DOI:
10.1039/D4RA04882B
(Paper)
RSC Adv., 2024,
14, 27215-27226
A dual-function chromogenic and fluorogenic benzofurazan probe for plazomicin and its innovative utility for development of two microwell assays with high throughput for analysis of drug substance and pharmaceutical formulations†
Received
6th July 2024
, Accepted 20th August 2024
First published on 27th August 2024
Abstract
Plazomicin (PLZ) is a novel aminoglycoside which has been recently approved by The US Food and Drug Administration for the treatment of complicated urinary tract infections including acute pyelonephritis, caused by certain Enterobacteriaceae, in adult patients with limited or no options for alternative treatment. This study focuses on the development of microwell-based photometric and fluorometric assays for the quantitative determination of PLZ in its bulk drug substance and commercial pharmaceutical formulations (Zemedri® injections). Both assays utilize the dual-function chromogenic and fluorogenic properties of the 4-fluoro-7-nitrobenzofurazan (NBD-F) probe. The reaction between PLZ and NBD-F, conducted in a borate buffer at pH 8, resulted in the formation of a colored and fluorescent reaction product. The product exhibited maximum light absorption at 473 nm and emitted fluorescence at 541 nm when excited at 473 nm. Factors influencing the reaction between PLZ and NBD-F were thoroughly investigated, and optimal conditions were determined. Under the optimized reaction conditions, calibration curves were generated to establish the relationship between absorbance and fluorescence intensities of the reaction product with the corresponding PLZ concentrations. The absorbance–concentration relation was linear in a PLZ concentration range of 20–800 μg mL−1 with a limit of quantitation of 25 μg mL−1, while the fluorescence–concentration relation was linear in the concentration range of 0.05–1.5 μg mL−1 with a limit of quantitation of 0.08 μg mL−1. Both assays underwent validation and were successfully applied to the quantitation of PLZ in its bulk drug substance and pharmaceutical formulations (injections) with satisfactory accuracy and precision. The eco-friendliness/greenness assessment of the assays demonstrated that both assays comply with the requirements of green analytical chemistry approaches. Furthermore, the proposed microwell assay plates allowed for the simultaneous handling of numerous samples with micro-volumes, enabling high-throughput analysis. In conclusion, this study represents the first evaluation of NBD-F as a dual-function probe for the microwell-based photometric and fluorometric determination of PLZ. The developed assays serve as valuable analytical tools for the quality control of PLZ's bulk drug substance and pharmaceutical formulations.
1. Introduction
Plzomicin (PLZ, formerly designated as ACHN-490) is a novel semi-synthetic aminoglycoside derivative of the naturally occurring aminoglycoside sisomicin. PLZ has enhanced broad spectrum antibacterial activity against multi-drug resistant Gram-negative and Gram-positive pathogens, including those producing extended spectrum β-lactamase and carbapenemase enzymes.1 The chemical structure of PLZ is given in Fig. 1, and its molecular formula is C25H48N6O10. PLZ was developed by Achaogen Biopharmaceuticals (San Francisco, CA, USA) via appending hydroxylaminobutyric acid and 2-hydroxyethyl function groups to sisomicin at position 1 and 6′, respectively. This structure alteration was designed to retain activity against all relevant aminoglycoside-modifying enzymes, which contribute to the main resistance mechanism for the other available aminoglycoside therapy.2 PLZ has been recently approved by The US Food and Drug Administration (FDA) for the treatment of complicated urinary tract infections including acute pyelonephritis, caused by certain Enterobacteriaceae, in adult patients with limited or no options for alternative treatment.3 It is marketed under the trade name of Zemdri® injection and is administered via once-daily intravenous infusion.4 PLZ exerts its bactericidal action by binding to bacterial 30S ribosomal subunits. It demonstrated a favorable minimum inhibitory concentration value compared to other aminoglycosides such as gentamicin, amikacin, and tobramycin. PLZ has a better safety profile than other aminoglycosides as it has demonstrated a lower level of nephrotoxicity and rarely ototoxicity, the main serious side effects of aminoglycosides.1,5
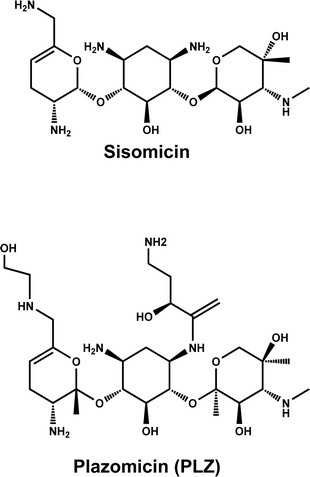 |
| Fig. 1 The chemical structures of sisomicin and plazomicin (PLZ). | |
To ensure effective and safe therapy using PLZ, its pharmaceutical formulation (Zemdri® injection) must possess high-quality in terms of an exact content of the active drug substance. Currently, there is only one available assay in the literature for analyzing PLZ and its impurities in bulk drug substances, which involves liquid chromatography coupled with an on-line mass spectrometry detector. However, there is currently no assay available for analyzing the exact drug content of Zemdri® injection. This study aims to address this gap by developing validated assays for determining the amount of PLZ in Zemdri® injection. It is well established that liquid chromatographic assays (HPLC) offer excellent accuracy and selectivity in diverse fields of analysis, it has the capability to separate complex samples which simple photometric and fluorometric methods lack, and that this added capability comes with the environmental cost of using greater amounts of solvents. Thus, there exists a class of samples (simple solutions with a limited number of solutes) for which the separation capabilities of HPLC are not needed, and for these it is environmentally advantageous, and advantageous in terms of throughput to use simpler direct photo- and fluorometric methods.6,7 Furthermore, the development of either HPLC or spectrometric (photo- and fluorometric) assays for PLZ is challenging due to the absence of chromophore and fluorophore. Therefore, derivatization procedures are required for development of either of the techniques.8,9 Based on the simplicity of sample matrices (bulk drug form and pharmaceutical formulation) targeted in this study for PLZ, it is crucial to develop photo- and fluorometric assays for determining the amount of PLZ in both its bulk form and formulation. These assays are particularly valuable because they can be easily automated with analyzers, allowing for the sequential analysis of numerous samples. They are especially useful in evaluating pharmaceutical formulations, especially for assessing the contents of drug substances formulations.10,11
The conventional spectrometric assays relying on a manual approach using volumetric flasks or cuvettes, result in the consumption of significant amounts of samples and solvents and imposed limitations on analytical throughput.10 Hence, it was essential to develop an alternative innovative assay that is environmentally friendly and offers enhanced capabilities for high throughput analysis. Microwell spectrometric assays have emerged as a notable environmentally friendly approach in pharmaceutical analysis.12,13 One of the key advantages of this methodology is its ability to utilize smaller volumes of samples and reagents compared to traditional spectrometric practices. This not only reduces waste generation but also lowers the costs associated with solvents and reagents. Moreover, the adoption of microscale techniques in the laboratory setting allows for a reduction in the use of hazardous chemicals and solvents, promoting sustainability. The versatility of microwell spectrometric assays enables their application across various areas of the pharmaceutical industry. Furthermore, this technique is well-suited for automation, leading to improved efficiency, reduced errors, and savings in time and labor. These advantages have significantly contributed to the growing popularity of microwell spectrometry within the pharmaceutical industry.14–16
This study describes, for the first time, the development of two microwell spectrometric (photometric and fluorometric) assays for the determination of PLZ in bulk form and Zemdri® injection. These assays were designated as MW-PM and MW-FL assays, respectively. Both assays involved the microscale formation of a dual function chromogenic and fluorogenic derivative upon reaction of PLZ with 4-fluoro-7-nitrobenzofurazan (NBD-F) probe. The analysis procedures were conducted in 96-well plates and the absorbances (in MW-PM) or fluorescence (in MW-FL) of the reaction's solutions were measured by a multifunction microplate reader. The absorbances were measured at 473 nm, and the emitted fluorescence signals were measured at 541 nm after excitation at 473 nm. Both MW-PM and MW-FL assays meet the requirements of green and high-throughput analytical approaches used for the quality control of PLZ in pharmaceutical industry.
2. Experimental
2.1. Apparatus
A double-beam, ultraviolet-visible spectrophotometer (model UV-1800: Shimadzu Corporation, Kyoto, Japan) with 1 cm quartz cells was used for recording the absorption spectra. A fluorescence spectrometer (model FP-8200: Jasco Corporation, Tokyo, Japan) was used for recording the fluorescence spectra. A slit width of 5.0 nm was employed for both the excitation and emission monochromators. Both instruments underwent regular calibration to ensure their signal linearity and wavelength accuracy, following the instructions provided by the instruments' manufacturers. A multi-mode microplate (absorbance, fluorescence and chemiluminescence) reader (BioTek Synergy HTX, Agilent, Santa Clara, CA, USA) was used for absorbance and fluorescence measurements in both MW-PM and MW-FL assays. The reader has a broad light reading range spanning from 200 to 999 nm and 1 nm increments, offering versatility in measuring at various wavelengths. It is compatible with different microplate formats, ranging from standard 6-well to 384-well, accommodating diverse experimental setups. The instrument's 4-zone system enables precise temperature control, maintaining stability for temperature-sensitive assays at levels up to 50 °C. In addition, it is equipped with an internal shaker that offers three speed settings (low, medium, and high), facilitating efficient mixing of solutions. The data acquisition was achieved utilizing Gen5® software provided with the instrument. A pH microprocessor laboratory pH meter from Mettler-Toledo International Inc. (Zürich, Switzerland) was utilized to adjust pH of buffer solution. Milli-Q water purification system from Labo, Millipore Ltd (Bedford, USA) was used to generate water used throughout the study.
2.2. Chemicals and materials
PLZ was purchased from MedChemExpress (Monmouth Junction, NJ, USA). The purity of PLZ was ≥99%. NBD-F was purchased from Sigma-Aldrich Chemicals Co. (St. Louis, MO, USA). A freshly prepared solution of NBD-F was prepared by dissolving 10 mg of NBD-F in 5 mL of methanol to achieve a concentration of 0.2% (w/v). The borate buffer solution (0.1 M) with a pH of 8 was prepared following a previously described procedure,17 and the pH was adjusted using a pH meter. The ingredients of borate buffer solution were obtained from Fisher Scientific (California, USA). The buffer was prepared by complete dissolving 0.8 g of sodium hydroxide pellets and about 48 g of boric acid in 90 mL of deionised water. The pH of the resulting solution was adjusted to 8 by adding boric acid or sodium hydroxide. After adjusting the pH, the solution was completed to 100 mL with deionised water. From this buffer solution, 50 μL was added to the sample solution in the analysis procedures. The final concentration of buffer in sample solution were 0.025 M. HCl (36%) was purchased from Fisher Scientific (California, USA). The 0.1 M HCl solution was prepared by slowly adding 9.44 mL of concentrated HCl to 90 mL of water in a beaker with stirring during adding the HCl. Then, the volume of solution was completed to 100 mL with water. From this solution, 50 μL was added to the sample solution in the analysis procedures. Zemdri® (Plazomicin) injection, a trademark of Cipla Therapeutics, a division of Cipla USA, Inc. (Warren, NJ, USA), manufactured for Achaogen, Inc. (South San Francisco, CA, USA). The injection was a single-dose vial (10 mL) labelled 500 mg of PLZ per vial (50 mg mL−1), and it was kept refrigerated at 2 °C to 8 °C. Corning® 96-well transparent and white opaque assay plates were acquired from Merck & Co. (Rahway, New Jersey, NJ, USA). The transparent plates were used for MW-PM assay, and the white opaque plates were used for MW-FL assay. Solvents and other materials were obtained from Fisher Scientific (California, USA).
2.3. Preparation of standard and sample solutions
2.3.1. PLZ standard solution. An accurate amount of PLZ weighing 20 mg was transferred quantitatively into a 10 mL calibrated flask. It was then dissolved in 10 mL of water, resulting in a stock solution with a concentration of 2 mg mL−1. This stock solution was subsequently diluted with water to obtain working solutions within the ranges of 20–800 and 0.05–1.5 μg mL−1 for analysis by MW-PM and MW-FL assays, respectively.
2.3.2. Zemedri® injection sample solution. An accurate aliquot (0.5 mL) of Zemedri® injection was transferred into a 10 mL calibrated flask. The volume was then completed to 10 mL with water, resulting in a stock solution with a concentration of 2.5 mg mL−1. This stock solution was subsequently diluted with water to obtain sample solutions within the ranges of 20–800 and 0.05–1.5 μg mL−1 for analysis by MW-PM and MW-FL assays, respectively.
2.4. General procedures of MW-PM and MW-FL assays
Accurate volumes of PLZ solution (50 μL) were transferred into individual wells of a 96-well plate. Following this, 50 μL of borate buffer solution (pH 8 ± 0.5) and 50 μL of NBD-F solution (0.2%, w/v) were added. The reaction mixture was allowed to proceed at room temperature (25 ± 2 °C) for 30 min. Subsequently, 50 μL of 0.1 M HCl was added. For the MW-PM assay, the absorbances of the resulting solutions were measured by the plate reader in absorbance mode at 473 nm. On the other hand, for the MW-FL assay, the fluorescence intensities of the solutions were measured in the reader's fluorescence mode at 541 nm after excitation at 473 nm. Blank wells were treated in the same manner, except that 50 μL of water was added instead of the PLZ sample solution.
2.5. Determination of reaction stoichiometry
The logarithmic limiting method was implemented for the determination of reaction stoichiometry by following the procedures outlined in a previous report.18 Two sets of experiments were conducted using the recommended general procedures mentioned above. In the first set of experiments, increasing concentrations of NBD-F (ranging from 2.37 × 10−1 to 8.19 × 10−1 M) were used while maintaining a fixed PLZ concentration of 6.75 × 10−4 M. On the other hand, the second set of experiments involved increasing concentrations of PLZ (ranging from 3.37 × 10−5 to 1.69 × 10−4M) while keeping the NBD-F concentration at a fixed value of 1.09 × 10−2 M. For the first set of experiments, the obtained absorbance and fluorescence intensities were plotted as functions of the logarithms of NBD-F concentration. Similarly, for the second set of experiments, the absorbance and fluorescence intensities were plotted against the logarithms of PLZ concentration. The slopes of the fitting lines were then calculated for both sets of experiments, from which the reaction molar ratio was determined.
3. Results and discussion
3.1. Strategy for assays development
PLZ, as an organic compound, has a very weak UV-absorption at short wavelengths before the practical region of spectrophotometric analysis without interferences from other species in the solution (e.g., buffer ingredients, etc.). Additionally, it has no fluorescence emission capabilities due to the absence of fluorophoric moieties in its chemical structure (Fig. 2). As a result, direct photometric or fluorometric determination of PLZ is not possible. In order to enable the development of photometric and fluorometric assays for PLZ, its derivatization with a chromogenic or fluorogenic probe is necessary. However, employing a dual-function probe, capable of both chromogenic and fluorogenic reactions, would be more advantageous. This approach allows for the development of both photometric and fluorometric assays using just one probe, offering convenience and efficiency. Benzofurazan derivatives, are a class of compounds with unique photophysical properties that make them highly valuable in photometric and fluorometric analysis, particularly for amine-containing analytes like PLZ. These compounds feature a benzofuran core with a nitro group and either fluorine or chlorine atoms. With their strong absorption and fluorescence characteristics, benzofurazan molecules serve as versatile chromogenic and fluorogenic probes in various analytical applications. They enable the development of sensitive and selective detection assays for a wide range of analytes, thanks to their distinct spectral properties and tunability. As a result, benzofurazan probes have become invaluable tools in the field of analytical chemistry.19–21 In this study, the unique dual-function spectral properties of benzofurazan-based probes were taken into consideration. Both 4-fluoro-7-nitrobenzofurazan (NBD-F) and 7-chloro-4-nitrobenzofurazan (NBD-Cl) are derivatives of benzofurazans that are utilized in a range of applications within photometric and fluorometric analysis. However, NBD-F exhibits distinct advantages over NBD-Cl in terms of stability, sensitivity, selectivity, and compatibility.19,22
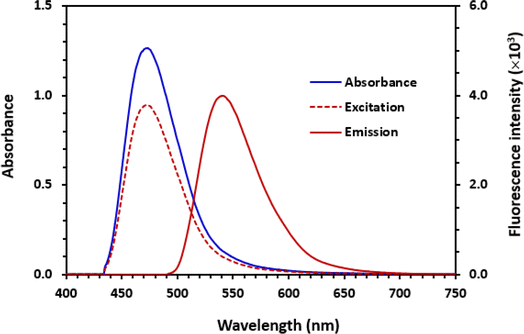 |
| Fig. 2 Absorption and fluorescence (excitation and emission) spectra of the reaction product of PLZ with NBD-F. The excitation wavelength for the emission spectrum was 473, and emission wavelength for the excitation spectrum was 541 nm. The concentrations of PLZ were 100 and 0.5 μg mL−1 for scanning the absorption and fluorescence spectra, respectively. | |
The reaction between NBD-F and PLZ, which contains amino groups, has not been previously reported. Hence, this study focuses on investigating this reaction and utilizing it to develop sensitive and straightforward photometric and fluorimetric assays for the determination of PLZ in bulk drug substance and pharmaceutical formulations, particularly injections. Considering the advantages of microwell-based analysis, as demonstrated in previous studies,14–16 this research aims to explore the utility of NBD-F as a dual-function probe in developing this methodology for PLZ. The aim is to form a colored and fluorescent PLZ-NBD derivative upon their reaction.
3.2. Spectral characteristics
In NBD-F, the fluorine atom is activated by the 7-nitro group, thus it can be easily substituted by nucleophilic groups such as amino groups to form the corresponding amino products. Accordingly, the reaction between NBD-F and PLZ was anticipated to proceed smoothly due to the presence of a labile fluorine atom in the chemical structure of NBD-F and the presence of amino groups in the chemical structure of PLZ. The spectra presented in Fig. 2 confirmed this reaction, as evidenced by the emergence of an orange-colored and yellow-fluorescent reaction product. Notably, distinct absorption and fluorescence emission peaks were observed in the absorption and fluorescence spectra of the reaction solution, further confirming the occurrence of the reaction. The absorption spectrum revealed a maximum absorption peak at 473 nm, while the fluorescence emission spectrum exhibited a peak at 541 nm when excited at 473 nm (Fig. 2). It is wise to mention that in most cases, it is indeed the emission wavelengths to be at longer wavelengths (lower energy) than the excitation wavelengths. This phenomenon, known as Stokes shift, occurs due to loss of some energy of the exciting light by various factors such as solvent effects, molecular environment, and energy transfer mechanisms.11 The stock shift (difference between emission and excitation wavelengths) in the fluorescence spectra of PLZ was 68 nm (541–473).
3.3. Optimization of reaction conditions
In the current study, NBD-F was specifically designed to serve as a dual-function chromogenic and fluorogenic probe. Therefore, the optimization of conditions was carried out, and both absorbances and fluorescence intensities were measured. In the subsequent sections, the results of absorbance measurements will be presented. Additionally, similar results were obtained when measuring the fluorescence intensities.
3.3.1. NBD-F concentration. The investigation into the effect of NBD-F concentration on its reaction with PLZ demonstrated a clear dependence of the reaction on the concentration of NBD-F. As depicted in Fig. 3A, the absorbance of the reaction solution increased with higher concentrations of NBD-F. The highest absorbance readings were obtained at an NBD-F concentration of 0.2% (w/v), beyond which no further increase in the signals was observed. Consequently, for subsequent experiments, a concentration of 0.2% (w/v) of NBD-F was selected as the optimal concentration.
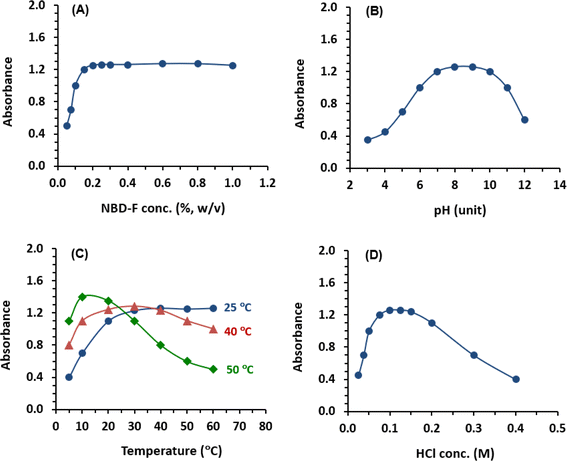 |
| Fig. 3 Effect of NBD-F concentration (A), pH of buffer solution (B), temperature and time (C), and HCl concentration (D) on the absorption intensity of the PLZ-NBD reaction product. The concentration of PLZ in all reactions was 100 μg mL−1. | |
3.3.2. Buffer solution and pH. To generate the necessary nucleophile from PLZ, the reaction must be conducted in an alkaline environment. Various alkaline buffer systems, including borate, phosphate, and carbonate buffers with a pH value of 8, were tested. Among these, the highest signal was achieved when the reaction was carried out in borate. However, when other buffers were utilized, issues such as the precipitation of a white colloid upon the addition of NBD-F reagent solution, non-reproducible results, or weak sensitivities were observed. Therefore, borate buffer solution was selected for the subsequent investigations. It is important to mention that the borate buffer solution is active near pH 9, and it buffering capacity decreases at lower and higher pH value. However, in the present study, the assay was performed very close to the optimum buffering action of borate buffer. Additionally, the volumes of buffer and sample solutions were similar (50 μL), avoiding the loss of buffer capacity that happens when the buffer solution is added to large volumes of samples.The effect of pH of borate buffer solution on the reactions was investigated using pH values ranging from 3 to 12. The results indicated that the signals increased as the pH increased, reaching maximum readings at pH range of 8–10 (Fig. 3B). This increase in signals with alkaline pH values can be attributed to the conversion of the amino group of PLZ from the acid salt form (at acidic pH values) to the free amino group as the pH becomes alkaline. This conversion facilitates the nucleophilic substitution reaction. However, readings sharply decreased at pH values above 10. This decline may be attributed to an increase in the concentration of hydroxide ions, which hinders the condensation reaction between PLZ and NBD-F. Accordingly, the subsequent experiments were carried out at pH 8 ± 0.5.
3.3.3. Temperature and time. To determine the optimal temperature and reaction time for the completion of the derivatization reaction, the reaction was initially conducted at room temperature (25 ± 2 °C), and the resulting signals were measured at different time intervals. However, it was observed that the reaction proceeded relatively slowly as it reached completion after 30 min (Fig. 3C). Consequently, further investigations were conducted at elevated temperatures (40 and 50 °C), with monitoring the induced signals for a duration of 60 min. Higher temperatures were not tested due to the limitations of the built-in temperature control in the reader, which could not provide temperatures exceeding 50 °C. Moreover, in order to streamline the assay procedures, we opted against using a separate thermostatically controlled water bath as an additional instrument to conduct the experiment.The results indicated that higher temperatures accelerated the reaction; however, they did not improve the signal values (Fig. 3C). The maximum signals were obtained when the reaction was carried out for 20 min at 40 °C and 10 min at 50 °C, respectively. It was observed that the signals remained stable for approximately 40 min at 40 °C, but they decreased significantly at 50 °C, and the values were not reproducible. This decline in the signals was likely due to the degradation of the reagent at high temperatures. Notably, these observations align with the findings previously reported by Aktas et al.23 Additionally, the imprecise readings might be attributed to inconsistent partial evaporation of methanol (used for NBD-F solution) from the wells during incubation at 50 °C. In order to develop assays with compromised features in terms of time, throughput, precision, and convenience, subsequent experiments were conducted at room temperature (25 ± 2 °C) for 30 min.
3.3.4. HCl concentration. In the aforementioned conditions, it was observed that there were significantly high levels of signal backgrounds. This occurrence was attributed to the hydrolysis process of NBD-F, which resulted in the formation of its hydroxy derivative called 4-hydroxy-7-nitrobenzo-2-oxa-1,3-diazole (NBD-OH).24 The signal background of NBD-OH was found to be reduced when the pH of the reaction medium was lowered to below one.22 Hence, prior to measuring the absorbance or fluorescence, it was necessary to acidify the reaction mixture in order to greatly decrease the background signals.25 Importantly, this acidification process did not affect the reaction product, thereby leading to an ultimate increase in sensitivity. The concentration of HCl needed for acidification was determined to be 0.1 M (Fig. 3D).
3.3.5. Stability of PLZ-NBD. The impact of time on the stability of the colored and fluorescent PLZ-NBD derivative was investigated by monitoring the absorbance and fluorescence signals of the reaction solution at various time intervals. It was observed that both the absorbance and fluorescence values remained unchanged for a minimum of 1 hour. This extended stability enabled the processing of substantial sample batches and facilitated convenient measurements. Consequently, this enhanced the convenience of the assay and made it suitable for analyzing a large number of samples.A concise overview of the variables affecting the reaction of PLZ with NBD-F is given in Table 1.
Table 1 A summary of optimization of conditions affecting the reaction of PLZ with NBD-F
Condition |
Studied range |
Optimum value |
Buffer solutions were: borate, phosphate, and carbonate. |
NBD-F concentration (%, w/v) |
0.05–1 |
0.2 |
Buffer type |
Differenta |
Borate |
Buffer pH |
3–12 |
8 ± 0.5 |
Temperature (°C) |
25–50 |
25 ± 2 |
Reaction time (min) |
5–60 |
30 |
HCl concentration (M) |
0.02–0.4 |
0.1 |
Stability of reaction product |
5–60 |
≥60 |
Absorbance wavelength (nm) |
400–750 |
473 |
Excitation wavelength (nm) |
400–750 |
473 |
Emission wavelength (nm) |
400–750 |
541 |
3.4. Reaction stoichiometry and pathway
The stoichiometry of the PLZ/NBD-F reaction was investigated using the limiting logarithmic method.18 The results are presented and discussed for absorbance measurements, while similar results were obtained for fluorescence measurements. A plot of log absorbance as a function of log[PLZ] at a constant concentration of NBD-F gave a straight line with a slope of 0.7633 (Fig. 4A). A plot of log absorbance as a function of log[NBD-F] at a constant concentration of PLZ gave a straight line with a slope of 0.7631 (Fig. 4B). Thus, the molar ratio of the reaction is 0.7633
:
0.7631 (i.e. molar ratio is 1.0003; 0.7633/0.7631). Based on this ratio, the proposed reaction pathway between PLZ and NBD-F is depicted in Fig. 5. It is wise noting that the PLZ molecule contains multiple potentially reactive amino groups; however, it was postulated that only one primary amino group of all groups contributed to the reaction with NBD-F, as illustrated in Fig. 5. It was also postulated that the other remaining primary or secondary amino groups did not react due to their unfavorable steric hindered positions in the PLZ's structure, or the mild reaction conditions (room temperature) employed in the study were not energetically adequate for their contribution in the reaction. The postulated reaction pathway (Fig. 5) was established based on key facts. It is important to note that to validate and support the hypothesis, further future experimental characterization of the reaction product would be required. In general, primary amines demonstrate higher reactivity compared to secondary amines in nucleophilic substitution reactions with NBD-F. This enhanced reactivity can be attributed to the fact that primary amines having only one alkyl group attached to the nitrogen atom, which facilitates a greater degree of nucleophilicity. Consequently, the lone pair of electrons on the nitrogen atom is more easily accessible for attacking the electrophilic carbon of the NBD-F molecule. The reactivity of different amino groups in the PLZ molecule during nucleophilic substitution reactions with NBD-F can also be influenced by various factors. These factors include steric hindrance, the presence of electron-donating or electron-withdrawing groups, and basicity. In contrast, secondary amino groups have nitrogen atoms attached to two alkyl groups, which impedes nucleophilic attack and diminishes reactivity. The steric hindrance imposed by the alkyl groups makes it more challenging for the nitrogen lone pair to effectively approach the electrophilic carbon of NBD-F.26
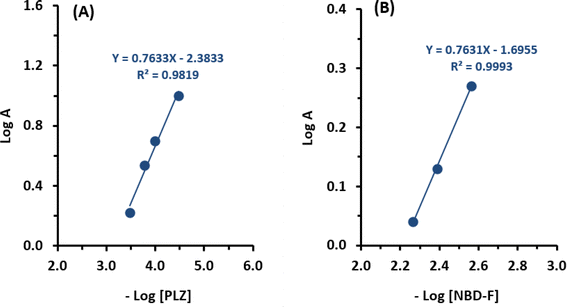 |
| Fig. 4 Limiting logarithmic plot for molar reactivity of PLZ with NBD-F. The first line (A) was generated using varying PLZ concentrations (3.37 × 10−5 to 1.69 × 10−4 M) and a fixed NBD-F concentration (1.09 × 10−2 M). The second line (B) was generated using varying NBD-F concentrations (2.37 × 10−1 to 8.19 × 10−1 M) and a fixed PLZ concentration (6.75 × 10−4 M). Log A, log[PLZ], and log[NBD-F] are the logarithm of absorbance, molar concentration of PLZ, and molar concentration of NBD-F, respectively. Linear fitting equations and their determination coefficients are given on the corresponding lines. | |
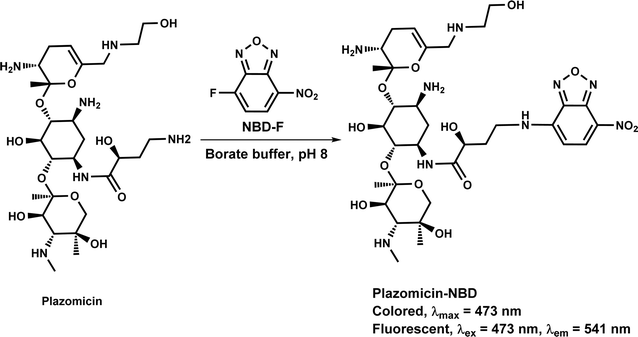 |
| Fig. 5 Scheme for the reaction pathway of PLZ with NBD-F. | |
3.5. Validation of MW-PM and MW-FL assays
3.5.1. Calibration and sensitivity. The calibration curves for the determination of PLZ through its reaction with NBD-F were constructed under the optimal conditions outlined in Table 1. The signals, namely absorbance for the MW-PM assay and relative fluorescence intensity (RFI) for the MW-FL assay, were plotted against the corresponding PLZ concentrations (Fig. 6). Regression analysis was performed on the dataset, resulting in the derivation of the following regression equations:
For MW-PM assay: Y = 0.0048 + 0.0031X (R2 = 0.9994) |
For MW-FL assay: Y = 0.0468 × 103 + 6121.7X (R2 = 0.9993) |
where Y is the absorbance or RFI (for MW-PM or MW-FL assay, respectively), X is the PLZ concentration in μg mL−1, and R2 is the determination coefficient.
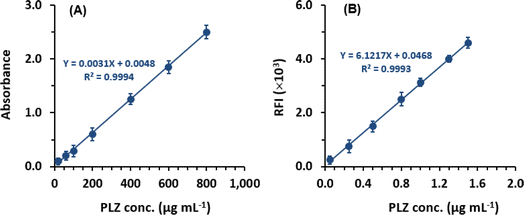 |
| Fig. 6 Calibration curves for the determination of PLZ by MW-PM (A) and MW-FL (B) assays via its reaction with NBD-F probe. RFI is the relative fluorescence intensity. Each point is the average of 5 replicate measurements, and the presented error bars are the standard deviations of the reading at 95% confidence. Linear fitting equations with their corresponding determination coefficients (R2) are given on the lines. | |
Linear relationships with small intercepts and determination coefficients (0.9994 and 0.0993 for MW-PM and MW-FL assays, respectively) were established within the concentrations ranges of 20 to 800 for MW-PM assay and 0.05 to 1.5 μg mL−1 for MW-FL assay (Table 2). The determination of the limit of detection (LOD) and limit of quantitation (LOQ) followed the guidelines of The International Council of Harmonization (ICH) for validating analytical procedures.27 For MW-PM, the LOD and LOQ values were determined to be 8.3 and 25 μg mL−1, respectively. For MW-FL, the LOD and LOQ values were 0.03 and 0.08 μg mL−1 (Table 2). The regression and performance parameters of the proposed MW-SP and MW-FL assays are summarized in Table 2.
Table 2 The regression and performance parameters for the determination of PLZ by the proposed MW-PM and MW-FL assays via reaction with NBD-F probe
Parameter |
Value |
MW-PM |
MW-FL |
Linear range (μg mL−1) |
20–800 |
0.05–1.5 |
Intercept |
0.0048 |
46.8 |
Standard deviation of intercept |
0.0078 |
49 |
Slope |
0.0013 |
6121.7 |
Standard deviation of slope |
0.0005 |
50.1 |
Determination coefficient (R2) |
0.9994 |
0.9993 |
Limit of detection, LOD (μg mL−1) |
8.3 |
0.03 |
Limit of quantitation, LOQ (μg mL−1) |
25 |
0.08 |
3.5.2. Precision and accuracy. To assess the precision of the proposed MW-PM and MW-FL assays, replicate samples of PLZ solutions with low, medium, and high concentration levels (Table 3) were analyzed by the proposed assays. The relative standard deviations (RSD) of the obtained results were calculated and used as measures for precision. Both intra-day and inter-day precision showed RSD values ranging from 1.2% to 1.8% for the MW-PM assay and from 0.9% to 1.6% for the MW-FL assay, indicating the high precision of both assays.
Table 3 The precision and accuracy of MW-PM and MW-FL assays for the determination of PLZ via its reaction with NBD-F probe
PLZ concentration (μg mL−1) |
Intra-day (n = 3) |
Inter-day (n = 6) |
Recovery (% ± RSD) |
Error (%) |
Recovery (% ± RSD) |
Error (%) |
MW-PM |
50 |
100.5 ± 1.8 |
0.5 |
101.4 ± 1.2 |
1.4 |
400 |
99.5 ± 1.2 |
−0.5 |
98.9 ± 1.6 |
−1.1 |
750 |
101.2 ± 1.4 |
1.2 |
100.8 ± 1.5 |
0.8 |
![[thin space (1/6-em)]](https://www.rsc.org/images/entities/char_2009.gif) |
MW-FL |
0.2 |
100.4 ± 1.2 |
0.4 |
101.6 ± 1.4 |
1.6 |
0.8 |
99.8 ± 1.5 |
−0.2 |
99.1 ± 1.6 |
−0.9 |
1.2 |
101.2 ± 0.9 |
1.2 |
100.5 ± 1.2 |
0.5 |
To assess the accuracy of the MW-PM and MW-FL assays, recovery studies were conducted using the same PLZ samples. As shown in Table 3, the recovery values ranged from 98.9% to 101.4% and 99.1% to 101.6% for MW-PM and MW-FL assays, respectively. Also, the errors ranged from −0.4% to 1.2% and −1.1 to 1.6% for MW-PM and MW-FL, respectively. These results demonstrate the high accuracy of both assays.
The high precision and accuracy achieved by the proposed MW-PM and MW-FL assays can be attributed to several key factors:
(1) Consistent sample handling: the use of microwell plates ensures reproducible samples and reagents (NBD-F, buffer, and HCl) handling. Each well is designed with uniform volume, shape, and dimensions, promoting standardized sample preparation. This consistency reduces variability in sample/reagents dispensing, leading to improved measurement precision.
(2) Parallel processing: the 96-well assay plates enable simultaneous dispensing of multiple samples and conducting reactions. This parallel processing capability minimizes the impact of random errors and enhances the statistical significance of the results. It also mitigates variations caused by sequential sample handling, which can introduce errors and affect precision.
(3) Small sample volumes: the MW-PM and MW-FL assays utilize small sample and reagent volumes (50 μL each). This approach reduces the influence of pipetting errors and enhances both precision and accuracy in measurements.
(4) Controlled environmental conditions: the procedures of both assays are performed under controlled room temperature conditions (25 ± 2 °C), effectively minimizing the effects of temperature fluctuations on samples and reactions. Temperature stability helps prevent errors and maintains the accuracy of measurements. By ensuring controlled conditions, the reproducibility and reliability of the results are enhanced.
(5) Optical pathlength: the optical pathlength is an important parameter in spectroscopic measurements of solutions in microwell assay plates by multi-mode reader as it affects the sensitivity and accuracy of both absorbance and fluorescence measurements. In multi-mode microwell plates, the optical pathlength can vary based on the design of the plate and the volume of the sample. In the present assays involving 200 μL as a total volume of sample solution, the optical pathlength is 2 mm.
3.5.3. Robustness and ruggedness. The robustness of both the MW-PM and MW-FL assays was evaluated to assess the impact of minor variations in assay variables on their analytical performance. These variables, such as the concentration of NBD-F probe, as well as pH, reaction time, and concentration of HCl, were intentionally deviated from their optimal values, as summarized in Table 4. Notably, it was observed that these deviations had no significant effects on the results of both MW-PM and MW-FL assays. The recovery values obtained ranged from 98.6% to 101.5% for MW-PM assay and from 99.2% to 101.6% for MW-FL assay. The precision of both assays, expressed as RSD values, ranged from 1.2% to 1.8% for MW-PM assay and from 0.8% to 1.6% for MW-FL assay. These findings confirm the suitability of both assays for routine analysis of PLZ.
Table 4 The robustness and ruggedness of the proposed MW-PM and MW-FL assays for the determination of PLZ via reaction with NBD-F probe
Parameters |
Recovery (% ± RSD)a |
MW-PM |
MW-FL |
The values are means of three determinations. |
Robustness |
NBD-F concentration (%, w/v) |
0.15 |
101.2 ± 1.5 |
100.6 ± 1.2 |
0.25 |
101.5 ± 1.4 |
101.6 ± 1.6 |
pH (unit) |
7.8 |
98.6 ± 1.8 |
101.4 ± 1.5 |
8.2 |
101.4 ± 1.2 |
99.5 ± 1.2 |
Reaction time (min) |
25 |
99.4 ± 1.6 |
101.6 ± 1.4 |
35 |
100.3 ± 1.2 |
99.2 ± 0.8 |
HCl concentration (M) |
0.8 |
99.4 ± 1.5 |
100.1 ± 1.2 |
1.2 |
101.2 ± 1.4 |
99.4 ± 1.4 |
![[thin space (1/6-em)]](https://www.rsc.org/images/entities/char_2009.gif) |
Ruggedness |
Analyst to-analyst |
Analyst-1 |
99.6 ± 1.2 |
100.8 ± 1.1 |
Analyst-2 |
101.6 ± 1.5 |
101.2 ± 1.2 |
Day-to-day |
Day-1 |
99.2 ± 1.8 |
99.5 ± 1.4 |
Day-2 |
100.4 ± 1.2 |
100.6 ± 1.5 |
Day-3 |
101.2 ± 1.3 |
98.4 ± 1.7 |
Furthermore, the ruggedness of the assays was assessed by involving two different analysts to perform the procedures on three different days. The results obtained from the day-to-day variations demonstrated reproducibility, with RSD values not exceeding 1.8% for both assays. This further underscores the reliability and consistency of both assays, even when conducted by different analysts on different occasions.
3.5.4. Selectivity and interference. According to the prescribing information for Zemdri® injection,4 sodium hydroxide is the only additive present in the formulation. Importantly, sodium hydroxide does not cause interference in the determination of PLZ within the injection formulation. The absence of potential interference can be attributed to two main reasons. Firstly, the reaction involved in both assays is selective for molecules containing an amino group, which is present in PLZ but not in the additive. Secondly, the absorbance measurements in the MW-PM assay and fluorescence measurements in the MW-FL assay are selective, as the colored and fluorescent PLZ-NBD derivative specifically absorbs light and emits fluorescence at certain wavelengths. Therefore, any potential interferences from the injection additive are avoided due to the specific characteristics of the PLZ derivative. It is important to state that the proposed assays are not appropriate for analysis of biological samples because biological interferences have not been accounted for here.
3.6. Analysis of PLZ drug substance and Zemdri® injection
The validated MW-PM and MW-FL assays were utilised to quantify PLZ in its drug substance and Zemdri® injection at definite predetermined concentrations within the specific linear range of each assay. The determined recovery percentage are presented in Table 5. The recovery values obtained ranged from 98.3 to 102.1%, for PLZ drug substance, and from 98.6 to 102.3% for Zemdri® injection. Additionally, the RSD values did not exceed 1.8% for both assays. These high recovery percentages and low RSD values confirm the accuracy and precision of the proposed assays for determining PLZ concentration in its drug substance and Zemdri® injection.
Table 5 Analysis of PLZ in its drug substance and Zemdri® injection by the proposed MW-PM and MW-FL via reaction with NBD-F probe
Analysed PLZ concentration (μg mL−1) |
Recovery (% ± RSD)a |
PLZ drug substance |
Zemdri® injection |
Values are mean of three determinations. |
MW-PM |
50 |
100.2 ± 1.1 |
99.4 ± 1.2 |
400 |
98.3 ± 1.7 |
99.3 ± 1.8 |
750 |
101.2 ± 1.2 |
101.1 ± 1.3 |
![[thin space (1/6-em)]](https://www.rsc.org/images/entities/char_2009.gif) |
MW-PM |
0.2 |
98.6 ± 1.8 |
98.6 ± 1.1 |
0.8 |
100.2 ± 1.2 |
102.3 ± 1.6 |
1.2 |
102.1 ± 1.6 |
101.1 ± 1.8 |
3.7. Eco-friendliness/greenness of MW-PM and MW-FL assays
Microwell-based assays supported by microplate readers align with the principles of GAC due to their miniaturized analytical processes. This approach enables the use of smaller volumes of samples and reagents, resulting in reduced waste generation compared to traditional techniques. To evaluate the eco-friendliness or greenness of the proposed MW-PM and MW-FL assays, three comprehensive metric tools were employed: Analytical Eco-Scale (AES),28 Green Analytical Process Index (GAPI),29 and Analytical Greenness Evaluation (AGREE).30 The instructions and guidelines of using these tools are outlined in their corresponding reports.28–30 Since both MW-PM and MW-FL assays involve the same reagents and operating conditions, both asasys were evaluated in a similar manner.
The results obtained from AES are presented in Table 6. With 50 μL of methanolic solution of NBD-F and 50 μL of HCl solution, a total of 4 penalty points (PPs) were assigned. The hazardous impact of methanol and the other reagents contributed to a subtotal of 9 PPs. Parameters related to energy consumption by the microplate reader and occupational hazards did not receive any PPs, as they complied with GAC guidelines. Waste production and treatment parameters received subtotals of 1 and 3 PPs, respectively. These scores were assigned because the assay generated less than 1 mL of waste per sample, and the waste was not treated. The total PPs for the assay amounted to 13, resulting in an Eco-scale score of 87 (100 − 13). This high score, exceeding 75, indicates that the proposed microwell-based analytical methods demonstrate an excellent level of environmental friendliness.
Table 6 Analytical Eco-Scale for assessing the eco-friendliness of the proposed MW-PM and MW-FL assays for the determination of PLZ via reaction with NBD-F probe
Eco-scale score parameters |
Penalty points (PPs) |
Amount of solvent/reagent |
Solvent, methanol: <1 mL (mL (g) per sample) |
1 |
Reagent, NBD-F: <1 mL (mL (g) per sample) |
1 |
HCl (0.1 M): <1 mL (mL (g) per sample) |
2 |
|
∑ = 4 |
![[thin space (1/6-em)]](https://www.rsc.org/images/entities/char_2009.gif) |
Hazard of solvent/reagent |
Solvent: methanol |
3 |
Reagent: NBD-F |
3 |
HCl |
3 |
|
∑ = 9 |
![[thin space (1/6-em)]](https://www.rsc.org/images/entities/char_2009.gif) |
Instrument: energy used (kW h per sample) |
Microplate reader |
0 |
|
∑ = 0 |
![[thin space (1/6-em)]](https://www.rsc.org/images/entities/char_2009.gif) |
Occupational hazardous |
|
Analytical process hermetic |
0 |
Emission of vapors and gases to the air |
0 |
|
∑ = 0 |
![[thin space (1/6-em)]](https://www.rsc.org/images/entities/char_2009.gif) |
Waste |
Production (<1 mL (g) per sample) |
1 |
Treatment (no treatment involved) |
3 |
|
∑ = 4 |
Total PPs |
13 |
Eco-scale score |
87 |
Fig. 7 presents a pictogram of the results obtained from the GAPI tool, which assesses 15 parameters. Two parameters (1, and 15) are depicted in red. Parameter 1 signifies that samples were collected or prepared offline, and parameter 15 reflects that the assays' waste was not treated. Parameters 5 and 6 are depicted in yellow, indicating that the assays are suitable for quantitative analysis and the samples were extracted on a microscale, respectively. The remaining parameters are shown in green, indicating that they meet the requirements of green procedures according to the tool's guidelines.29
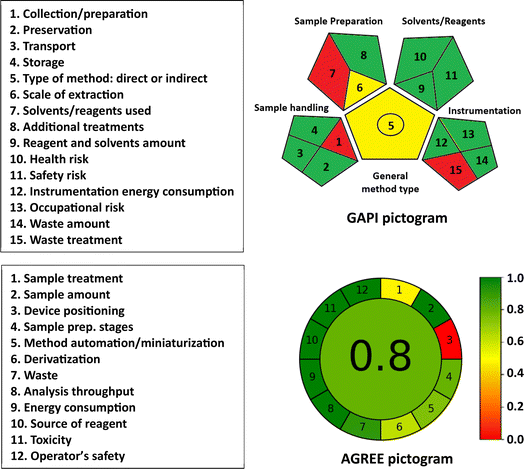 |
| Fig. 7 Results of GAPI and AGREE metric tools for assessment of the greenness of the proposed MW-PM and MW-FL methods for the determination of PLZ. | |
The AGREE pictogram, displayed in Fig. 7, illustrates the assessment results. Parameter 1, related to sample treatment, is marked with yellow due to the manual execution of sample treatment. Parameter 3, which concerns device positioning (online or offline) is depicted in red because the analysis was performed offline using a plate reader. The remaining parameters are assigned a green color. The total score obtained is 0.8 out of 1, indicating a high level of environmental friendliness for the assays.
In conclusion, the results obtained from the three assessment tools provide conclusive evidence of the eco-friendliness of the proposed MW-PM and MW-FL assays for PLZ and their alignment with the principles of GAC.
3.8. High throughput of MW-PM and MW-FL assays
Microwell-based techniques have garnered significant recognition in the pharmaceutical analysis field due to their capacity to simultaneously analyze and manipulate multiple individual entities with high efficiency. The throughput of the proposed MW-PM and MW-FL was assessed based on employing 96-well plates and a specific reaction time of 30 min for both assays. An analyst can comfortably process a minimum of 6 plates as a batch simultaneously. Under these conditions, a total of 1152 samples could be processed per hour (6 plates × 96 wells × 2 rounds per hour), highlighting the exceptional throughput of the MW-PM and MW-FL assays. Furthermore, there are additional approaches that can further enhance the throughput of the assays. One such approach involves utilizing plates with a higher well count, such as 384, 1536, or 3456 wells, which would allow for the simultaneous processing of even more samples. Another approach involves automating the process through the utilization of automated systems or robots. Implementing these strategies can significantly contribute to achieving an even higher throughput, enabling the analysis of a larger number of samples within a given timeframe.
4. Conclusion
In this study, the reactivity of PLZ with the NBD-F probe was investigated and found that the reaction proceeds smoothly under mild conditions. The resulting product exhibited both chromogenic and fluorogenic properties, displaying light absorption at 473 nm and fluorescence emission at 541 nm when excited at 473 nm. The reaction stoichiometry was determined, and the reaction pathway was proposed. The dual chromogenic and fluorogenic nature of the NBD-F probe was utilized in the developed photometric and fluorometric assays for PLZ using a microwell-based analytical approach. These assays, named MW-PM and MW-FL, represent the first microwell-spectrometric methodology for PLZ analysis. They were successfully applied to determine PLZ levels in bulk drug substance and pharmaceutical formulation (injection) with high reliability. Notable features of these assays include their high throughput capability, enabling the analysis of a large sample size within a short timeframe. Additionally, these assays are environmentally friendly in pharmaceutical manufacturing laboratories, as they require minimal amounts of samples and reagents. A total description of the improvement of the proposed MW-PM and MW-FL over methodologies that are reported in the literature for PLZ is given in Table 1S.†
Abbreviations
PLZ | Plazomicin |
FDA | The US Food and Drug Administration |
NBD-F | 4-Fluoro-7-nitrobenzofurazan |
GAC | Green analytical chemistry |
MW-PM | Microwell photometry |
MW-FL | Microwell fluorometry |
RFI | Relative fluorescence intensity |
ICH | The international Council of Harmonization |
LOD | Limit of detection |
LOQ | Limit of quantitation |
RSD | Relative standard deviation |
AES | Analytical Eco-scale |
PPs | Penalty points |
GAPI | Green analytical procedure index |
AGREE | Analytical greenness |
Data availability
The data supporting the current study are available from the corresponding author [I. A. D.] upon request.
Conflicts of interest
The authors report no conflicts of interest for this work.
Acknowledgements
The authors extend their appreciation to the Researchers Supporting Project Number (RSPD2024R944), King Saud University, Riyadh, Saudi Arabia, for funding this work.
References
- J. A. Clark and D. S. Burgess, Plazomicin: a new aminoglycoside in the fight against antimicrobial resistance, Ther. Adv. Infect. Dis., 2020, 7, 1–15 Search PubMed.
- A. Bilinskaya, K. E. Linder and J. L. Kuti, Plazomicin: an intravenous aminoglycoside antibacterial for the treatment of complicated urinary tract infections, Expert Rev. Anti-Infect. Ther., 2020, 18, 705–720 CrossRef CAS.
- M. W. McCarthy, Plazomicin for the treatment of patients with complicated urinary tract infection, Drugs Today, 2018, 54, 513–518 CrossRef CAS.
- FDA, ZEMDRI™ Prescribing Information, available from: https://www.accessdata.fda.gov/drugsatfda_docs/label/2018/210303orig1s000lbl.pdf, accessed on June 29, 2024.
- A. Alfieri, S. Di Franco, V. Donatiello, V. Maffei, C. Fittipaldi, M. Fiore, F. Coppolino, P. Sansone, M. C. Pace and M. B. Passavanti, Plazomicin against multidrug-resistant bacteria: a scoping review, Life, 2022, 12, 1949 CrossRef CAS.
- A. Eldin, O. Ismaiel, W. Hassan and A. Shalaby, Green analytical chemistry: Opportunities for pharmaceutical quality control, J. Anal. Chem., 2016, 71, 861–871 CrossRef CAS.
- P. G. Wang, High-throughput Analysis in the Pharmaceutical Industry, CRC Press, New York, 2008 Search PubMed.
- F. Farouk, H. M. E. Azzazy and W. M. A. Niessen, Challenges in the determination of aminoglycoside antibiotics, a review, Anal. Chim. Acta, 2015, 890, 21–43 CrossRef CAS.
- R. Hari, S. Taherunnisa, S. Y. Raut, S. Mutalik and K. B. Koteshwara, Challenges in the development of analytical test procedure for aminoglycosides: A critical review, J. Appl. Pharm. Sci., 2019, 9, 145–152 CrossRef CAS.
- S. Görög, Ultraviolet-visible Spectrophotometry in Pharmaceutical Analysis, CRC Press, New York, NY, USA, 2018 Search PubMed.
- A. Bose, I. Thomas, G. Kavitha and E. Abraham, Fluorescence spectroscopy and its applications: a review, Int. J. Adv. Pharm. Anal., 2018, 8, 1–8 CrossRef CAS.
- G. Liu and J.-M. Lin, Microplate-based assays: The future of pharmaceutical analysis, Trends Anal. Chem., 2016, 85, 43–48 Search PubMed.
- P. Singh and B. Singh, Microwell spectrophotometry: A green analytical technique for pharmaceutical analysis, J. Pharm. Anal., 2017, 7, 203–208 CrossRef.
- M. S. Alsalhi, M. Al-Outaibi and I. A. Darwish, Development of three innovative green microwell spectrometric methods with high throughput for determination of seleciclib in its bulk form and capsules, Sustainable Chem. Pharm., 2024, 37, 101443 CrossRef CAS.
- I. A. Darwish and N. Z. Alzoman, Development and validation of green and high-throughput microwell spectrophotometric assay for the determination of selective serotonin reuptake inhibitors in their pharmaceutical dosage forms, Molecules, 2023, 28, 4221 CrossRef CAS PubMed.
- I. A. Darwish and N. Z. Alzoman, Development of green and high throughput microplate reader-assisted universal microwell spectrophotometric assay for direct determination of tyrosine kinase inhibitors in their pharmaceutical formulations irrespective the diversity of their chemical structures, Molecules, 2023, 28, 4049 CrossRef CAS PubMed.
- M. Pesez and J. Bartos, Colorimetric and Spectrofluorimetric Analysis of Organic Compounds and Drugs, Marcel Dekker Inc., New York, 1974, pp. 628–630 Search PubMed.
- I. A. Darwish, H. H. Abdine, S. M. Amer and L. I. Al-Rayes, Simple spectrophotometric method for determination of paroxetine in tablets using 1,2-naphthoquinone-4-sulphonate as a chromogenic reagent, Int. J. Anal. Chem., 2009, 2009, 237601 CrossRef.
- H. Raissi, I. Chérif, H. Ayachi, A. H. Said, F. Hassen, S. Ayachi and T. Boubaker, Structure-Property relationships in benzofurazan derivatives: A combined experimental and DFT/TD-DFT investigation, in Density Functional Theory: Recent Advances, New Perspectives and Applications, ed. D. Glossman-Mitni, IntechOpen, London, 2021 Search PubMed.
- T. Santa, Recent advances in development and application of derivatization reagents having a benzofurazan structure: a brief overview, Biomed. Chromatogr., 2014, 28, 760–766 CrossRef CAS PubMed.
- B. I. Salman, M. F. B. Ali, M.
A. Marzouq and S. A. Hussein, Utility of the fluorogenic characters of benzofurazan for analysis of tigecycline using spectrometric technique; application to pharmacokinetic study, urine and pharmaceutical formulations, Luminescence, 2019, 34, 175–182 CrossRef CAS.
- A. O. Alnajjar, A. A. Elbashir, R. E. Elgorashe, A. M. Ebrahim, A. M. Idris and H. M. Abd El-Lateef, Utilization of 4-fluoro-7-nitro-2,1,3-benzoxadiazole (NBD-F) as a fluorogenic reagent for the development of a spectrofluorometric assay method for taurine in energy drinks, J. Chem. Res., 2022, 46, 1–7 Search PubMed.
- E. S. Aktas, L. Ersoy and O. Sagirh, A new spectrofluorimetric method for the determination of lisinopril in tablets, Farmaco, 2003, 58, 165–168 CrossRef CAS PubMed.
- H. Miyano, T. Toyo’oka and K. Imai, Further studies on the reaction of amines and proteins with 4-fluoro-7-nitrobenzo-2-oxa-1,3-diazole, Anal. Chim. Acta, 1985, 170, 81–87 CrossRef CAS.
- K. Imai, T. Toyo Oka and H. Miyano, Fluorigenic reagents for primary and secondary amines and thiols in high-performance liquid chromatography. A review, Analyst, 1984, 109, 1365–1373 RSC.
- A. A. Elbashir, F. E. O. Suliman and H. Y. Aboul-enein, The application of 7-chloro-4-nitrobenzoxadiazole and 4-fluoro-7-nitro-2,1,3-benzoxadiazole for the analysis of amines and amino acids using high-performance liquid chromatography, Gazi Univ. J. Sci., 2011, 24, 679–697 Search PubMed.
- ICH, International Council for Harmonisation of Technical Requirements for Pharmaceuticals for Human Use; ICH Harmonised Guideline; Validation of Analytical Procedure: Q2(R2), ICH, Geneva, Switzerland, 2022 Search PubMed.
- A. Gałuszka, P. Konieczka, Z. M. Migaszewski and J. Namieśnik, Analytical eco-scale for assessing the greenness of analytical procedures, Trends Anal. Chem., 2012, 37, 61–72 CrossRef.
- J. Płotka-Wasylka, A new tool for the evaluation of the analytical procedure: Green Analytical Procedure Index, Talanta, 2018, 181, 204–209 CrossRef.
- F. Pena-Pereira, W. Wojnowski and M. Tobiszewski, AGREE-analytical greenness metric approach and software, Anal. Chem., 2020, 92, 10076–10082 CrossRef CAS.
|
This journal is © The Royal Society of Chemistry 2024 |
Click here to see how this site uses Cookies. View our privacy policy here.