF-EH, suggesting that mixed enzymolysis, and mixed enzymolysis assisted with acetylation or carboxymethylation changed the hydrogen bonds of JKF.35 Moreover, the peaks at wavenumbers of 1760 and 1046, 1500 cm−1 (indicative of the asymmetric bend of acetyl groups, C–O–C, and –C
O, respectively) in the spectrum of JKF separately appeared at 1762, 1037, and 1508 cm−1 in the spectrum of JKE-SGEA,6 demonstrating that acyl ester groups had been introduced into the JKF molecules after acetylation. Additionally, a new peak appeared compared with the spectrum of JKF, with the spectrum of JKF-EC showing this new peak at around 964 cm−1, which was attributed to the introduction of the carboxymethyl group after carboxymethylation.2 Previous studies have shown that both acetylation and carboxymethylation could influence the structure of the fibres by changing their chemical bonds.23,24
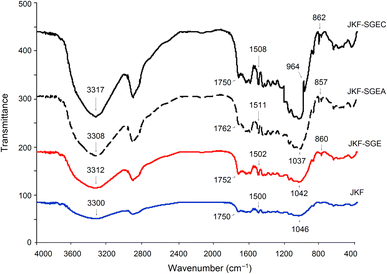 |
| Fig. 2 Fourier-transformed infrared spectra of JKF, JKF-E, JKF-EA, and JKF-EC. | |
3.4. Water adsorption and expansion capacities
High WAC and WEA mean fibres have a larger expansion volume in wastewater, which is helpful for the adsorption of heavy metal ions, oil, sugar, and nitrite ions on the fibres.36 The results in Table 2 show that mixed enzymatic hydrolysis, and mixed enzymatic hydrolysis combined with carboxymethylation or acetylation all showed enhanced WACs and WEAs compared to JKF (p < 0.05), which could be mainly attributed to the improvement of the soluble fibre content and the changes in the surface area and microstructure of JKF after these modifications. Their higher soluble fibre contents (Table 1) mean that JKF-E, JKF-EA, and JKF-EC have a stronger affinity with water molecules than JKF. Moreover, the larger surface area and more porous microstructures (Fig. 1B, C and Table 2) can ensure that JKF-E, JKF-EA, and JKF-EC have more contact chance and stronger interactions with water molecules.11
JKF-EC exhibited the highest WAC (10.66 g g−1) and the largest expansion volume (8.50 mL g−1) among these JKFs, corresponding to its highest soluble fibre content (18.32 ± 0.82 g 100 g−1), evidencing that mixed enzymatic hydrolysis combined with carboxymethylation was more effective for improving the water adsorption and expansion capacities of JKF than mixed enzymatic hydrolysis alone. The main reason for this was that after carboxymethylation, the introduced carboxymethyl groups can significantly increase the polarity of JKF, and enhance the steric hindrance between its polysaccharide chains, both of which play an important role in the WAC and WEA of the fibres.1 Moreover, JKF-EA also had a higher WAC and WEA than JKF (p < 0.05), but these values were not significantly different from those of JKF-E (p > 0.05), probably due to the introduced acyl ester groups having a relatively high hydrophobicity rather than hydrophilicity.14 A higher WEA is helpful for JKF to expand its structure, and contact and adsorb pollutants in effluents.
3.5. Surface hydrophobicity
Surface hydrophobicity is positively correlated with the chemical adsorption of hydrophobic harmful substances in wastewater on fibres.1 The results in Table 2 show that the surface hydrophobicities of JKF-E and JKF-EC were both lower than that of JKF (p < 0.05), highlighting that mixed enzymolysis and mixed enzymolysis combined with carboxymethylation both reduced the hydrophobicity of JKF. Mixed enzymatic hydrolysis causes degradation of the glycosidic bonds of JKF and releases more hydrophilic groups,10,37 and the introduced phosphate groups increases the hydrophilicity of JKF,15 which all contribute to the lower surface hydrophobicities of JKF-E and JKF-EC. However, a lower surface hydrophobicity is not conducive to the treatment of oil wastewater. In contrast, the surface hydrophobicity of JKF-EA was significantly higher than that of JKF (p < 0.05), because the introduced acyl ester group after acetylation have a relatively high hydrophobicity.29 Previous studies have found that acetylation improved the surface hydrophobicity of banana and oil palm empty fruit bunch fibres, too.14,38
3.6. Adsorption capacity
3.6.1 OAA of JKFs. JKF showed a poor OAA (1.56 ± 0.21 g g−1, Table 2), which was consistent with the results of Xia et al.39 JKF-EA and JKF-EC both offered a stronger adsorption affinity to oil (OAA: 2.62–4.47 g g−1) than JKF (p < 0.05); while the OAA of JKF-E was lower than that of JKF (p > 0.05), evidencing that acetylation and carboxymethylation could improve the OAA of JKF, but mixed enzymatic hydrolysis reduced it. The main reason for the high OAA of JKF-EA was that the introduced acyl ester groups after acetylation significantly increases the surface hydrophobicity of JKF (Table 2) and thus enhances the OAA of JKF. Moreover, the increment in surface area and WEC (Table 2), and more porous microstructure (Fig. 1C) after acetylation are all helpful for the adsorption of oil on JKF-EC.40 Asadpour et al. also found that the OAA of oil palm empty fruit bunch fibre could be significantly improved by acetylation.29 Other substances with a porous structure, such as active carbon, exhibit excellent oil-adsorption capacity, too.41 With respect to JKF-EC, the high OAA was mainly attributed to its larger surface area, higher WEC, and porous microstructure (Table 2 and Fig. 1D). A higher WEC indicates that DFs have a larger expansion volume in wastewater, which is conducive for the adsorption of oil on DFs.16 By contrast, although having a bigger WEC and porous microstructure (Table 2 and Fig. 1B), JKF-E showed a poor OAA, mainly due to its lower surface hydrophobicity (28.65 ± 2.59, Table 2). It has been shown that fibres can adsorb oil via a chemical adsorption mechanism or physical adsorption patterns.13 The results in the current study demonstrated that oil molecules can be adsorbed by JKF-E, JKF-EA, or JKF-EC through both chemical and physical adsorption mechanisms. However, their true effects on the oil in effluents need further study.
3.6.2 ACNI of JKFs. Nitrite in industrial effluents may contaminate drinking water, and a high concentration of nitrites poses a risk to human health, potentially resulting in nitrite poisoning and various digestive tract tumours.30 Compared with JKF, the modified JKFs (JKF-E, JKF-EC, and JKF-EA) all offered a higher ACNI (7.24–10.57 μmol g−1) (p < 0.05), reflecting that all three mixed modifications improved the ACNI of JKF. It has been evidenced that nitrite ions are unstable in water, and can react with hydrogen ions to form nitrates, which in turn form nitrogen oxides. Organic acids in fibres, especially phenolic, uronic, and carboxylic acids, can quickly combine with these nitrogen oxides.5 Therefore, the higher extractable polyphenol content (Table 1) was one reason for the higher ACNI of JKF-E, JKF-EC, and JKF-EA. Apart from that, a larger WEA and surface area (Table 2), and more fragmented and porous microstructure (Fig. 1B–D) can increase the contact chance and affinity between nitrite ions and JKFs.36 Both JKF-E and JKF-EC offered the highest ACNI values (10.57 and 9.69 μmol g−1, respectively) among these samples, in accordance with their high extractable polyphenols (12.65 and 9.56 mg g−1, respectively, Table 1). JKF-EC exhibited a higher ACNI than that of JKF-EA, because the introduced carboxymethyl group has a relatively stronger affinity with nitrite ions than the acetyl ester group.2 Zheng et al. found that carboxymethylation and acetylation both can improve the ACNI of millet fibre, too.15 The high ACNI of JKF-E, JKF-EC, and JKF-EA (7.24–10.57 μmol g−1) suggested their potential ability to reduce the risk of nitrite contamination in effluents.1
3.6.3 ACLI of JKFs. Lead pollution worsens water quality, threatens the survival of aquatic organisms, and can cause Minamata disease.42 During tests, it was found that within 5–80 min, the adsorption of lead ions(II) on JKF, JKF-E, JKF-EA, and JKF-EC was time dependent (Fig. 3). The ACLI of these JKFs all reached an equilibrium value at around 95 min. Moreover, the results in Table 2 and Fig. 2 show that mixed enzymatic hydrolysis, and mixed enzymatic hydrolysis combined with carboxymethylation or acetylation improved the ACLI of JKF, as JKF-E, JKF-EA, and JKF-EC all exhibited a higher equilibrium ACLI than JKF (p < 0.05). After these composite modifications, the improved WEA, increased polyphenol content and surface area, and more porous microstructure (Tables 1, 2, and Fig. 1B–D) all supported JKF to adsorb more lead ions. The increase in the WEA or surface area improves the volume or area of JKF that can contact Pb2+ in water; while fibres with a porous microstructure have a higher capacity to capture Pb2+.8 More importantly, JKF-EC offered the highest ACLI (29.85 ± 1.64 mg g−1), mainly due to the introduction of carboxymethyl groups. The negative polarity of JKF was significantly enhanced by the introduced carboxymethyl groups after carboxymethylation. Since negative polarity plays a key role in the adsorption of metal ions on fibres,1 the introduced carboxymethyl groups remarkably improved the ACLI of JKF-EC (p < 0.05). Additionally, JKF-E, JKF-EA, and JKF-EC all showed a higher Pb2+-adsorbing capacity than phytic acid-treated corn starch and acetylated banana peel fibre (10.45 and 15.32 mg g−1, respectively),2,5 highlighting their potential usage as renewable and environmentally friendly adsorbents for lead ions in effluents.
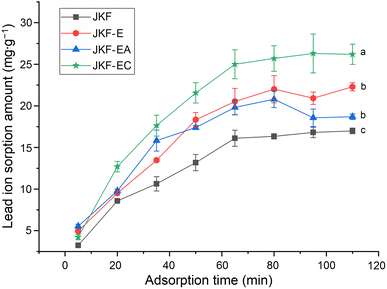 |
| Fig. 3 Isothermal adsorption kinetics of lead ions(II) on JKF, JKF-E, JKF-EA, and JKF-EC. Different lowercase letters (a–c) near the lines indicate significant difference (p < 0.05). | |
3.6.4 Adsorption capacity of copper ions(II) on JKFs. Metal mining, smelting, and manufacturing industries produce a large number of wastes containing excessive copper ions (Cu2+), which can cause a poor growth of crops, contaminate drinking water, and damage the liver and other organs of humans and animals.8 The copper isotherm adsorption kinetics profiles of the JKFs are shown in Fig. 4, and their equilibrium ACCI is shown in Table 2. It is obvious that the adsorptions of copper ions on JKF, JKF-E, JKF-EA, and JKF-EC were all time dependent during 0–60 min, and these JKFs all showed an equilibrium ACLI at approximately 60 min. All the composite modifications significantly enhanced the adsorbing capacity of JKF to copper ions because of their higher ACLI (p < 0.05) (Table 2). After these composite modifications, the surface area and soluble fibre content were all increased (Tables 1 and 2), and the microstructure became more porous (Fig. 1B–D), which were all conducive for copper adsorption by JKF. More importantly, more functional groups, especially phenolic and carboxyl groups, were exposed after the hydrolysis with cellulase, hemicellulase, and laccase. These functional groups can considerably enhance the binding power of JKF to copper ions.7 Meanwhile, the equilibrium ACLI of JKF-EA and JKF-EC were both higher than that of JKF-E (p < 0.05), and JKF-EA and JKF-EC both showed a higher ACLI than JKF-E (p < 0.05) (Table 2), showing that mixed enzymatic hydrolysis combined with carboxymethylation or acetylation were more effective at improving the ACLI of JKF than mixed enzymatic hydrolysis alone. The introduced carboxymethyl group and acetyl ester group both can increase the negative polarity of JKF, which plays an important role in the chelation of fibres with copper ions.1 Since carboxymethyl groups have a higher binding power with copper ions than acetyl ester groups,15 the ACLI of JKF-EC (23.47 ± 1.33 mg g−1) was higher than that of JKF-E (p < 0.05). Additionally, the ACLI of JKF-E, JKF-EA, and JKF-EC (15.97–23.47 mg g−1) was higher than that of rice bran fibre (4.88 mg g−1) and millet bran fibre (13.66 mg g−1),3,15 highlighting their potential usage as absorbents for wastewater purification.
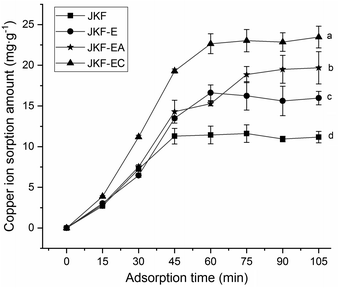 |
| Fig. 4 Isothermal adsorption kinetics of copper ions(II) on JKF, JKF-E, JKF-EA, and JKF-EPG. Different lowercase letters (a–d) near the lines indicate significant difference (p < 0.05). | |
4 Conclusions
Mixed enzymatic hydrolysis alone or combined with acetylation or carboxymethylation increased the surface area and made the microstructure of JKF more porous, and improved its soluble fibre content, and water adsorption and expansion capacities. Mixed enzymatic hydrolysis combined with acetylation remarkably enhanced the surface hydrophobicity and oil-adsorption ability of JKF; while mixed enzymatic hydrolysis combined with carboxymethylation significantly increased the solubility and hydration properties of JKF, and improved its chelating abilities toward glucose, copper, and lead ions. Moreover, mixed enzymatic hydrolyzed JKF showed the highest nitrite ions-adsorption ability. Thus, JKF-EC, JKF-EA, and JKF all exhibited potential as wastewater adsorbents. However, the adsorption kinetics and mechanism of these modified JKFs on pollutants in wastewater, and the chemical oxygen demand of the effluents after the treatment of JKFs at the optimum conditions need further study.
Data availability
The data supporting this article have been included as part of the manuscript.
Author contributions
Nan Qin: conceptualization, writing – original draft, funding acquisition. Yunfei Li: investigation, methodology, writing – original draft. Lan Zhang: investigation, methodology. Lili Guo: validation, writing—review and editing; Wenfang Zhang: investigation, methodology. Guanwen Li: validation, writing—review and editing. Jun Bai: methodology, validation.
Conflicts of interest
There are no conflicts to declare.
Acknowledgements
This work was supported by the Shanxi University of Chinese Medicine Science and Technology Innovation Team (2022TD2008), and the special fund for Science and Technology Innovation Teams of Shanxi Province (202304051001043).
References
- F. Haq, S. Mehmood, M. Haroon, M. Kiran, K. Waseem, T. Aziz and A. Farid, Role of starch based materials as a bio-sorbents for the removal of dyes and heavy metals from wastewater, J. Polym. Environ., 2022, 30(5), 1730–1748, DOI:10.1007/s10924-021-02337-6.
- A. Akinterinwa, E. Oladele, A. Adebayo, E. Gurgur, O. O. Iyanu and O. Ajayi, Cross-linked-substituted (esterified/etherified) starch derivatives as aqueous heavy metal ion adsorbent: A review, Water Sci. Technol., 2020, 82(1), 1–26, DOI:10.2166/wst.2020.332.
- R. T. Prabha and T. H. Udayashankara, Adsorption of copper metal ions from aqueous solution using rice husk and groundnut shell, Int. J. Sci. Res., 2014, 8, 705–709, DOI:10.1080/19443994.2014.914446.
- N. A. Abd El-Ghany, M. H. A. Elella, H. M. Abdallah, M. S. Mostafa and M. Samy, Recent advances in various starch formulation for wastewater purification via adsorption technique: A review, J. Polym. Environ., 2023, 31(7), 2792–2825, DOI:10.1007/s10924-023-02798-x.
- S. R. Kim, J. Y. Park and E. Y. Park, Effect of ethanol, phytic acid and citric acid treatment on the physicochemical and heavy metal adsorption properties of corn starch, Food Chem., 2024, 431, 137167, DOI:10.1016/j.foodchem.2023.137167.
- D. I. L. Gil-López, J. A. Lois-Correa, M. E. Sánchez-Pardo, M. A. Domínguez-Crespo, A. M. Torres-Huerta, A. E. Rodríguez-Salazar and V. N. Orta-Guzmán, Production of dietary fibers from sugarcane bagasse and sugarcane tops using microwave-assisted alkaline treatments, Ind. Crops Prod., 2019, 135, 159–169, DOI:10.1016/j.indcrop.2019.04.042.
- D. Zadeike, R. Vaitkeviciene, R. Degutyte, J. Bendoraitiene, Z. Rukuiziene and D. Cernauskas, et al., A comparative study on the structural and functional properties of water-soluble and alkali-soluble dietary fibers from rice bran after hot-water, ultrasound, hydrolysis by cellulase, and combined pre-treatments, Int. J. Food Sci. Technol., 2021, 57(2), 15480, DOI:10.1111/ijfs.15480.
- Z. Raji, A. Karim, A. Karam and S. Khalloufi, A review on the heavy metal adsorption capacity of dietary fibers derived from agro-based wastes: Opportunities and challenges for practical applications in the food industry, Trends Food Sci. Technol., 2023, 137, 74–91, DOI:10.1016/j.tifs.2023.05.004.
- L. Zhu, B. Yu, H. Chen, J. Yu, H. Yan, Y. Luo, J. He, Z. Huang, P. Zheng, X. Mao, J. Luo and D. Chen, Comparisons of the micronization, steam explosion, and gamma irradiation treatment on chemical composition, structure, physicochemical properties, and in vitro digestibility of dietary fiber from soybean hulls, Food Chem., 2022, 366, 130618, DOI:10.1016/j.foodchem.2021.130618.
- H. Zohaib, I. Muhammad, A. M. Haseeb and K. M. Kamran, Ultrasound-Assisted Modification of Insoluble Dietary Fiber from Chia (Salvia hispanica L.) Seeds, J. Food Qual., 2021, 5035299, DOI:10.1155/2021/5035299.
- Y. Liu, S. Yi, T. Ye, Y. Leng, M. A. Hossen, D. E. Sameen and W. Qin, Effects of superfine-grinding and homogenization on physicochemical properties of okara dietary fibers for 3D printing cookies, Ultrason. Sonochem., 2021, 77, 105693, DOI:10.1016/j.ultsonch.2021.105693.
- S. Zhang, Z. Dong, J. Shi, C. Yang, Y. Fang, G. Chen, H. Chen and C. Tian, Enzymatic hydrolysis of corn stover lignin by laccase, lignin peroxidase, and manganese peroxidase, Bioresour. Technol., 2022, 361, 127699, DOI:10.1016/j.biortech.2022.127699.
- J. Y. Si, C. R. Yang, Y. Chen, J. H. Xie, S. L. Tian, Y. A. Cheng, X. B. Hu and Q. Yu, Structural properties and adsorption capacities of Mesona chinensis Benth residues dietary fiber prepared by cellulase treatment assisted by Aspergillus niger or Trichoderma reesei, Food Chem., 2023, 407, 135149, DOI:10.1016/j.foodchem.2022.135149.
- K. Rani, T. Gomathi, K. Vijayalakshmi, M. Saranya and P. N. Sudha, Banana fiber Cellulose Nano Crystals grafted with butyl acrylate for heavy metal lead (II) removal, Int. J. Biol. Macromol., 2019, 131, 461–472, DOI:10.1016/j.ijbiomac.2019.03.064.
- Y. Zheng, B. Xu, P. Shi, H. Tian, Y. Li, X. Wang, S. Wu and P. Liang, The influences of acetylation, hydroxypropylation, enzymatic hydrolysis and crosslinking on improved adsorption capacities and in vitro hypoglycemic properties of millet bran dietary fibre, Food Chem., 2022, 368, 130883, DOI:10.1016/j.foodchem.2021.130883.
- P. Kanwar, R. B. Yadav and B. S. Yadav, Cross-linking, carboxymethylation and hydroxypropylation treatment to sorghum dietary fiber: Effect on physicochemical, micro structural and thermal properties, Int. J. Biol. Macromol., 2023, 233, 123638, DOI:10.1016/j.ijbiomac.2023.123638.
- W. Cai, F. Tang, Z. Guo, Q. Zhang, X. Zhao, M. Ning and C. Shan, Effects of pretreatment methods and leaching methods on jujube wine quality detected by electronic senses and HS–SPME–GC–MS, Food Chem., 2020, 330, 127330, DOI:10.1016/j.foodchem.2020.127330.
- P. Agrawal, T. Singh, D. Pathak and H. Chopra, An updated review of Ziziphus jujube: Major focus on its phytochemicals and pharmacological properties, Pharmacol. Res. – Mod. Chin. Med., 2023, 8, 100297, DOI:10.1016/j.prmcm.2023.100297.
- L. Bai, H. Zhang, Q. Liu, Y. Zhao, X. Cui, S. Guo, L. Zhang, C. T. Ho and N. Bai, Chemical characterization of the main bioactive constituents from fruits of Ziziphus jujube, Food Funct., 2016, 7, 2870–2877, 10.1039/C6FO00613B.
- A. K. Rashwan, N. Karim, M. R. I. Shishir, T. Bao, Y. Lu and W. Chen, Jujube fruit: A potential nutritious fruit for the development of functional food products, J. Funct. Foods, 2020, 75, 104205, DOI:10.1016/j.jff.2020.104205.
- X. Ji, C. Hou, Y. Yan, M. Shi and Y. Liu, Comparison of structural characterization and antioxidant activity of polysaccharides from jujube (Ziziphus jujuba Mill.) fruit, Int. J. Biol. Macromol., 2020, 149, 1008–1018, DOI:10.1016/j.ijbiomac.2020.02.018.
- M. Al-Khalili, N. Al-Habsi, A. Al-Alawi, L. Al-Subhi, M. T. Z. Myint, M. Al-Abri, M. I. Waly, S. Al-Harthi, A. Al-Mamun and M. S. Rahman, Structural characteristics of alkaline treated fibers from date-pits: Residual and precipitated fibers at different pH, Bioact. Carbohydr. Diet. Fibre, 2021, 25, 100251, DOI:10.1016/j.bcdf.2020.100251.
- M. Y. Zhang, A. M. Liao, K. Thakur, J. H. Huang, J. G. Zhang and Z. J. Wei, Modification of wheat bran insoluble dietary fiber with carboxymethylation, complex enzymatic hydrolysis and ultrafine comminution, Food Chem., 2019, 297, 124983, DOI:10.1016/j.foodchem.2019.124983.
- Y. Zheng, J. Li, X. Wang, M. Guo, C. Cheng and Y. Zhang, Effects of three biological combined with chemical methods on the microstructure, physicochemical properties and antioxidant activity of millet bran dietary fibre, Food Chem., 2023, 411, 135503, DOI:10.1016/j.foodchem.2023.135503.
- AOAC, Official Methods of Analysis, Association of Official Analytical Chemists, Washington, DC, 2020 Search PubMed.
- V. V. Cecilia, G. V. R. María, A. R. Carolina, L. C. Jorge, G.-G. Teresa, A. Roberto, O. P. García, J. L. Rosado, C. M. López-Sabater, A. I. Castellote, H. M. A. Monteayor and K. d. l. T. Carbot, Total phenolic compounds in milk from different species. Design of an extraction technique for quantification using the Folin-Ciocalteu method, Food Chem., 2015, 176, 480–486, DOI:10.1016/j.foodchem.2014.12.050.
- Y. J. Zheng and Y. Li, Physicochemical and functional properties of coconut (Cocos nucifera L) cake dietary fibres: Effects of cellulase hydrolysis, acid treatment
and particle size distribution, Food Chem., 2018, 257, 135–142, DOI:10.1016/j.foodchem.2018.03.012.
- K. He, Q. Li, Y. Li, B. Li and S. Liu, Water-insoluble dietary fibers from bamboo shoot used as plant food particles for the stabilization of O/W Pickering emulsion, Food Chem., 2020, 310, 125925, DOI:10.1016/j.foodchem.2019.125925.
- R. Asadpour, S. Yavari, H. Kamyab, V. Ashokkumar, S. Chelliapan and A. Yuzir, Study of oil sorption behaviour of esterified oil palm empty fruit bunch (OPEFB) fibre and its kinetics and isotherm studies, Environ. Technol. Innovation, 2021, 22, 101397, DOI:10.1016/j.eti.2021.101397.
- M. Hua, J. Lu, D. Qu, C. Liu, L. Zhang, S. Li, J. Chen and Y. Sun, Structure, physicochemical properties and adsorption function of insoluble dietary fiber from ginseng residue: A potential functional ingredient, Food Chem., 2019, 286, 522–529, DOI:10.1016/j.foodchem.2019.01.114.
- K. Gao, T. Liu, Q. Zhang, Y. Wang, X. Song, X. Luo, R. Ruan, L. Deng, X. Cui and Y. Liu, Stabilization of emulsions prepared by ball milling and cellulase treated pomelo peel insoluble dietary fiber: Integrity of porous fiber structure dominates the stability, Food Chem., 2024, 440, 138189, DOI:10.1016/j.foodchem.2023.138189.
- R. Nasseri, R. Ngunjiri, C. Moresoli, A. Yu, Z. Yuan and C. Xu, Poly(lactic acid)/acetylated starch blends: Effect of starch acetylation on the material properties, Carbohydr. Polym., 2020, 229, 115453, DOI:10.1016/j.carbpol.2019.115453.
- M. Ma and T. Mu, Modification of deoiled cumin dietary fiber with laccase and cellulase under high hydrostatic pressure, Carbohydr. Polym., 2016, 136, 87–94, DOI:10.1016/j.carbpol.2015.09.030.
- X. Qin, C. Yang, J. Si, Y. Chen, J. Xie, J. Tang, X. Dong, Y. Cheng, X. Hu and Q. Yu, Fortified yogurt with high-quality dietary fiber prepared from the by-products of grapefruit by superfine grinding combined with fermentation treatment, LWT–Food Sci. Technol., 2023, 188, 115396, DOI:10.1016/j.lwt.2023.115396.
- B. J. Hazarika and N. Sit, Effect of dual modification with hydroxypropylation and cross-linking on physicochemical properties of taro starch, Carbohydr. Polym., 2016, 140(20), 269–278, DOI:10.1016/j.carbpol.2015.12.055.
- E. Miehle, M. Hass, S. Bader-Mittermaier and P. Eisner, The role of hydration properties of soluble dietary fibers on glucose diffusion, Food Hydrocolloids, 2022, 131, 107822, DOI:10.1016/j.foodhyd.2022.107822.
- E. Backes, C. G. Kato, R. C. G. Corrêa, R. F. P. M. Moreira, R. A. Peralta, L. Barros, I. G. F. R. Ferreira, G. M. Zanin, A. Bracht and R. M. Peralta, Laccases in food processing: Current status, bottlenecks and perspectives, Trends Food Sci. Technol., 2021, 115, 445–460, DOI:10.1016/j.tifs.2021.06.052.
- Y. J. Zheng, Y. Li and H. L. Tian, Effects of carboxymethylation, acidic treatment, hydroxypropylation and heating combined with enzymatic hydrolysis on structural and physicochemical properties of palm kernel expeller dietary fiber, LWT–Food Sci. Technol., 2020, 133, 109909, DOI:10.1016/j.lwt.2020.109909.
- X. Xia, F. Li, H. Ran, J. Zhao, X. Lei, L. Lei, J. Wen, G. Xiao, K. Zeng and J. Ming, Effect of jujube kernel powder addition on moisture absorption performance, color stability, texture properties and agglomeration characteristics of jujube powder, LWT–Food Sci. Technol., 2023, 174, 114452, DOI:10.1016/j.lwt.2023.114452.
- J. Q. Sang, L. Li, J. Wen, H. C. Liu, J. J. Wu, Y. S. Yu, Y. J. Xu, Q. Gu, M. Fu and X. Lin, Chemical composition, structural and functional
properties of insoluble dietary fiber obtained from the Shatian pomelo peel sponge layer using different modification methods, LWT–Food Sci. Technol., 2022, 165, 113737, DOI:10.1016/j.lwt.2022.113737.
- A. Campos, A. R. Sena Neto, V. B. Rodrigues, B. R. Luchesi, J. M. Mattoso and J. M. Marconcini, Effect of raw and chemically treated oil palm mesocarp fibers on thermoplastic cassava starch properties, Ind. Crops Prod., 2018, 124, 149–154, DOI:10.1016/j.indcrop.2018.07.075.
- F. A. Borges, L. M. Costa, C. R. T. Tarley, G. de Fatima Lima Martins and E. C. Figueiredo, Lead determination in commercial juice samples by direct magnetic sorbent sampling flame atomic absorption spectrometry (DMSS-FAAS), Food Chem., 2023, 413, 135676, DOI:10.1016/j.foodchem.2023.135676.
|
This journal is © The Royal Society of Chemistry 2024 |
Click here to see how this site uses Cookies. View our privacy policy here.