DOI:
10.1039/D4RA05244G
(Review Article)
RSC Adv., 2024,
14, 33384-33417
Recent updates on potential of VEGFR-2 small-molecule inhibitors as anticancer agents
Received
19th July 2024
, Accepted 4th October 2024
First published on 22nd October 2024
Abstract
The vascular endothelial growth factor receptor (VEGFR) system is the key component for controlling angiogenesis in cancer cells. Blocking vascular endothelial growth factor receptor 2 (VEGFR2) signalling is one of the most promising approaches to hindering angiogenesis and the subsequent growth of cancer cells. The USFDA-approved small-molecule drugs targeting VEGFR-2 are developing drug resistance over the course of chemotherapy, and cardiac-related side effects are consistently being reported; hence, there is an urgent need for more safe and effective anticancer molecules. The present review focuses on the structure and physiology of VEGFR-2 and its involvement in the progression of cancer cells. The recent updates from the last five years through papers and patents on structure–activity relationships, pharmacophoric attributes, molecular docking interactions, antiangiogenic assays, cancer cell line studies, and the potencies (IC50) of VEGFR-2 inhibitors are discussed herein. The common structural framework requirements, such as the Asp-Phe-Gly (DFG) motif of VEGFR-2 interacting with the HBD–HBA region in the ligand molecules, the central aryl ring occupying the linker region, and a variety of bio-isosteres, can enhance activity against VEGFR-2. At one end, the heteroaryl moiety is essential for interaction within the ATP-binding site of VEGFR-2, while the terminal hydrophobic tail occupies the allosteric binding site. Three to five bond spacers between the heteroaryl and HBD–HBA regions provided a better result towards VEGFR-2 inhibition, mirroring the behaviors of standard drugs. The in-depth analysis of recent updates on VEGFR-2 inhibitors presented in this paper will help prospective synthetic and medicinal chemists to discover new lead molecules for the treatment of various cancers.
1. Introduction
Cancer killed over 10 million people worldwide in 2020, or one in six.1 By 2040, there will be an additional 29.5 million new cases of cancer, according to the world health organization (WHO).2 The administration of antibodies and small-molecules for cancer chemotherapy are currently the two main strategies used in targeted cancer treatment. Despite their often-high selectivity, antibodies are limited in their ability to reach deep tissues because of their large molecular weight.3 Small-molecule inhibitors have significantly transformed the field of drug design and development in recent decades. This is because they possess the capability to effectively bind to a wider range of targets, both inside and outside of cells.4
Clinical investigation and drug resistance studies show that single targeting may not always have the desired biological effect, even when the target is inactivated or inhibited. Targeting a single oncoprotein may not generate long-term remission, hence studying biological networks for cancer is essential.5 Two prominent approaches are utilized for developing multi-targeting therapies. First, combining drugs aimed at different targets for an additive or synergistic effect.6 The second strategy is to develop a single drug with multiple targets to effectively block the numerous carcinogenic pathways.7 The search for a single agent that can act on two or more targets at the same time is a crucial aspect of the process of developing multi-targeting therapies.
1.1 Angiogenesis inhibition: a promising approach in anticancer therapy
Angiogenesis is the process by which primary solid tumours generate new blood capillaries to feed nutrition and oxygen, remove metabolic waste, and accelerate metastasis.8 John Hunter, a renowned British surgeon from the 18th century, gained widespread recognition for coining the term 'angiogenesis' in his writings.9 Angiogenesis, the physiological mechanism through which new blood vessels are generated from existing ones, plays a vital role in female reproductive health, cellular proliferation, wound healing, and tissue regeneration.10 The regulation of angiogenesis has a significant role in the development of several disorders, such as cancer, atherosclerosis, diabetes, and rheumatoid arthritis.11 As newly formed blood vessels offer oxygen and vital nutrients, they enable tumour growth and subsequently aid in the onset of metastasis, which leads to mortality in several cancers.12
Growth factors (GFs) are protein- or steroid-based hormones that stimulate growth, proliferation, and tissue regeneration.13 They attract smooth muscle cells and fibroblasts, which aid in the formation of blood vessels during angiogenesis. GFs also promote the growth and differentiation of endothelial cells. Collectively, these processes are known as sprouting and splitting.14 Several angiogenic GFs and their receptors have been discovered so far, with the most notable ones being angiopoietins (ANG), PDGF/R, basic fibroblast growth factor (bFGF/R, FGF/R-2), VEGF/R, TGF/R, insulin-like growth factor/receptor, and EGF/R.15,16 Antiangiogenic drugs for cancer treatment primarily target the inhibition of these factor's receptors.17 Fig. 1 illustrates the chronological sequence of identifying antiangiogenic factors that are crucial targets for fighting against various forms of carcinoma.
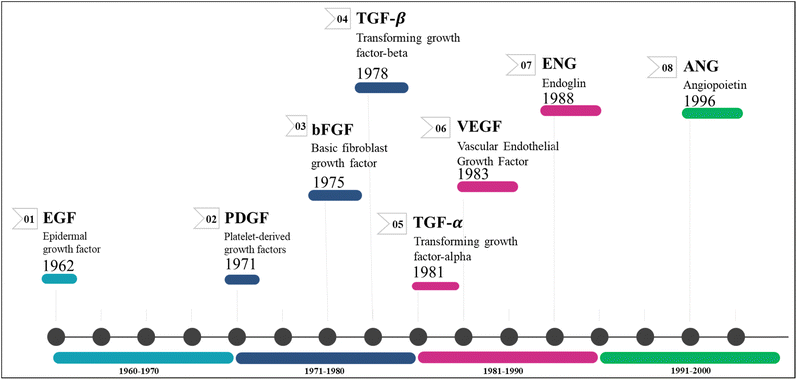 |
| Fig. 1 Discovery timeline of various angiogenic targets. | |
The inhibition of angiogenesis has become a viable approach for cancer treatment with the identification of new genes, transcription factors, signalling pathways, and mechanisms linked to tumour angiogenesis.18,19 Tumour blood vessels have higher vascular permeability and a better angiogenic potential than normal blood vessels. VEGF is a key component in controlling angiogenesis. As cancer progresses, VEGF has been found to be widely distributed and overexpressed.20
1.2 Involvement of VEGF and VEGFRs in angiogenesis
The goal of anti-angiogenic therapy is to limit the blood flow to tumour tissue by delivering anti-angiogenic drugs to reduce tumour growth and metastasis.21 The VEGF protein was first identified in 1989, and its role in angiogenesis was discovered.22,23 Having a weight of 40–45 kD, VEGF is a dimeric protein rich in cysteine that is highly conserved in mammals. It was discovered that VEGF improves the permeability of tumour blood vessels and induces ascites development.24 VEGFR-2 is a member of the family of receptor tyrosine kinases (RTKs). When VEGF binds to the protein kinase VEGFR-2, it triggers the production of blood capillaries and mediates the signalling pathway.25 Proangiogenic signalling molecules like VEGF and its cognate receptor, VEGFR-2, are essential for angiogenesis and are overexpressed in a lot of cancers.26
Blocking VEGFR-2 signalling is therefore seen as one of the most promising ways to prevent tumour-induced angiogenesis.27,28 Early antiangiogenic clinical trials focused on VEGF/VEGFR signalling blockade.29 The VEGF family is part of a broader category of signalling proteins known as cytokines. The subgroup consists of five proteins, namely VEGF-A, VEGF-B, VEGF-C, VEGF-D, and PlGF (placental growth factor), which have a significant impact on the processes of angiogenesis and lymphangiogenesis.30 The most particular of them, VEGFA, was discovered initially and is known to induce angiogenesis.31 The tyrosine kinase enzyme in the intracellular receptor domain is activated when VEGF binds to the extracellular domain. This leads to the phosphorylation of tyrosine and the activation of specific intracellular signalling pathways.32 VEGFR-1, VEGFR-2, and VEGFR-3 are the three extensively acknowledged receptors for the various members of the VEGF family.33 Both VEGFR-1 (Flt-1) and VEGFR-2 (KDR) are mainly expressed by vascular endothelial cells and hematopoietic stem cells, while VEGFR-3 is predominantly located on lymphatic endothelial cells.34 Each of these can incorporate specific components related to the VEGF family due to their unique affinity and selectivity. Although there are numerous VEGF/R-related factors that can cause pathological angiogenesis, the primary initiators of lymphangiogenesis are VEGF-C, VEGF-D, and the VEGFR-3 receptor.35
Endothelial cells are the primary source of VEGF-A secretion; however, other cell types such as astrocytes, macrophages, dendrocytes, thrombocytes, osteoblasts, lymphocytes, and tumour cells can also release it.36 It promotes the recruitment of inflammatory cells, such as macrophages and granulocytes, increases the permeability of blood vessels, prevents cell death, and encourages cell growth.37 VEGF-B's contribution to angiogenesis is rather minor. VEGF-B has a crucial role in promoting the survival of smooth muscle cells, neurons, pericytes, cardiac cells, and vascular endothelial cells in normal conditions.38 VEGF-C and VEGF-D emerge from the proteolytic cleavage of their precursors. When lymphatic vessels form during embryonic development, VEGF-C expression is high in the heart, thyroid, ovary, placenta, and gut as an adult.37 Lymphangiogenesis is stimulated by VEGFR-3 receptors, whereas angiogenesis is less affected. Recent discoveries show that it also binds to the NP-2 receptor, which boosts VEGFR-2 function.39 The extracellular portion of the VEGF receptors has seven motifs identical to immunoglobulin, while the intracellular portion contains a tyrosine kinase domain. VEGFR-1 expression in monocytes and macrophages has been demonstrated. It is known that VEGFR-3 is expressed by lymphatic endothelial cells.40 The role of VEGFR-3 in embryonic and pathological lymphangiogenesis and its affinity for VEGF-D and VEGF-C are well recognized. Signal pathways like PKC, Ras, and Akt/GDP are responsible for VEGFR-3 activation. Active VEGFR-3 promotes lymphatic endothelial cell proliferation, migration, differentiation, and survival.41
2. Structure of VEGFR-2
VEGFR-2 is a 200–230 kDa molecular weight receptor known as FLK-1 as well as KDR.42 VEGFR-2 is widely acknowledged as the most significant among all three VEGFRs,43 has been extensively studied, and its presence has been firmly confirmed in various types of cancer.44 When VEGF attaches to the extracellular domain, it triggers the activation of tyrosine kinase in the intracellular domain 45. VEGF's interaction with VEGFR-2 triggers the activation of the PLC/PKC, Ras/Raf/ERK MAPK, and PI3K/Akt pathways. These pathways play a crucial role in regulating both normal and abnormal blood vessel growth.46,47 There are 18 N-linked glycosylation sites, 15 phosphorylation sites, and numerous ATP-binding sites and substrate binding sites in human VEGFR-2, which play important roles in VEGFR-2 post-translational modifications, protein folding, protein activation, and cellular attachment and can further regulate the VEGFR-2 function.48,49 Human VEGFR-2 encodes 1356 amino acids of the full-length receptor, and the corresponding gene for it is situated at chromosome locus 4q.50 It responds better to VEGF-A than it does to VEGF-D or VEGF-C. Tyrosine kinase activity is higher in VEGFR-2 than in VEGFR-1. VEGFR-2 has a tyrosine kinase domain, an external ligand-binding domain, and a transmembrane domain. The goal of activating the receptor is to boost angiogenesis and vascular permeability.51 VEGFR-2 levels grow during the development of embryonic blood vessels, angiogenesis, and tumour angiogenesis.52 Fig. 2 shows the structure of VEGFR-2. The main places where phosphorylation happens are Y951, Y1054, Y1059, Y1175, and Y1214.53 Following VEGF binding, the primary auto-phosphorylation sites in human VEGFR-2, Y1175 and Y1214, activate various downstream pathways, including PI3K, p38MAPK, FAK, Src, and Akt, which are usually hyperactivated in several tumours.54 The analysis of the binding site reveals that the VEGFR-2 active site consists of four main components: the hinge area, two hydrophobic areas (hydrophobic-I and hydrophobic-II), and the hydrogen bond-rich region.55,56
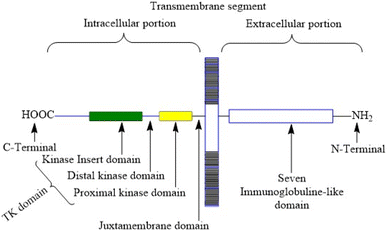 |
| Fig. 2 Structure of vascular endothelial growth factor receptor-2. | |
2.1 Pathophysiological role of VEGFR-2 in tumour
The VEGF/VEGFR-2 system is greatly involved in abnormal blood vessel formation, seen in conditions like muscular degeneration, diabetic retinopathy, inflammation, and cancer growth.57 De-regulation of VEGF/VEGFR-2 implicates directly in various diseases, and dysfunctional VEGFR-2 can cause developmental disorders of the vascular system and hematopoietic system during embryonic development.58 The VEGF/VEGFR-2 system is an important regulator of abnormal angiogenesis in cancer and healthy vasculogenesis in the early embryonic and adult stages.59 During the early stages of embryonic life, specifically at day 7.5 of gestation, certain cells known as mesodermic hemangioblasts exhibit the expression of VEGFR-2.60 The VEGFR-2 expression affects the migration and differentiation of these cells into endothelial cells. Additionally, it also contributes to the formation of vascular islands in the yolk sac, which marks the initiation of vasculogenesis.61 Vasculogenesis is the initial stage of embryonic blood circulatory system development, where progenitors of endothelial cells differentiate and assemble to form the basic vascular plexus.62,63
VEGF-activated VEGFR-2 triggers the phosphorylation of multiple proteins in the signalling pathways, such as Akt (protein kinase B), mTOR (mammalian target of rapamycin), Erk1/2 (extracellular signal-regulated kinase 1/2), FAK (focal adhesion kinase), and p70S6K (ribosomal protein S6 kinase), thereby facilitating tumour angiogenesis.64 These proteins are prime targets of VEGFR inhibitors.65
2.2 Bio-physiological significance of VEGFR-2
The majority of VEGFR-2 is expressed on blood and lymph vessel endothelial cells, where it stimulates integrins to promote cell motility and inhibit cell death.66 When Akt protein kinase is activated, it leads to vasodilation by enabling the production of endothelial nitric oxide synthase (eNOS),67 which forms nitric oxide. In addition, the activation of VEGFR-2 stimulates the production of von Willebrand factor (vWF) by endothelial cells.68 Since VEGFR-2 has been reported to have the highest degree of proangiogenic action, inhibiting it may have clinical implications.
VEGFR-2 is more active as a tyrosine kinase than VEGFR-1 but has a lower affinity for VEGF-A.69 Tumour cells release VEGF, which activates its receptor VEGFR-2, promoting vascular development and supplying oxygen and nourishment into hypoxic parts of tumour tissues.70 Multiple inquiries have demonstrated that the VEGF/VEGFR-2 signalling pathway exerts direct control over neuronal development and its function, particularly by promoting increased branching of axons.71,72
2.3 VEGFR-2 and its involvement in different pathways
VEGF-A binding to VEGFR-2 triggers the activation of the Ras/Raf/ERK/MAPK, PI3K/Akt, and PLCγ/PKC pathways, which influence angiogenesis in pathophysiological domains.41 The PI3K dimer governs angiogenesis, emigration, cell division, and viability with its p110 catalytic domain and p85 response regulator. VEGF-A upregulates PI3K and phosphorylates p85. Phosphatidylinositol-3,4,5-triphosphate accumulates when VEGFR stimulates PI3K, phosphorylating Akt/PKB. Akt/PKB upregulates and suppresses BAD, caspase-9, and proapoptotic proteins.50 Fig. 3 denotes the inhibitors and their targeting pathways associated with VEGFR.
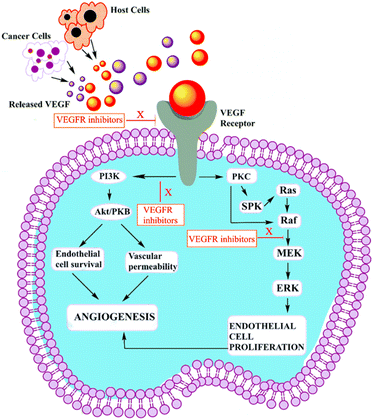 |
| Fig. 3 Role of VEGFR-2 inhibitor in tumour cell. | |
Upon VEGFR auto-phosphorylation, a T-cell-specific adaptor binds Tyr951 and links to Src. Src kinases form actin stress fibers and may activate PI3K in response to VEGF-A. VEGFR complex formation regulates Ras expression and starts the Raf-1-MEK-ERK signal cascade, which is essential for growth factor-induced cell division.73 When VEGF binds to VEGFR-2, some tyrosine residues of VEGFR-2 become autophosphorylated, such as Try801 on JMD, which further mediates the PLCg-PKC pathway and subsequently eNOS-NO or MEK-ERK, and Try951 on KID, mediates the TSAd-Src-PI3K-Akt pathway. Try1054 and Try1059 on TKD2 increase VEGFR-2 kinase activity.74
Try1175 regulates the PLCg-PKC, SHB-FAK-paxillin, and SHB-PI3K-Rac pathways, and Try1214 mediates cell migration via the NCK-p38-MAPK-APK2/3 pathways. These signalling networks regulate angiogenesis, endothelial cell survival, proliferation, and motility, as well as vascular permeability and penetration, through the action of VEGF/VEGFR-2.75 Fig. 4 (adapted from Modi, S. J. et al. 2019) depicts various signal transduction pathways and phosphorylation sites associated with VEGFR-2.51
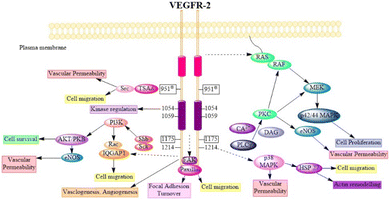 |
| Fig. 4 Physiology of VEGFR-2 in normal cells (adapted from Modi, S. J. et al. 2019).51 | |
3. USFDA-approved drugs targeting VEGFR-2
Small-molecule as VEGFR-2 inhibitors have indeed emerged as an important class of drugs in cancer treatment over the last few decades. Fig. 5 illustrates the structures of VEGFR-2 inhibitors that received USFDA approval for various types of cancer. The approval of these small-molecule inhibitors marks significant progress in cancer treatment, offering patients additional options and improving outcomes for certain types of cancer.47,76 The USFDA approved drugs principally targeting VEGFRs along with specific cancer types are mentioned in the Table 1. However, like all cancer treatments, they come with some moderate to serious side effects and considerations, and their efficacy is dependent on individual patients' cancer profile. While there are many VEGFR-2 inhibitors on the market, there have been documented cases of heart failure and a significant risk of haemorrhages associated with imatinib and other multikinase inhibitors treatments.100
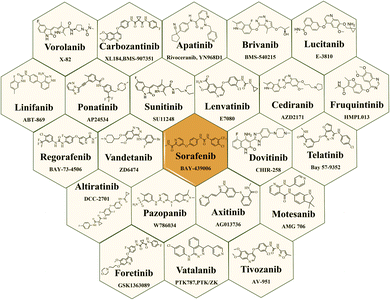 |
| Fig. 5 USFDA approved drugs targeting VEGFR-2 as anticancer agents. | |
Table 1 USFDA approved drugs targeting VEGFRs and other RTKsa
Sr. no. |
USFDA approved drugs |
Targets |
Cancer type |
Ref. |
mMTC: metastatic medullary thyroid cancer; HCC: hepatocellular carcinoma; mCRC: metastatic colorectal cancer; CRC: colorectal cancer; SCLC: small-cell lung cancer; NSCLC: non-small cell lung cancer; CML: chronic myeloid leukemia; ALL: acute lymphoblastic leukemia; mGIST: metastatic gastrointestinal stromal tumor; RCC: renal cell carcinoma; DTC: differentiated thyroid cancer; PHE: pseudomyogenic hemangioendothelioma. |
1 |
Vorolanib |
VEGFR-2, PDGFR-β, FLT3, and C-Kit |
RCC and lung cancer |
77 |
2 |
Cabozantinib |
MET and VEGFR-2 |
MTC and RCC |
78 |
3 |
Apatinib |
VEGFR-2 |
Gastric cancer |
79 |
4 |
Brivanib |
VEGFR-2 and 3, and FGFR-1, 2 and 3 |
Solid tumor, HCC, and mCRC. |
80 |
5 |
Lucitanib |
FGFR-1 and VEGFR |
Solid tumor, SCLC, and mCRC. |
81 |
6 |
Linifanib |
VEGFR-2 and PDGFR-β |
Breast cancer, CRC, liver cancer, and NSCLC. |
82 |
7 |
Ponatinib |
VEGFR-2, FGFR, PDGFR, SRC, RET, KIT, and FLT1 |
CML and Philadelphia chromosome-positive ALL |
83 |
8 |
Sunitinib |
VEGFR, PDGFR, and c-KIT. |
mGIST and RCC |
84 |
9 |
Lenvantinib |
VEGFR 1–3, FGFR 1–4, PDGFRα, KIT and RET. |
DTC, RCC, and HCC. |
85 |
10 |
Cediranib |
VEGFR-2 |
Ovarian cancer, Glioblastoma, liver cancer |
86 |
11 |
Fruquintinib |
VEGFR-1, -2 and -3 |
mCRC |
87 |
12 |
Regorafenib |
VEGFR1–3, TIE2 |
CRC, GIST, and HCC |
88 |
13 |
Vandetanib |
VEGFR-2 and EGFR |
MTC |
89 |
14 |
Sorafenib |
VEGFR-1, -2, -3, PDGFR-β, RET, c-Kit and Fms-like tyrosine kinase 3 |
Unresectable HCC and aRCC |
90 |
15 |
Telatinib |
VEGFR-2, VEGFR-3, and PDGFR |
PHE |
91 and 92 |
16 |
Altiratinib |
VEGFR-2, MET, and TIE2 (TEK) |
Glioblastoma |
93 |
17 |
Pazopanib |
VEGFR-1, -2, and -3, PDGFR-β and -α, and c-KIT |
Advanced soft-tissue sarcoma and RCC. |
94 |
18 |
Axitinib |
VEGFR-1, -2, and -3 |
aCRC and Advanced thyroid cancer |
95 |
19 |
Motesanib |
VEGFR-2, c-KIT, and PDGFR. |
Breast cancer, Bladder cancer, and thyroid cancer |
96 |
20 |
Foretinib |
VEGFR-2 and -3, c-MET, c-KIT and TIE-2 |
Ovarian cancer, gastric cancer, and lung cancer |
97 |
21 |
Vatalanib |
VEGFR-1, -2 and 3, EGFR, and FGFR-1 |
Solid tumor |
98 |
22 |
Tivozanib |
VEGFR-1, -2, and -3 |
Advanced or metastatic RCC |
99 |
4. Small-molecule VEGFR-2 inhibitors
Development of small molecules targeting VEGFR-2 with diverse molecular scaffolds using molecular hybridization and pharmacophore hybridization approaches holds promise for cancer treatment. Utilising different molecular scaffolds and pharmacophoric features, researchers are constantly aiming to discover highly potent, selective, and therapeutically effective lead molecules against various cancers. This strategy allows for the creation of a library of compounds with different chemical structures and properties, offering a wide range of options for optimizing drug candidates for cancer and overcoming resistance mechanisms.
Fig. 6 illustrates several types of molecular frameworks and heterocyclic components that have been investigated in recent years through the molecular hybridization approach and have demonstrated significant efficacy against VEGFR-2 and different cancer cells. Various cell lines with their codes tested for compounds covered in this review are mentioned in Table 2.
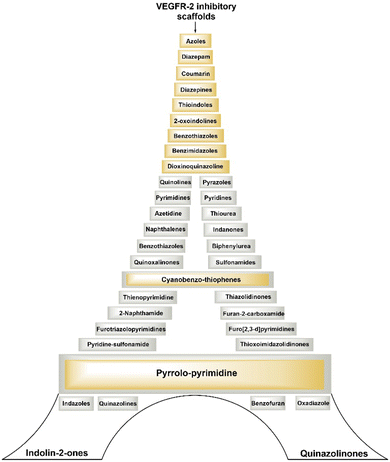 |
| Fig. 6 Various scaffolds covered in this review explored for potential VEGFR-2 inhibitory activity. | |
Table 2 Various cell lines and their codes tested for compounds covered in this review
Code |
Cell lines |
Code |
Cell lines |
MDA-MB-231 |
Human breast cancer cell line (MDA-MB-468, MDA-MB-435, MCF7, T-47D) |
UO-31, CAKI-1, 786-O |
Human renal cancer cell lines |
DU-145 |
Human prostate cancer cell line |
MRC5 |
Human normal lung cell line |
HCT-116, HT-29 |
Human colorectal cancer cell line (HCT-8, SW-620, SW-480, H460) |
U87MG |
Human glioblastoma cell line |
HepG2 |
Hepatocellular cancer cell line |
HT-29 |
Human colon cancer cell lines |
PC-3 |
Human prostate carcinoma |
HEK-293 |
Normal human embryonic kidney cells |
K562 |
Chronic myelocytic leukemia cell lines |
MKN-45, SNU-16, MKN-74 |
Human gastric cancer cell line |
HL60 |
Human acute lymphoblastic leukaemia cell line |
A549/ATCC, H1299, H3255, H1975, HCC-78, H460, HCC827 |
Non-small cell lung cancer cell line |
A498, ACHN |
Human renal cancer cell line |
RPE1 |
Human retinal pigment epithelial cell line |
A375 |
Human melanoma cancer cell line |
A431 |
Human epidermoid carcinoma cell line |
BxPC-3 |
Human pancreatic cancer cell lines |
Hu02 |
Human dermal fibroblasts cells |
HeLa |
Human cervical cancer cell line |
U251 |
Human glioblastoma cell line |
SNU-5, BGC-823 |
Human gastric adenocarcinoma cell line |
SMMC7721 MHCC97H |
Human hepatocellular carcinoma cell line |
HEK293, 293T |
Human embryonic kidney cell line |
HBE |
Human bronchial epithelial cells |
Huh7 |
Human hepatoma cell line |
HL-60 (TB) |
Human leukaemia cell lines |
SGC-7901 |
Human gastric adenocarcinoma |
LO2 |
Human fetal hepatocyte cell line |
U251 |
Brain tumour cell line |
HGC-27 |
Human gastric lymph node cancer cell line |
CACO-2 |
Human colon adenocarcinoma |
NCI-H522 |
Human lung adenocarcinoma |
HUVEC |
Human umbilical vein endothelial cells |
RPMI-8226 |
Human myeloma cell line |
A549 |
Human lung cancer cell line |
VERO |
Monkey kidney epithelial line |
SNB-75 |
Human brain tumour cell line |
MCF-10F |
Non-tumourigenic epithelial cell line |
KM12 |
Human colon cancer cell line |
MRC-5 |
Human Foetal lung fibroblast cells |
HOP-92 |
Human lung large cell carcinoma |
M-NFS-60 |
Bipotential murine hemopoietic cell line |
BJ |
Human neonatal normal foreskin fibroblasts |
Hep3B |
Human hepatoma cell line |
PANC-1 |
Human pancreatic epithelial cell line |
THLE2 |
Human normal liver cell line |
MCV-7 |
Merkel cell polyomavirus |
|
This review focuses on the design, synthesis, and structure–activity relationship of small compounds that have shown improved anticancer attributes in recent years. This article covers the development of compounds and their subsequent testing against VEGFR-2 to suppress the process of angiogenesis. Fig. 7 and 8 displays the structures of potent compounds against VEGFR-2 from various published reports that outperformed the reference standard, sorafenib, in terms of bioactivity results. Fig. 7 and 8 also displays a comparison of the IC50 values of the most potent compounds in the respective series and the IC50 values of the reference standard sorafenib obtained under similar bioassay conditions.
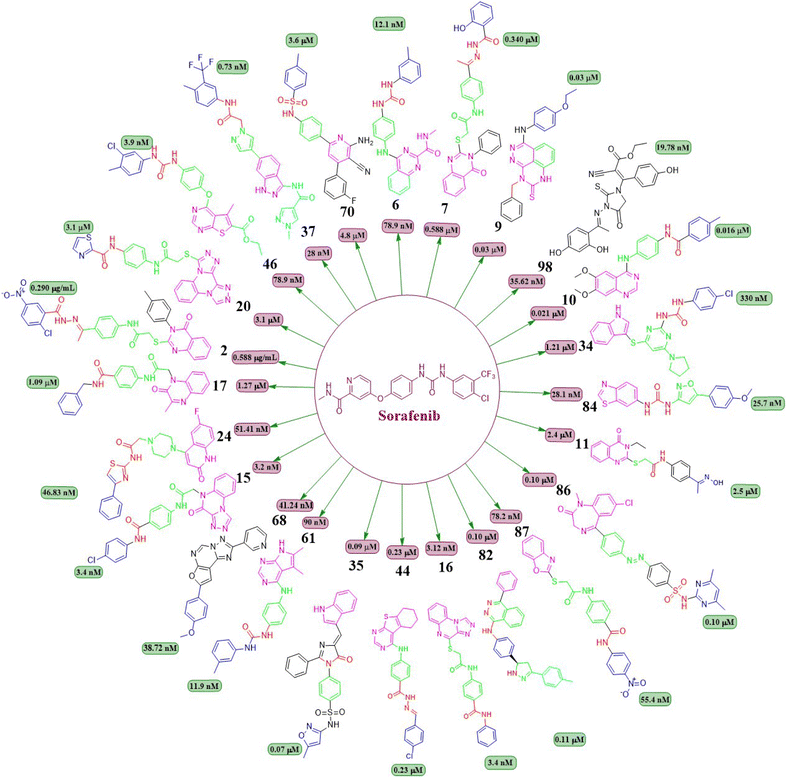 |
| Fig. 7 Compounds that displayed comparable inhibitory activity with sorafenib against VEGFR-2*. *Brown-coloured box indicates VEGFR-2 activity corresponds to sorafenib, and green-coloured box indicates activity corresponds to respective compounds using sorafenib as the reference standard in the bioassay. Color code description: heteroaromatic system; central aromatic ring; HBD–HBA; solvent-accessible region; terminal hydrophobic region. | |
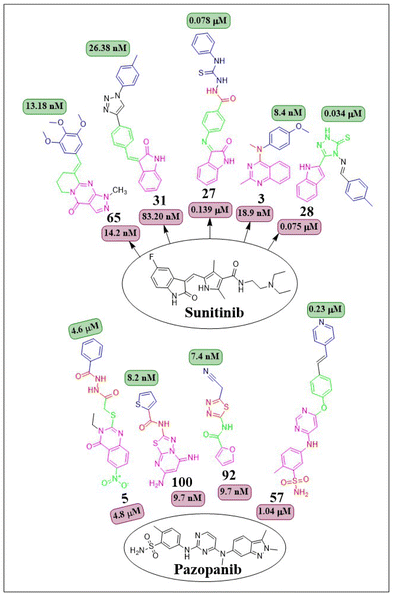 |
| Fig. 8 Compounds that displayed comparable inhibitory activity against VEGFR-2 using sunitinib or pazopanib as reference standard*. *Brown-coloured box indicates VEGFR-2 activity corresponds to sunitinib and pazopanib, while green-coloured box indicates activity corresponds to respective compounds using sunitinib and pazopanib as reference standard in the bioassays, respectively. | |
4.1 Quinazoline analogues
Ibrahim H. Eissa et al. (2021) investigated the features of quinazolin-4 (3H)-ones and evaluated them against the HepG-2 cell line of hepatocellular carcinoma. Compound 1 demonstrated the highest potency (IC50 = 4.33 ± 0.2 μg mL−1) against the VEGFR-2 kinase. It exhibited greater activity than doxorubicin (IC50 = 4.50 ± 0.2 μg mL−1) and was 78% as powerful as sorafenib (IC50 = 3.40 ± 0.25 μg mL−1). Compound 1 demonstrated exceptional binding energy of −59.62 kcal mol−1 in the active region of VEGFR-2.101
In a study conducted by El-Adl and co-workers (2021), some quinazolin-4(3H)-ones were modified and tested to determine their ability to inhibit VEGFR-2. The IC50 value for the most effective compound was found to be 0.290 ± 0.05 μg mL−1, which was greater than the reference standard sorafenib's IC50 value (0.588 ± 0.04 μg mL−1). Compound 2, which includes a 2-chloro-5-nitrophenyl group, has been identified as the most potent member. The activity is enhanced by incorporating electron-withdrawing groups on the 3rd position of the phenyl ring in the terminal hydrophobic region. The compound exhibited approximately 1.96-, 5.73-, and 4.39-fold higher activity towards MCF-7, HCT-116, and HepG2 cells, respectively, compared to sorafenib. The computed binding free energy of compound 2 at the active site of VEGFR-2 was found to be −58.02 kcal mol−1.102
In their study, Shruti Choudhary et al. (2021) afforded substituted quinazolines and tested their effectiveness against EGFR, Flk-1 (VEGFR-2), and PDGFR-β kinases. They discovered that compounds 3 and 4 exhibited greater potency, with kinase activity levels of 8.4 ± 2.2 nM and 9.3 ± 3.9 nM, respectively, compared to sunitinib, which had an activity of 18.9 ± 2.7 nM at VEGFR-2. Remarkably, it is worth noting that compounds 3 and 4 outperformed in the CAM experiment with IC50 values of 2.8 ± 1.1 μM and 3.1 ± 1.3 μM respectively, as compared to the reference standard erlotinib (IC50 = 3.1 ± 1.3 μM). The binding affinity of the complex between VEGFR-2 and compound 3 was −10.71 kcal mol−1, closer to that of axitinib (−13.25 kcal mol−1). The quinazoline structure binds to Phe1047 and forms a cation–pi interaction with the sidechain of Lys868. The 4th-position substituent of aniline (compound 3) forms interactions with Val848, Cys919, Leu1035, and Phe1047. The lack of methyl group on the 2nd position of quinazoline leads to a significant decrease in VEGFR-2 activity.103
A group of quinazolin-4(3H)-ones was developed and tested against VEGFR-2 by Abdallah E. Abdallah et al. (2021). The most effective compound 5, shown IC50 of 4.6 ± 0.06 μM, which was more significant than pazopanib's IC50 of 4.8 ± 0.07 μM. Also, it showed IC50 values of 30.85 ± 2.3 μg mL−1 against MCF cell lines, 17.23 ± 1.5 μg mL−1 against HepG2, and 26.10 ± 2.2 μg mL−1 against PC3. Results from docking investigations showed that compound 5 could bind to the active site of VEGFR-2 with a score of −7.42 kcal mol−1, effectively forming three crucial hydrogen bonds with the amino acid residues Glu885, Asp1046, and Cys919 in the appropriate way.104
In a recent study conducted by Soha R. Abd El Hadi et al. (2020), a series of urea-based quinazoline derivatives were meticulously designed and synthesized. These derivatives were then thoroughly evaluated for their VEGFR-2 inhibition profile. Compound 6 exhibited remarkable potency, with an IC50 value of 12.1 nM, surpassing that of sorafenib (IC50 = 78.9 nM). The NCI 60 cell line screen program was used to investigate most of the newly synthesized compounds. The docking study of the synthesized compounds showed that compound 6 has binding modes similar to the lead compound at the active site of VEGFR-2. The presence of a terminal phenyl ring is crucial for the activity. Introducing an amide or ester moiety at position 2 enhances the activity by creating an additional hydrogen bond with Cys919. Ester derivatives show higher activity when substituted with a 3-F group compared to the unsubstituted compound. The alignment of target compound 6 with sorafenib as co-crystallized ligand (PDB ID: 4ASD) showed a significant alignment with score of 0.790, suggesting a strong resemblance in their molecular fields.105
Ibrahim H. Eissa et al. (2020) conducted a study where they developed and tested a new series of quinazolin-4(3H)-ones for their potential antiproliferative outcomes. Compound 7 demonstrated a greater inhibitory action on VEGFR-2 (IC50 = 0.340 ± 0.04 μM) compared to sorafenib (IC50 = 0.588 ± 0.06 μM), which served as the reference drug. Upon comparing the cytotoxic activity of compound 7 against HepG-2, MCF-7, and HCT-116 cell lines with that of doxorubicin and sorafenib, it was discovered to be quite promising. According to docking studies, the novel compounds were found to have a strong inhibitory effect on VEGFR-2. The inhibition was attributed to their ability to make hydrophobic contact with the receptor's hydrophobic pocket and bind to the important residues Glu883 and Asp1044 in the active region. Compound 7 was successfully docked into the active site of the VEGFR-2 kinase enzyme, exhibiting a docking energy score of −56.21 kcal mol−1, which outperformed Sorafenib's score of −52.20 kcal mol−1. Substituting the hydroxyl group in position 7 for the phenyl group connected to the hydrazone moiety resulted in improved biological activity and binding affinity with VEGFR-2 compared to members that had substituted the chloro group (Fig. 9).106
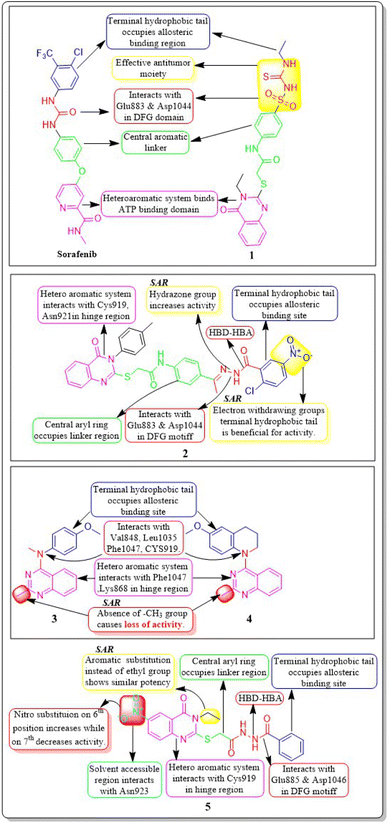 |
| Fig. 9 Pharmacophoric features and SAR of compounds 1 to 5. | |
Haoru Fan et al. (2019) developed and assessed dioxinoquinazoline derivatives as inhibitors of VEGFR-2. During the anti-tumour animal trials conducted on mice, the tumour exhibited a significant reduction, with a tumour growth inhibition (TGI) rate of 133.0%. After six days of administering a dose of 8, it was observed that it exhibited potent inhibitory action against VEGFR-2 (IC50 = 2.4 nM) and displayed remarkable antiproliferative effects on HUVEC cells (IC50 = 1.2 nM). Compound 8 exhibited significant action against the HEK293, LO2, and SMMC7721 cell lines, with respective concentrations of 7541 nM, 3855 nM, and 375.5 nM. The strong inhibitory impact can be attributed to the similar bonding sites between compound 8 and lenvatinib in the active site of VEGFR-2.107
In a recent study, Marwa El-Gazzar et al. (2019) synthesized and bio-evaluated new variations of pyridazino[3,4,5-de]quinazoline. Out of all, compound 9 showed greater anticancer activity against the HepG2 cell line and displayed excellent inhibition towards VEGF-2 (IC50 = 0.22 μM and 0.03 μM) compared to the reference standard sorafenib (IC50 = 1.06 μM and 0.03 μM, respectively). The synthesized compound achieved a docking score of −15.21 kcal mol−1 at the active binding site of VEGFR-2 (Fig. 10).108
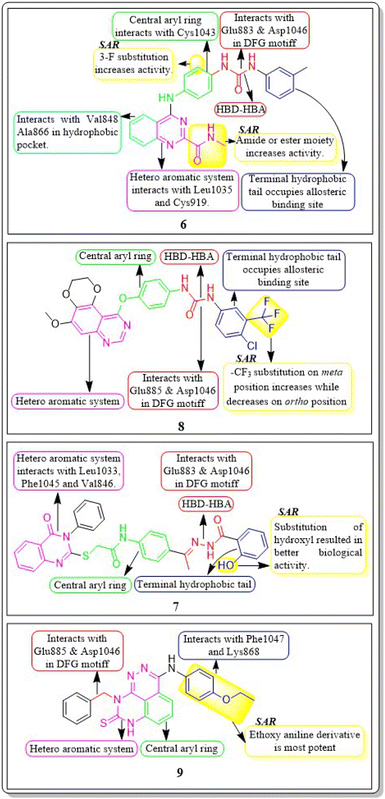 |
| Fig. 10 Pharmacophoric features and SAR of compounds 6 to 9. | |
Ru Wang et al. (2021) designed, synthesized, and analysed 6,7-dimethoxy-4-anilinoquinazoline analogues containing a diarylamide group. Compound 10 had the highest level of inhibition for VEGFR-2 (IC50 value of 0.016 ± 0.002 μM). This is superior to the reference standard, sorafenib, which had an IC50 value of 0.021 μM. Compound 10 also exhibited an antiproliferative impact against Hep-G2 and MCF-7, with concentrations of 7.5 ± 0.5 μM and 13 ± 2.8 μM, respectively. The positional influence of the functional group on the terminal phenyl is as follows: meta is greater than ortho, which is greater than para.109
A new set of quinazolin-4(3H)-one analogues was developed by Hazem A. Mahdy et al. (2021). Compound 11 has shown superior potency in the biological evaluation when compared to the other compounds. Compound 11 demonstrated impressive activity towards VEGFR-2 (IC50 = 2.5 ± 0.04 μM). The level of activity observed was nearly on par with sorafenib, which exhibited an IC50 value of 2.4 ± 0.05 μM. Compound 11 has shown remarkable activity against HepG-2 and HCT-116 cells, exhibiting IC50 values of 3.97 ± 0.2 μg mL−1 and 4.83 ± 0.2 μg mL−1, respectively. By comparison, its activity was 1.13 and 1.08 times greater than that of doxorubicin (IC50 = 4.50 ± 0.2 μg mL−1 and 5.23 ± 0.2 μg mL−1, respectively) and 1.17 and 0.91 times greater than that of sorafenib (IC50 = 3.40 ± 0.2 μg mL−1 and 5.30 ± 0.2 μg mL−1, respectively). Surprisingly, compound 11 exhibited a lower binding free energy (ΔG) of −59.90 kcal mol−1 compared to the reference drug's −52.20 kcal mol−1 when tested against the VEGFR-2 active site.110
2-Thioxobenzo[g]quinazoline derivatives were synthesized and assessed by Hatem A. Abuelizz et al. (2020). Compound 12 was the most effective out of all of them, exhibiting substantial action against VEGFR-2 at an IC50 of 44.4 ± 2.6 nM. It was unexpectedly important to note that compound 12 outperformed doxorubicin (IC50 = 28.5 ± 1.9 μM and 10.3 ± 0.8 μM, respectively) in terms of activity towards the MCF-7 (IC50 = 9.4 ± 0.7 μM) and HepG2 (IC50 = 26.0 ± 2.5 μM) cell lines. For 12 and sorafenib, the estimated free energies of binding were −9.669 kcal mol−1 and −11.01 kcal mol−1, respectively.111
Abdulmalik S. Altamimi et al. (2021) conducted a study where they synthesized and evaluated a novel set of 8-methoxy-2-trimethoxyphenyl-3-substituted quinazoline-4(3)-one compounds. The anticancer properties of compounds were determined by testing them against three different cell lines: MDA, A549, and HeLa. The researchers compared the results of these tests to those of docetaxel, which served as the reference drug. Compound 13 exhibited the highest effectiveness against cancer and was identified as a VEGFR-2 inhibitor with an IC50 value of 106 nM, in comparison to docetaxel, which had an IC50 value of 56.1 nM. Compound 13 exhibited potent activity towards the HeLa cell line, with an IC50 value of 2.8 μM, which was significantly greater than that of docetaxel (IC50 = 9.65 μM). Compound 13 exhibited a comparable cytotoxic effect against the MDA cell line (IC50 = 0.79 μM) compared to docetaxel (IC50 = 3.98 μM). Compound 13 binds to the ATP-binding pocket of VEGFR-2 with a docking energy of −7.3 kcal mol−1 (Fig. 10 and 11).112
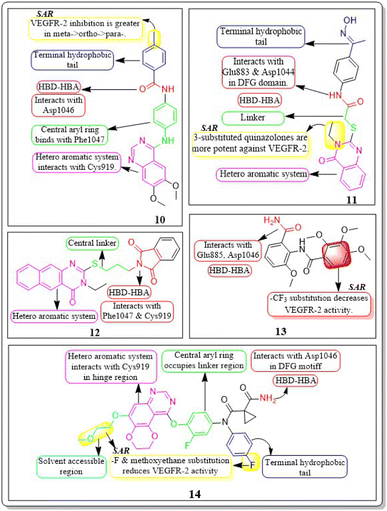 |
| Fig. 11 Pharmacophoric features and SAR of compounds 10 to 14. | |
Dengshuai Wei et al. (2019) developed a series of [1,4]dioxino[2,3-f]quinazoline derivatives. Compound 14 has the highest inhibition for VEGFR-2 (IC50 = 4.8 nM). Compound 14 inhibited HEK293 and LO2 cell lines (IC50 = 11.9 μM, 12.2 μM). Following additional analysis, compound 14 inhibited MHCC97H and HUVEC cell lines more effectively than cabozantinib (IC50 = 25.0 nM, 2.7 nM). Compound 14 showed a TGI (%) of approximately 120.4%. The –F substitution and methoxyethane moiety improve VEGFR-2 activity. Methyl substitution in the heteroaromatic system boosts both the activity and cytotoxicity of the molecule. Compound 14 established an H-bond with the Asp1046 residue at the active site of VEGFR-2.113
4.2 Quinoxaline derivatives
Nawaf A. Alsaif et al. (2021) constructed a novel class of [1,2,4]triazolo[4,3-a]quinoxalin-4(5H)-ones and tested their ability to inhibit the growth of two specific cancer cell lines, MCF-7 and HepG2. Compound 15 exhibited a significant inhibitory effect against VEGFR-2 (IC50 = 3.4 ± 0.3 nM) compared to sorafenib (3.2 ± 0.1 nM). The ΔG (binding free energy) of the synthesised compound 15 against VEGFR-2 was identified to be −21.59 kcal mol−1, while the reference sorafenib had a ΔG of −21.17 kcal mol−1. Upon molecular docking visualisation, it was shown that compound 15 interacts with the binding site of VEGFR in a manner that is analogous to that of sorafenib (Fig. 11).114
In their recent study, Alsaif et al. (2021) synthesised a new set of [1,2,4]triazolo[4,3-a]quinoxaline derivatives and assessed their viability as VEGFR-2 inhibitors. Compound 16 demonstrated exceptional potency, as evidenced by its IC50 value of 3.4 nM, which was closer to sorafenib's IC50 of 3.12 nM. By substituting a –Me group on the meta- or ortho-position on the phenyl group, the inhibitory effect was drastically diminished. Compound 16 demonstrated the most pronounced inhibitory effects on cell proliferation in the MCF-7 cell line, as evidenced by its IC50 values of 8.2 M, which were considerably lower than those of sorafenib (IC50 = 3.51 M).
A double staining experiment was performed utilising annexin V and propidium iodide (PI) to assess whether the inhibition of VEGFR-2 induced apoptosis in HepG2 cells. The experimental procedure involved subjecting HepG2 cells to compound 16 at a 5.4 μM concentration for a period of 24 hours. Compound 16 demonstrated a marginally reduced energy binding of −21.94 kcal mol−1 in comparison to sorafenib's energy binding of −22.10 kcal mol−1.115
In a recent study, Khaled El-Adl et al. (2021) conducted a thorough study of a novel set of quinoxaline-2(1H)-one derivatives. These compounds were specifically designed to investigate their potential to inhibit cell proliferation in three different cancer cell lines (HCT-116, HepG-2, and MCF-7). Compounds 17 and 18 demonstrated strong inhibition of VEGFR-2 with IC50 values of 1.09 μM and 1.19 μM, respectively, which were significant than that of sorafenib (IC50 = 1.27 μM). Typically, compounds 17 and 18, with their hydrophobic and electron-withdrawing distal benzyl moiety, exhibited greater activity against the three cancer cell lines compared to compounds with hydrophobic and electron-donating propyl, cyclohexyl, dimethyl, and ethyl moieties. The docking binding free energies of the synthesised compounds 17 and 18 against the VEGFR-2 active site have been estimated to be −19.34 kcal mol−1 and −19.65 kcal mol−1, respectively.116
Mohammed M. Alanazi et al. (2021) produced a novel class of bis([1,2,4]triazolo)[4,3-a:3′,4′-c]quinoxaline analogues and tested them against two human cancer cell lines, HepG-2 and MCF-7. Compounds 19 and 20 showed promising VEGFR-2 inhibitory activity, with IC50 values of 3.2 μM and 3.1 μM, respectively, compared to the reference drug sorafenib, which had an IC50 value of 3.1 μM. The synthesised compounds 19 and 20 have greater binding free energies (ΔG) than the reference candidate sorafenib (−26.50 kcal mol−1) against the active site of VEGFR-2 (Fig. 12).117
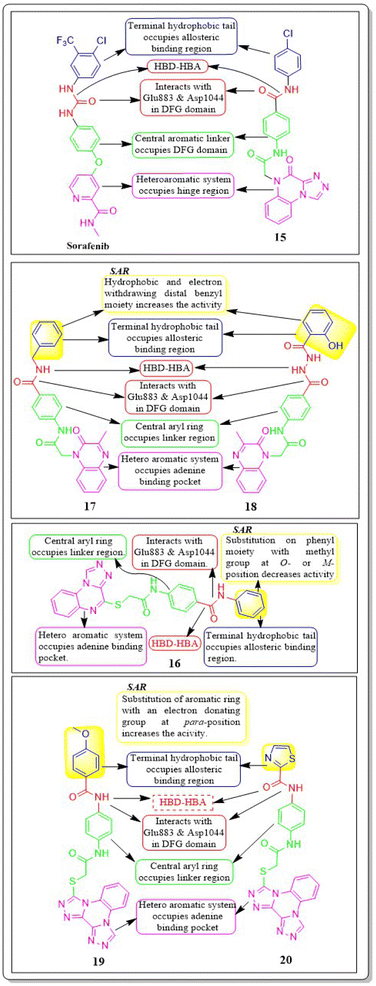 |
| Fig. 12 Pharmacophoric features and SAR of compounds 15 to 20. | |
4.3 Quinoline analogues
In their study, Xinyu Li et al. (2018) developed an assortment of 3-aryl-quinolin compounds specifically designed to interact with VEGFR-2. Out of all the compounds tested, 21 exhibited impressive activity at a value of 86 nM against VEGFR-2. Cell proliferation was effectively inhibited by Compound 21 in HUVEC, MCF-7, and Ishikawa cell lines, with IC50 values of 7.4 μM, 1.8 μM, and 1.8 μM, respectively. The inhibitory effects on the growth of MCF-7 breast cancer cells and the potential to prevent angiogenesis in laboratory settings are primarily associated with the presence of 2-methylpiperazine at the side chain terminal.118
Yuqin Yao et al. (2020) developed and analysed a new group of quinoline-thiourea motiff. Remarkably, compound 22 exhibited superior activity (IC50 = 7.0 ± 2.0 nmol L−1) compared to the reference standard Nintedanib (IC50 = 8.8 ± 3.6 nmol L−1) against VEGFR-2.119 In addition, compound 22 significantly decreased HUVEC growth, with an IC50 value of 71.0 ± 5.0 nmol L−1. The quinoline ring and thiourea-containing compound 22 established hydrogen binding with Cys919 (hinge region) and Glu885 on VEGFR-2. This interaction hindered the attachment of ATP to the ATP-binding site of VEGFR-2.119
In a recent study, Malose J. Mphahlele et al. (2020) successfully synthesised a series of pyrroloquinoline-5-carbaldehydes. In comparison to the reference standard camptothecin (IC50 = 10.37 μM), compound 23 exhibited the most potent inhibitory action (IC50 = 0.13 μM) against VEGFR-2 kinase. Compound 23 demonstrated significant efficacy against MCF-7, MDA-MB-231, and HEK293-T cell lines, with IC50 values of 11.33 ± 0.53, 9.64 ± 0.01, and >100, respectively. For increased activity, it is believed that the carbaldehyde group of these polycyclic compounds forms hydrogen bonds with the catalytic region of VEGFR-2, acting as a hydrogen acceptor. The free energy of binding for compound 23 has been identified to be −9.45 kcal mol−1. The antiproliferative activity of a 4-fluorophenyl group in the 2-position of the heterocyclic ring was found to be lower compared to when the halogen atom was on the meta position of the phenyl group (Fig. 13).120
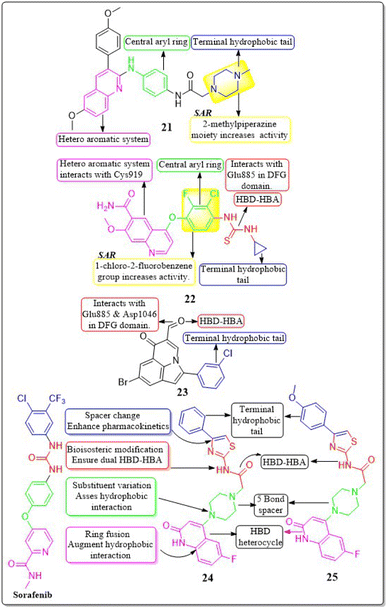 |
| Fig. 13 Pharmacophoric features and SAR of compounds 21 to 25. | |
4.4 Oxoquinolone derivatives
In a recent study, Abdelfattah Hassan et al. (2021) developed a novel series of 2-(4-(2-oxo-1,2-dihydroquinolin-4-yl)piperazin-1-yl)-N-(4-phenylthiazol-2-yl)acetamides.121 These analogues were then tested for their potential anticancer properties. Compounds 24 and 25 exhibited inhibitory activity with IC50 values of 46.83 ± 2.4 nM and 51.09 ± 2.6 nM, respectively. These values are comparable to the inhibitory activity of sorafenib, which has an IC50 value of 51.41 ± 2.3 nM. Substituting the nitrogen atom of the quinoline nucleus results in a decline in potency, whereas the presence of a fluoro substitution is crucial for maintaining activity. Compounds 24 and 25 exhibited binding interactions at the active site of VEGFR that were comparable to those of the standard drug sorafenib. Compound 24 revealed an interesting interaction with Cys919 in the hinge region of the enzyme. Specifically, quinoline-2(1H)-one formed a dual hydrogen bond with Cys919 through its nitrogen and oxygen atoms, with distances of 3.5 Å and 2.52 Å, respectively. Compound 24 formed hydrogen binding with Phe918 (2.87 Å) and Asp1046 (2.99 Å). Both compounds 24 and 25 replaced the central phenyl group of sorafenib with the piperazine moiety, effectively filling the gap between the enzyme's hinge region and gate area. Compound 24's 4-phenylthiazole part fit snugly into the enzyme's hydrophobic allosteric site, which was made up of the side chains of Ile888, Leu892, Val898, Val899, and Cys1024. This site was similar to the one occupied by Sorafenib's 3-trifluoromethyl-4-chlorophenyl part.121
4.5 Indole hybrids
Taghour et al. (2022) linked the thiazolidine-2,4-dione nucleus with 2-oxo-1,2-dihydroquinoline and 2-oxoindoline to generate new hybrid compounds. Out of the synthesised derivatives, compound 26 exhibited the highest potency with IC50 values of 116.3 nm against the VEGFR-2 enzyme. Replacing 2-oxo-1,2-dihydroquinolin with 2-oxoindolin linked to the thiazolidine-2,4-dione nucleus enhances the activity. The in silico docking binding free energy (ΔG) of compound 26 at the active site of the VEGFR-2 enzyme was −27.44 kcal mol−1, closer to the reference standard sorafenib's free energy of −26.30 kcal mol−1. Experiments utilising molecular dynamics (MD) simulations have shown that 26 exhibits a significant potential and optimal dynamics to fit tightly within the active region of VEGFR-2. The MM-PBSA analysis accurately determined the binding affinity to VEGFR-2 to be −92 kJ mol−1.122
The series of indoline-2-ones was designed by Mohamed A. Abdelgawad et al. (2022). The compound 27 had a VEGFR-2 kinase inhibition of 0.078 ± 0.003 μM, which is 1.78 times more effective than the reference standard sunitinib, which had an inhibitory activity of 0.139 ± 0.007 μM. The presence of the 1-phenylthiourea moiety as a hydrophobic tail in compound 27 increases the activity of VEGFR-2 compared to the 1,3,4-oxadiazole-2(3H)-thione moiety found in other derivatives. Compound 27 was accurately positioned at the VEGFR-2 active site, achieving a docking score of −20.1061 kcal mol−1.123
Sami A. Al-Hussain and co-workers (2020) investigated a variety of indolyl-1,2,4-triazole hybrids for their VEGFR-2 kinase profiles. It was noteworthy that three compounds; 28, 29, and 30 had remarkable action on the VEGFR-2 enzyme (IC50 = 0.085 ± 0.002 μM, 0.034 ± 0.001 μM, and 0.071 ± 0.002 μM, respectively), in contrast to sunitinib, which has an IC50 of 0.075 ± 0.002 μM. Compound 29 performed better in an in vitro study against the CAKI-1 and A498 cell lines than the reference standard sunitinib (IC50 = 4.93 ± 0.16 μM, 1.25 ± 0.04 μM, and IC50 = 0.89 ± 0.04 μM, 2.2 ± 0.1 μM, respectively). Compounds 29 and 30 had docking energy scores of −7.00 kcal mol−1 and −8.39 kcal mol−1, respectively, when inserted into the active site of the VEGFR-2 kinase enzyme, comparable to sunitinib (−7.13 kcal mol−1). An important hydrogen link was discovered between the carbonyl oxygen of Glu917 and the NH of the indolyl molecule. Furthermore, one H-bond was provided by the Cys919 residue to the nitrogen atom in the 1,2,4-triazole core (Fig. 14).124
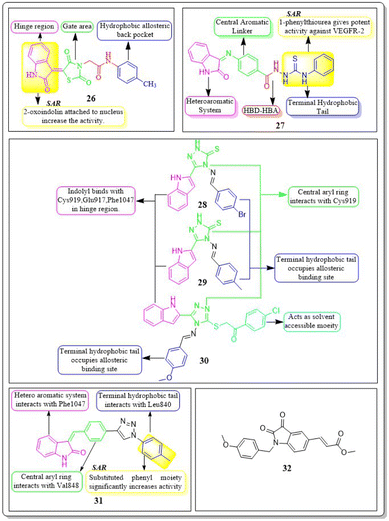 |
| Fig. 14 Pharmacophoric features and SAR of compounds 26 to 31. | |
De-pu Wang et al. (2021)125 synthesised and bioevaluated a novel class of 1,2,3-triazoles. Among all the derivatives, compound 31 exhibited a lower level of toxicity towards HUVECs and had a greater capacity to inhibit kinase activity compared to sunitinib. In addition, it exerted potent inhibition on MKN-45 and HT-29 cells, with IC50 values of 1.92 ± 0.37 μM and 1.61 ± 0.45 μM, respectively.
Compound 31 interferes with the phosphorylation of the VEGFR-2 protein on HUVECs, proven by tube formation assessment, transwell, and western blot tests. The in vivo study using the zebrafish model labelled with VEGFR-2 indicated that compound 31 exhibited more anti-angiogenic action compared to sunitinib. Compound 31 demonstrated stability in binding to the active site of VEGFR-2, as indicated by the findings of docking and MD simulations. The inhibitory effect on VEGFR-2 was more pronounced when unsubstituted phenyl groups or phenyl groups substituted with electron-donating groups were introduced, as compared to analogues replaced with electron-withdrawing groups.125
Yunsong Chang and co-workers (2020) developed and synthesized a series of 5-(2-carboxyethenyl)isatin derivatives. These compounds were then tested to determine their impact on cell viability. Remarkably, 32 exhibited an outstanding capacity to specifically target and destroy liver hepatocellular carcinoma HepG-2 cells, with an impressive IC50 value of 7.13 nM. Compound 32 demonstrated significant efficacy in inhibiting HepG2 cell migration, inducing apoptosis, and arresting the cell cycle at the G2/M phase. In addition, 32 greatly decreased actin organisation and tube formation in HUVECs. Chick chorioallantoic membrane assays were utilized to assess the in vivo antiangiogenic effects of compound 32. It was found that VEGF and its downstream signalling pathways, including the PI3K/Akt/mTOR pathway and the mitogen-activated protein kinase pathway (ERK), were accountable for the effects caused by compound 32.126
New rigid sorafenib analogues based on the indole ring were developed by Rawan M. Sbenati et al. (2020). The most potent compound, 33, successfully inhibited VEGFR-2 (IC50 = 95.7 ± 3.2 nM). Surprisingly, it was noteworthy to mention that derivative 33 showed better results (IC50 = 8.01 μM, 4.31 μM, and 1.95 μM) than sorafenib (IC50 = 8.62 μM, 7.55 μM, and 7.22 μM) against Hep3B, Huh7, and Hep-G2 cell lines, respectively. The N-methylpiperazinyl moiety enhances the activity of VEGFR-2 inhibition. Urea moiety binds with essential amino acids (Glu885 and Asp1046) by forming two hydrogen bonds.127
In a recent study by Sravani Sana et al. (2020), a new scaffold was developed through the integration of pyrimidine and thioindole. The inhibitory potency of the indole–pyrimidine conjugate was tested, showing an IC50 value of 330 nM. Compound 34 demonstrated significant inhibition of the MDA-MB-231, HepG, A549, and PC-3 cell lines, with IC50 values of 5.85, 7.87, 6.41, and 10.43 μM, respectively. In molecular docking experiments, it was observed that compound 34 effectively formed hydrogen-bonding with the catalytically active residues Asp-1046 and Glu-885 of VEGFR-2. It was discovered that improving the benefits of VEGFR-2 inhibition requires modifications to the benzene ring. The order of reactivity is 4-Cl > 2-CH3-4-Cl > H > 4-OMe > 3,5-diOMe. Adding the -chloro group to the para position of phenyl urea 34 significantly increased the enzyme inhibition, surpassing the electron-donating and unsubstituted analogue by approximately 8 and 10 times, respectively.128
Hanaa M. Roaiah et al. (2018) synthesised and analyzed several new indole derivatives. Compound 35 had superior inhibitory activity against VEGFR-2 (IC50 = 0.07 μM) compared to that of sorafenib's IC50 of 0.09 μM. Compound 35 exhibited a wide range of anticancer activity on forty-seven cell lines, with GI% values ranging from 31% to 82.5%. The docking score of 35 was found to be −15.08 kcal mol−1, which is similar to that of sorafenib (−15.19 kcal mol−1) when targeting the active site of VEGFR-2 (Fig. 15).129
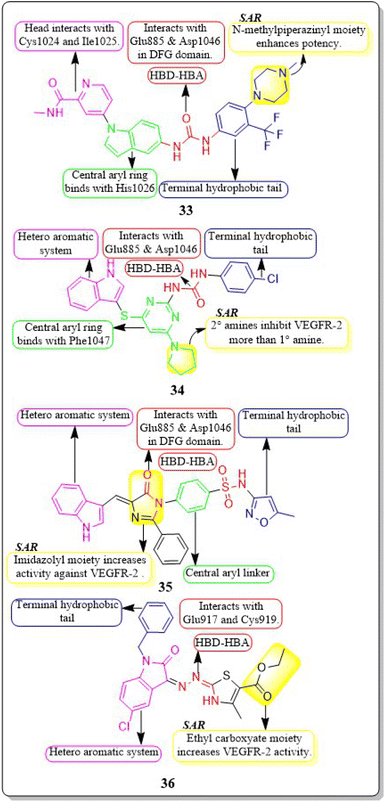 |
| Fig. 15 Pharmacophoric features and SAR of compounds 33 to 36. | |
Huda K. Mahmoud et al. (2020) modified and examined sunitinib analogues to assess their ability to inhibit VEGFR-2. The potency of compound 36 in inhibiting the growth of CAKI-1 and A498 cell lines was discovered to be the highest. Compound 36 exhibited substantial inhibition (IC50 = 0.092 ± 0.003 μM) against VEGFR-2. Compound 36, with a binding energy score of −6.70 kcal mol−1, tightly fits into the ATP-binding site of VEGFR-2. Phenyl substitution significantly decreases activity, while the presence of a terminal ethyl carboxylate moiety enhances it. Replacing the dihydrothiazole ring with diazane and toluyl groups enhances the inhibitory effect on VEGFR-2, but it also leads to an increase in cytotoxicity.130,131
A collection of new derivatives of 2-(4-(1H-indazol-6-yl)-1H-pyrazol-1-yl)acetamide has been developed, synthesised, and tested for their biological properties by Xing-Rong Wang et al. (2021).131 These compounds, 37, 38, and 39, demonstrated remarkable inhibitory effects on both VEGFR-2, with IC50 values of 0.73 nM, 1.4 nM, and 1.6 nM, respectively. An amide moiety on the 3rd position of indazole enhances activity compared to those with imine or amino coupling.
Having a –Me group at the para position is more favourable than at the ortho position. The presence of –C
O decreases the steric hindrance in the binding region and increases the affinity of the kinase. Compounds containing an amide moiety at the 3rd position of indazole exhibit higher activity compared to those with imine or amino coupling. The compounds 37, 38, and 39 showed satisfactory anti-proliferation activity against HGC-27 tumour cells, with IC50 values of 0.021 ± 0.01, 0.63 ± 0.24 μM, and 0.36 ± 0.11 μM, respectively (Fig. 16).131
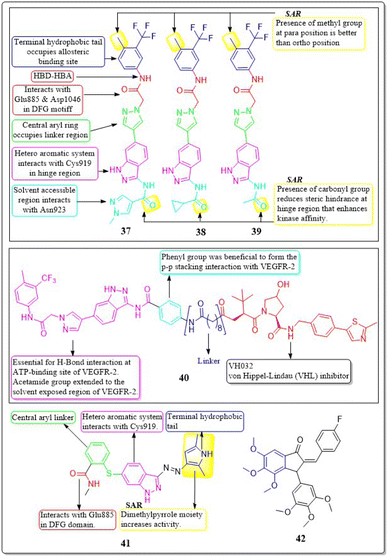 |
| Fig. 16 Pharmacophoric features and SAR of compounds 37 to 42. | |
In their study, Xing-Rong Wang et al. (2022) investigated a group of new VEGFR-2-PROTAC degraders to improve the effectiveness of protein degradation and its anti-tumour properties. The development and production of VEGFR-2-PROTAC degraders were guided by the Lys residue zone located on the surface of the VEGFR-2 receptor. Out of all the degraders that were developed, compound 40 showed the highest level of degradation activity against the VEGFR-2 protein in HCG-27 cells when tested in a controlled environment (DC50: 0.084 ± 0.04 μM, D%: 73.7%). This led to a decrease in the amount of time it takes for the VEGFR-2 protein to be produced without affecting the expression of the mRNA of VEGFR-2 in HGC-27 cells. The 8-carbon alkanedioic acid side chain serves as a vital connector, offering sufficient flexibility to incorporate the terminal ester bond into the confined groove on the exposed part of the solvent. The substitution of the ester bond with VHL-L likely induced the ubiquitination of Lys residues, resulting in the destruction of VEGFR-2.132
Na Wei et al. (2018) synthesized axitinib analogues. With an activity of 88 nM, compound 41 outperformed the other variants. Compound 41 exhibited notable anti-proliferative effects against HUVEC cells in vitro, with an IC50 value of 99.29 ± 0.78 μM. Molecular modelling revealed that compound 41 maintained the activity via forming a hydrogen bonding between the amino of the pyrrole moiety and the carbonyl oxygen of the Lys920 backbone. Substituting a 4-methoxy-2,6-diaminophenyl group for the terminal dimethylpyrrole group results in a dramatic decrease in activity.133 The angiogenic activity of a series of fluorinated benzylidene indanones was designed, synthesised, and evaluated by Ankita Srivastava et al. (2020). VEGF was inhibited by 15–22% in a concentration-dependent pattern by compound 42. 17.2% VEGF inhibition was observed in MCF-7 cells, whereas 19–33% inhibition was induced by doxorubicin at different concentrations. Doxorubicin inhibited 33% of VEGF at its IC50.134
4.6 Thienopyrimidine derivatives
Yara El-Dash et al. (2021) introduced a set of fresh hybrid compounds that combine hexahydrobenzo [4,5]thieno[2,3-d]pyrimidine with aminothiazole scaffolds. Compound 43 exhibited remarkable potency against VEGFR-2 with IC50 value of 62.48 ± 3.7 nM. Based on the in vitro antiproliferative assay, it was found that compound 43 showed the highest potency against SNB-75, SF-295, and CAKI-1 cell lines when compared to sorafenib. When the chlorine atom in compound 43 is replaced with a bromine atom at the para position, there is a decrease in activity. Based on the molecular docking study, it was found that compound 43 exhibited a binding pattern that aligned with its VEGFR-2 inhibitory activity. The binding free energy of compound 43 was determined to be −9.0323 kcal mol−1, while the reference standard drug sorafenib had a binding free energy of −10.2499 kcal mol−1.135
In a recent study conducted by Souad A. El-Metwally et al. (2021), researchers explored derivatives of thieno[2,3-d]pyrimidines that share structural similarities with VEGFR-2 inhibitors. Compound 44 demonstrated exceptional potency, with an IC50 value of 0.23 ± 0.03 μM, which is comparable to the reference compound, sorafenib (IC50 = 0.23 ± 0.04 μM). This compound exhibits remarkable activity against HepG2, HCT-116, and the VEGFR-2 kinase enzyme, making it highly potent. On the other hand, when the electron-withdrawing group (p-Cl) in 44 is replaced with an electron-donating one (p-OCH3), it unfortunately results in a loss of potency. The docking binding free energies of synthesised compound 44 have been found to be −26.27 kcal mol−1 against the active site of VEGFR-2.136
Eman Z. Elrazaz and co-workers (2021) conducted a study where they synthesised and tested a range of 4-substituted thieno[2,3-d]pyrimidine derivatives. Compounds 45 and 46 displayed remarkable inhibitory activity, with IC50 values of 5 and 3.9 nM, respectively. A terminally substituted phenyl ring is crucial for achieving optimal activity. The ether linkage demonstrated a more pronounced inhibitory effect in comparison to the amine linker. During the molecular dynamic simulation, the average binding energy for compounds 45 and 46 was estimated to be −375.65 ± 2.31 kJ mol−1 and −381.08 ± 1.4 kJ mol−1, respectively (Fig. 17).137
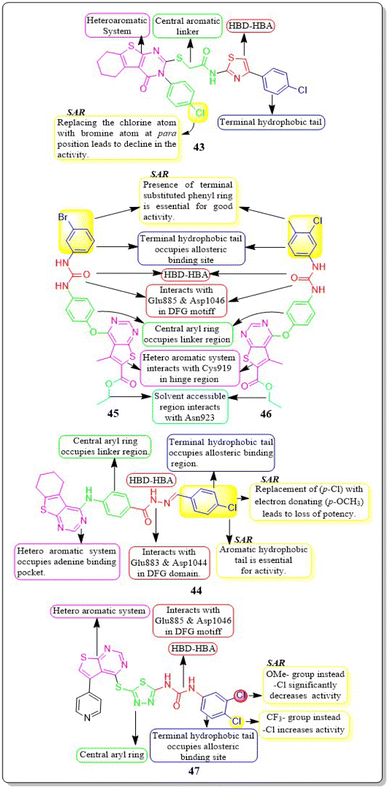 |
| Fig. 17 Pharmacophoric features and SAR of compounds 43 to 47. | |
Compounds based on thienopyrimidine that possess diaryl urea functionality were developed and synthesised by Aram Faraji et al. (2021).138 Compound 47 showed stronger anti-proliferative action against the PC3 cell line than sorafenib, according to the MTT assay.
Compound 47, with an IC50 value of 3.6 μM, triggered apoptosis in PC3 cells when compared to sorafenib as a standard drug. Results showed that 47 inhibited VEGFR-2 phosphorylation when tested by western blotting. VEGFR-2 phosphorylation was disrupted in a concentration-dependent manner during western blotting on the PC3 cell line, resulting in a noticeable decrease in band size at a dose of 5.4 μM. The proliferation of HUVEC cells is inhibited by compound 47, which has an IC50 value of 7.8 μM. It should be mentioned that compound 47 had a cytotoxic impact against the normal fibroblast Hu02 cell line that was similar to sorafenib's (IC50 = 40.0 ± 0.5 μM). The binding free energy values for 47 and sorafinib have been identified to be −8.49 and −8.57 kcal mol−1, respectively. Aram Faraji et al.138 also developed and synthesised diaryl-urea-functionalized thienopyrimidines. Out of all the compounds with an IC50 value of 3.6 μM, 48 showed significant activity against the PC3 prostate cancer cell line.
Compound 48 exhibited a roughly fourfold inhibition of PC3 cell proliferation in comparison to sorafenib. The CAM assay demonstrated a significant 48% inhibition of blood vessel development. The western blot analysis for compound 48 demonstrated the suppression of VEGFR-2 phosphorylation. In addition, compound 48 had a similar cytotoxic impact against the normal fibroblast Hu02 cell line (IC50 = 34.3 ± 0.3 μM) as sorafenib (IC50 = 40.0 ± 0.5 μM). The computed binding free energies for 48 and sorafenib were −8.49 kcal mol−1 and −8.57 kcal mol−1, respectively.138
Amna Ghith et al. (2018) synthesised and assessed a number of new thieno [2,3-d] pyrimidine derivatives. With an IC50 of 2.27 μM, the most powerful derivative, 49, showed noteworthy efficacy against VEGFR-2. These findings were also explained by molecular docking experiments, which showed that the urea-based derivatives were able to build an important network of contacts with the residues Cys919, Glu885, and Asp1046. For maximum activity, a terminal substituted phenyl ring is required.139
Rasoul Motahari et al. (2022) have successfully designed and synthesised new variations of tetrahydropyridothienopyrimidine-based compounds. Compound 50 exhibited significant action against MCF-7, PC-3, SW480, HEPG-2, HUVEC, MRC5, and MCF7 cell lines, with IC50 values of 2.67 ± 0.21 μM, 11.35 ± 0.09 μM, 6.84 ± 0.05 μM, 7.20 ± 0.03 μM, 2.09 ± 0.08 μM, 38.10 ± 0.81 μM, and 2.67 ± 0.21 μM, respectively. Compound 50 demonstrates a little less potent inhibitory effect on developing CAM in comparison to the positive control.140
4.7 Pyrimidine motifs
Asmaa M. Sayed et al. (2021) designed a series of sulfonamides equipped with hydrazone linked to dimethyl and/or diethyl malonates. Compounds 51–53 were discovered to be the most effective derivatives, with IC50 values of 0.14 ± 0.02 μM, 0.15 ± 0.02 μM, and 0.15 ± 0.02 μM, respectively, showing the greatest inhibition of VEGFR-2. Compounds 51–53 were discovered to have higher anticancer activity than the other compounds due to the presence of their heteroaromatic pyrimidine, isoxazole, and pyrazolidine moieties, as well as their diazene linkers. The strongest VEGFR-2 inhibitory activity was found in compound 51, which has a six-membered heteroaromatic pyrimidine ring and pyrazolidine tail, as contrasted with compounds 52 and 53, which only had one of the two aforementioned rings. Pyrimidine 51, a heteroaromatic ring, showed more VEGFR-2 inhibitory action than izoxazole 52. Compounds 51–53 have yielded binding free energy (ΔG in Kcal mol−1) of −119.58, −119.12, and −119.05, respectively. Compounds 51–53 exhibited the highest potency among all the derivatives tested against the three cancer cell lines, HepG2, HCT116, and MCF-7, with IC50 values of (6.43 ± 0.5, 9.66 ± 0.8, 10.57 ± 0.9 μM), (8.65 ± 0.7, 7.49 ± 0.6, 14.29 ± 1.3 μM), and (8.97 ± 0.7, 10.13 ± 0.9, 13.82 ± 1.1 μM), respectively.141
In their study, Ghada H. Al-Ansary et al. (2021) developed a novel set of biphenylurea/thiourea derivatives conjugated with heteroarylsulfonamide motifs. The researchers then examined the vitality of HUVEC, conducted a migration test, and performed western blot analysis using sorafenib as a reference standard. The synthesised compounds showed more efficacy than sorafenib in all three assays. Compound 54 exhibited superior antiproliferative activity against HUVECs, with an IC50 value of 10.54 μM, in comparison to sorafenib (IC50 = 17.74 μM). The investigated compounds exhibited a notable suppression of HUVEC cell migration mediated by VEGF, with the most significant effect reported at a dose of 10 μM. Compound 54 has shown a higher potency, inhibiting up to 86% of cell migration compared to the reference drug sorafenib, which only inhibited 75.56%. The compounds were evaluated in vitro for their cytotoxic effects on MCF-7, HepG2, CaCo-2, and HCT-116 cancer cell lines, as well as normal RPE1 cells. The results indicated that the compounds have dual properties, acting as both antiangiogenic and cytotoxic agents.142
Guoshun Luo et al. (2018) developed and synthesised a variety of 2,4-disubstituted pyrimidines. Compound 55 had the highest potency against the VEGFR-2 enzyme (IC50 = 0.085 μM). 55 effectively inhibited the Raf-1/MAPK/ERK pathway, causing apoptosis and suppressing migration in MCF-7 and Ishikawa cells (IC50 = 0.81 μM and 5.93 μM, respectively). Furthermore, 55 substantially decreased blood vessel formation in CAM while also inhibiting VEGFR-2 protein expression (Fig. 18).143
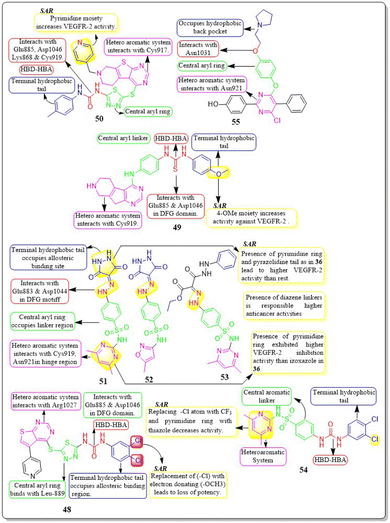 |
| Fig. 18 Pharmacophoric features and SAR of compounds 48 to 55. | |
Mahitab K. Sobhy et al. (2019) designed and synthesised a group of 6,7-dihydro-5H-cyclopenta[d]pyrimidines, which were then evaluated for anticancer activity. Compound 56 demonstrated VEGFR-2 inhibition with an IC50 value of 0.85 μM. Compound 56 exhibited a significant pharmacophore mapping fit value of 9.21 and a greater docking score of −29.29 kcal mol−1. The effectiveness diminishes when the third position of the terminal phenyl ring contains a methyl group instead of a trifluoromethyl group.144
Wuji Sun et al. (2018) synthesized a new series of derivatives based on pyrimidine. Inhibitory activity against VEGFR-2 was observed to be greater in compound 57 (IC50 = 0.23 M) than in the reference standard pazopanib (IC50 = 1.04 M). It is worth noting that compound 57 exhibited remarkable cellular potencies against the A549 and HepG2 cell lines (IC50 = 13.17 μM and 11.94 μM, respectively), in contrast to Pazopanib (IC50 = 21.18 μM and 36.66 μM). In contrast to Pazopanib (ΔG = −10.28 kcal mol−1), Compound 57 (ΔG = −10.37 kcal mol−1) demonstrated notably superior binding capacities. These results corroborated the compound's exceptional inhibitory potency against the A549 and HepG2 cell lines.145
A new group of substituted 4-amino-2-thiopyrimidines was developed, synthesised, and assessed by Heba T. Abdel-Mohsen et al. (2019). Compound 58 demonstrated impressive inhibitory activity against VEGFR-2 (IC50 = 0.17 μM) and BRAF (IC50 = 0.15 μM). Compound 58 showed significant inhibition of MCF7 and T-47D cell lines, with IC50 values of 13.02 μM and 2.18 μM, respectively. The compound 58 exhibited a VEGFR-2 inhibition of 46.00 4.11 ng mL−1 against the MCF7 cell line, resulting in an impressive 84 percent inhibition. Through molecular docking, the interaction pattern of the co-crystalized ligand in VEGFR-2 binding sites was accurately replicated, yielding energy scores of −15.19 kcal mol−1. Furthermore, the docking poses accurately replicated the crucial interactions within the binding site regions of VEGFR-2 (Glu885, Cys919, and Asp1046).146
A group of researchers led by Adel A. Marzouk et al. (2020) synthesized and bio-evaluated novel 1,6-dihydropyrimidin-2-thiol derivatives. Compound 59 exhibited remarkable potency, displaying an IC50 value of 198.7 nM against VEGFR-2. The docking study revealed that the new compounds fit well into the active site of VEGFR-2, with binding free energies ranging from −9.80 to −11.25 kcal mol−1. This is slightly lower than the binding free energy of sorafenib, which was −12.12 kcal mol−1. Through in vitro five-dose tests, it was observed that the GI50 values ranged from 19 to 100 μM, indicating its potency. Additionally, the selectivity ratios at the GI50 level ranged between 0.75 and 1.71, further highlighting its effectiveness (Fig. 19).147
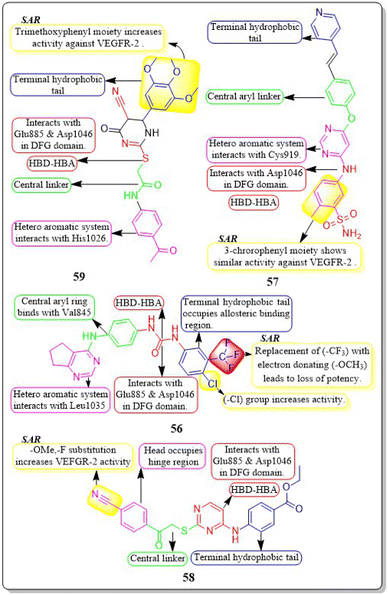 |
| Fig. 19 Pharmacophoric features and SAR of compounds 56 to 59. | |
4.8 Pyrrolo[2,3-d] pyrimidine analogues
Mai Adel et al. (2022)148 afforded a new set of compounds by connecting pyrrolo[2,3-d]pyrimidine to flurorinated diarylureas, which were then tested for their VEGFR-2 activity. The compound 60 exhibited the highest level of action, with a potency of 52.4 nM, compared to the IC50 of 78.9 nM for the standard drug sorafenib. The substitution of a trifluoromethyl group on the meta position of the benzene ring enhances the activity, whereas the presence of a halogen and/or methoxy moiety on the benzene ring decreases the activity against VEGFR-2.
The docking investigation demonstrated that the synthesised compounds effectively occupied the binding site of VEGFR2, with docking scores ranging from −8.76 to −10.28 kcal mol−1. This is comparable to the binding free energy of sorafenib, which is −10.12 kcal mol−1. In addition, Mai Adel et al. (2022) synthesized compounds based on pyrrolo[2,3-d]pyrimidine as inhibitors of VEGFR-2. The pyrrolo[2,3-d]pyrimidine derivatives (61 and 62), which have an m-toluyl urea tail connected through a NH or ether linker, exhibited the most potent nanomolar inhibition against VEGFR-2. Specifically, compounds 61 and 62 displayed an IC50 value of 11.9 nM and 13.7 nM, respectively, superior to that of sorafenib (IC50 = 90 nM). Compounds 61 and 62 exhibited antiproliferative action against HUVEC cells, with IC50 values of 0.31 ± 0.01 μM and 3.74 ± 0.18 μM, respectively.148
A series of pyrazolo[3,4-d] pyrimidines was discovered and evaluated by Dan-Xia Ying et al. (2022). It is interesting to note that compound 63 showed higher activity against the HepG2 and T47D cell lines (IC50 = 5.90 ± 0.06 μM and 5.57 ± 1.55 μM, respectively) compared to sorafenib (IC50 = 9.05 ± 0.54 μM and 7.41 ± 3.08 μM). Docking studies showed that 63 exhibited the ability to form two hydrogen bonds with the Cys919 and Asp1046 residues at the active sites of VEGFR-2, mirroring the behaviour of sorafenib. Replacing the terminal chlorophenyl moiety with a toluyl moiety results in a notable decrease in VEGFR-2 activity, whereas having the chlorophenyl moiety enhances the activity.149
In their recent study, Mater H. Mahnashi et al. (2022) introduced a set of 1,2,5-oxadiazole-2-oxides. Compound 64 displayed remarkable activity, with an IC50 value of 0.092 μM, demonstrating VEGFR-2-inhibitory potential similar to that of sorafenib (IC50 = 0.049 μM). In addition, compound 64 exhibited superior anticancer activity when compared to the reference drug, sorafenib. It displayed IC50 values of 13 μM, 11.5 μM, and 11.6 μM against the MDA-MB-231, HepG-2, and A2780CP cell lines, respectively. The free energy of binding for 64 was found to be −11.3747 kcal mol−1 (Fig. 20).150
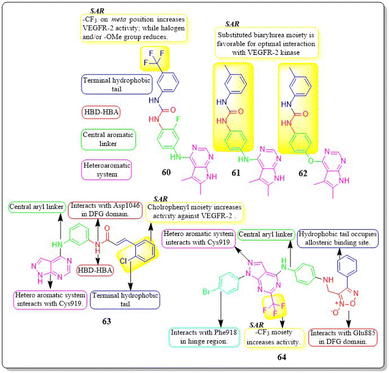 |
| Fig. 20 Pharmacophoric features and SAR of compounds 60 to 64. | |
4.9 pyrazolo[3,4-d] pyrimidine hybrids
Zukela Ruzi and co-workers (2022) discovered a group of new pyrazolo[3,4-d] pyrimidine analogues. It is worth noting that compound 65 exhibited stronger inhibition of VEGFR-2 (IC50 = 13.18 ± 0.17 nM) compared to the reference standard sunitinib (IC50 = 14.2 ± 0.02 nM). Compound 65 showed IC50 values of 0.03 ± 0.01 μM, 0.04 ± 0.03 μM, 0.19 ± 0.01 μM, 0.09 ± 0.01 μM, and 1.61 ± 0.02 μM against HT-29, HCT-116, HGC-27, HeLa, and MDA-MB-231 cell lines, respectively. Additionally, it effectively inhibited the migration, adhesion, and tube formation activities of HUVEC cells. Compound 65 exhibits affinity for the VEGFR-2 protein, specifically targeting Val-848, Phe-1047, Leu-1035, Cys-919, and Phe-918 amino acid residues as its primary binding sites. The binding energy for 65 was lower than −8.30 kcal mol−1.151
The researchers Yuanyuan Wang et al. (2018) developed, synthesised, and conducted biological evaluations on a set of 1H-pyrazolo[3,4-d]pyrimidine derivatives. Compound 66 exhibited significant inhibitory effects on the growth of BRAFV600E-expressing A375 (IC50 = 1.74 μM) and H-29 (IC50 = 6.92 μM) cells, as well as VEGFR-2-expressing HUVEC (IC50 = 5.89 μM). Compound 66 exhibited potent inhibition against BRAFV600E (IC50 = 0.171 μM) and VEGFR-2 (IC50 = 0.779 μM) and showed notable anti-proliferative effects on three cell lines (HUVEC, A375, and HT-29). Replacing a –CH3 group on the first position of the pyrazolopyrimidine ring is found to be more efficacious than using ethyl and isopropyl. Replacing the benzene ring at the para position is significantly more efficient. The binding-free energies were determined through calculations utilising the MM-PBSA and MMGBSA programmes. It was discovered that 66 demonstrated reduced effectiveness as a ligand in comparison to sorafenib.152
Qiumeng Zhang et al. (2018) developed and synthesised pyrazolo[4,3-b]pyrimido[4,5-e]1,4 diazepines. Out of these, compound 67 had the highest level of effectiveness as a VEGFR-2 inhibitor, with a potency of 8.3 ± 4.7 nM. The morpholine derivative of compound 67 exhibited notable inhibitory effects on VEGFR-2, Aurora A, and Aurora B, with IC50 values of 21.6 nM, 46.2 nM, and 37.6 nM, respectively. The anti-proliferative efficacy of a morpholine derivative was assessed against various human gastric cancer cell lines, including SNU-5, MKN-45, MKN-74, SGC-7901, and BGC-823.153
Menna M.A. et al. (2021) synthesised a number of fluro[3,2-e][1,2,4]triazolo[1,5-c]pyrimidines and furo[2,3-d]pyrimidines and tested them for their ability to inhibit VEGFR-2 in a laboratory setting. These compounds showed effective inhibition in the nanomolar range, with some demonstrating enhanced ligand efficiencies. Compound 68 exhibited significant activity with an IC50 value of 38.72 ± 1.7 nM, while compound 69 showed substantial activity with an IC50 value of 41.40 ± 1.8 nM.
These values were compared to sorafenib, which had an IC50 value of 41.24 ± 1.9 nM. Compound 68 exhibited superior antiproliferative activity in the HUVEC cell assay, with an IC50 value of 17.37 ± 1.03 μM, compared to sorafenib, that had an IC50 value of 20.64 ± 1.22 μM. The docking investigation demonstrated that the compounds 68 and 69 exhibited a binding free energy of −8.00 kcal mol−1 and −7.58 kcal mol−1, respectively, at the active site of VEGFR-2 (Fig. 21).154
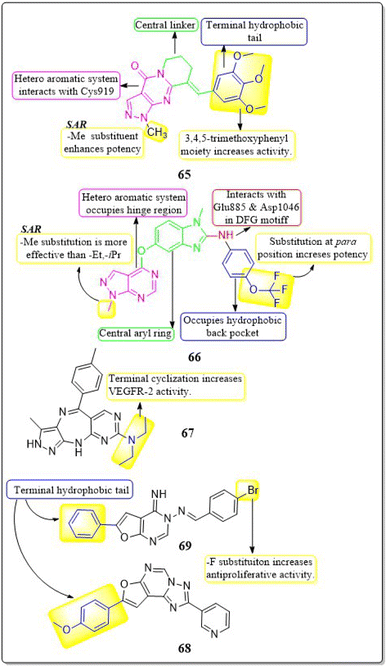 |
| Fig. 21 Pharmacophoric features and SAR of compounds 65 to 69. | |
4.10 Pyridine derivatives
Ahmed et al. (2021) synthesised a new anticancer pyridine-sulfonamide scaffold. Compound 70 was found to be an effective VEGFR-2 inhibitor (IC50 = 3.6 μM) compared to sorafenib (IC50 = 4.8 μM). Annexin V-FITC/PI experiments and DNA flow cytometry showed that hybrid 70 disrupted the renal UO-31 cell cycle and induced apoptosis. Compound 70, with a docking score of −27.09 kcal mol−1, strongly binds to the VEGFR-2 active site. The pyridine moiety interacted hydrophobically with Leu1033, Ala864, and Cys917. The terminal phenyl group made two hydrophobic connections with Cys1022 and Ile886. With Glu883 and Asp1044, the sulfonamide group created two hydrogen bonds. The phenyl (spacer) group interacted hydrophobically with Cys1043, Val914, Val89, Lys886, and Phe1045. The meta-fluro is active against all cell lines.155
A unique series of thiourea-azetidine hybrids was developed by Deepa R. Parmar et al. (2021) and evaluated against a range of human cancer cell lines. With EC50 values of 0.03, 0.25, 0.6, and 0.03 μM, respectively, compound 71 was shown to be the most effective member against 786-O, PC3, U251, and A431 cancer cell lines. It also demonstrated greater potency than doxorubicin in PC3, A431, and 786-O cell lines. The inhibitory action significantly increased upon the insertion of a methoxy group. 71's binding free energy (ΔG in kcal mol−1) has been estimated to be −21.12 kcal mol−1.156
In a recent study, Eslam B. Elkaeed et al. (2022) successfully synthesised and evaluated a series of nicotinamide-based derivatives. This compound, 72, showed impressive inhibitory potential against VEGFR-2 in laboratory tests, with a potency of 51 nM. It also displayed promising cytotoxicity against MCF-7 and HCT-116 cancer cell lines, with IC50 values of 8.25 μM and 6.48 μM, respectively. These results indicate a high level of selectivity, with selectivity indexes of 12.89 and 16.41 for the two cell lines. Through DFT studies, the binding mode of compound 72 with VEGFR-2 was confirmed. The MM-GBSA analysis further supported the proper binding, revealing a total binding energy of −38.36 kcal mol−1.157
Amal Abdel Haleem et al. (2020)158 performed the synthesis of molecules based on the 3-cyano-6-naphthylpyridine scaffold. These derivatives were designed to specifically block VEGFR-2. Compound 73 exhibited the highest potency compared to all other synthesised derivatives.
Delphinidin, a drug with well-established inhibitory effects on VEGFR-2, served as a reference drug. Compound 73 outperformed the reference standard, which is worth mentioning. Compound 73 effectively blocked the activity of VEGFR-2 in laboratory tests and computer simulations, with an IC50 value of 0.19 ± 0.01 nM and a binding energy score of −9.9868 kcal mol−1, while delphinidin showed VEGFR-2 inhibition at an IC50 value of 5.09 ± 0.42 nM with a docking score of −8.2655 kcal mol−1. Compound 73 exhibited superior inhibition of PC3, DU145, MCF-7, and MDA-MB435 cell lines compared to the reference standard Doxorubicin. The IC50 values for compound 73 were 55 ± 3.1 nM, 8.5 ± 0.43 nM, 0.5 ± 0.001 nM, and 96 ± 1.6 nM, respectively, while the IC50 values for doxorubicin were 59 ± 2.1 nM, 1.8 ± 0.01 nM, 11 ± 0.37 nM, and 69 ± 1.37 nM, respectively (Fig. 22).158
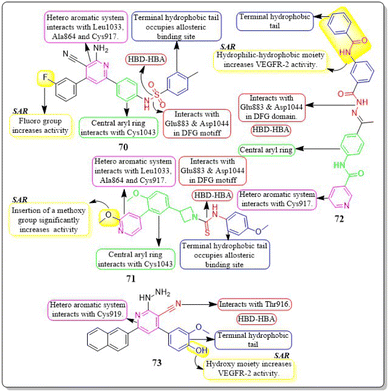 |
| Fig. 22 Pharmacophoric features and SAR of compounds 70 to 73. | |
4.11 Benzimidazole analogues
Islam H. Ali et al. (2023) studied 2-arylbenzimidazole-thiopyrimidine and -thioquinazolin-4(3H)-one conjugates. The benzimidazole-quinazolinone compounds have shown significant anticancer activity (GI50 = 1.3−4.2 μM) against tested cancer cell lines. In a VEGFR-2 kinase test, compound 74 had the highest potency at 6.14 μM. Any substitution on the 2-arylbenzimidazole nucleus diminishes activity, but the p-tolyl substitution on the third position, 4-oxo-3,4-dihydroquinazolin boosts it. Compounds 74 had a docking score of −14.82 kcal mol−1 at the VEGFR-2 binding site, compared to the native ligand sorafenib, which had a binding value of −15.19 kcal mol−1, reflecting their experimental inhibitory efficacy.159
An investigation conducted by Ayman Abo Elmaaty et al. (2023)160 showed the repurposing of thirteen FDA-approved benzimidazole anthelmintic medicines as VEGFR-2 antagonists. Based on the investigation involving molecular docking and molecular dynamic simulations against VEGFR-2, three benzimidazoles (fenbendazole 75, mebendazole 76, and albendazole 77) were identified as possible VEGFR-2 antagonists. Furthermore, these drugs demonstrated increased efficacy in inhibiting the growth of MCF7, A549, and HUH7.
In addition, to boost the solubility of mebendazole in water, it was synthesised as mixed micelles (MMs). These MMs exhibited improved drug release and demonstrated more promising cytotoxicity results in a cell-based VEGFR-2 assay compared to the unrefined mebendazole. Compounds 75–77 exhibited favourable interactions with VEGFR-2 in both docking and MD modelling tests. An ELISA was used to quantify VEGFR-2 in treated HUH7 cells; the results showed that all tested drugs significantly decreased the concentration of VEGFR-2. The greatest inhibition of VEGFR-2 was observed in MBZ-loaded MMs, with a concentration of 860.8 ± 312 pg mL−1. This was even better than the reference drug, sorafenib, which had a concentration of 1073 ± 41.1 pg mL−1.160
A group of researchers, led by Amany S. Mostafa et al. (2018), developed and evaluated a novel set of 2-phenylbenzimidazoles. Compound 78 exhibited the highest level of VEGFR-2 inhibitory activity (IC50 = 6.7 ± 1.3 nM) against the MCF7 cell line, surpassing the reference standard Sorafenib (IC50 = 7.6 ± 2.8 nM). Compound 78 demonstrated considerable inhibition of the MCF-10F, BJ, and MRC-5 cell lines, with IC50 values of 33.1 ± 1.8 μM, 40.6 ± 2.5 μM, and 17.3 ± 0.4 μM, respectively. This inhibition was comparable to that of the reference standard doxorubicin, which had IC50 values of 22.6 ± 2.7 μM, 17.2 ± 0.7 μM, and 15.2 ± 1.1 μM for the same cell lines, respectively. The presence of a nitro group on the fourth position of the terminal aryl ring enhances the activity of VEGFR-2.161
Xu Yuan et al. (2019) developed, synthesised, and tested a new family of benzimidazole compounds. Compound 79 exhibited the highest levels of inhibition towards VEGFR-2 kinase, HUVEC, and HepG2 cells, with IC50 values of 51.4 nM, 1.47 μM, and 2.57 μM, respectively. Compound 79 has shown significantly higher anti-angiogenic effects compared to sorafenib. The SAR investigations demonstrated that the addition of halogen atoms to the end of the phenyl group enhances the activity of VEGFR-2 (Fig. 23).162
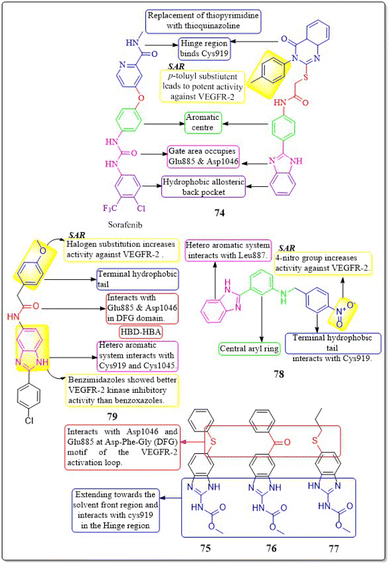 |
| Fig. 23 Pharmacophoric features and SAR of compounds 74 to 79. | |
4.12 Naphthalene derivatives
Em Cahn et al. (2022) synthesised a range of 2-naphthamide derivatives and assessed their antibacterial, antifungal, and anticancer properties in laboratory settings. Compound 80 demonstrated high inhibitory activity for VEGFR-2 in laboratory tests, with an IC50 value of 0.384 μM, while sorafenib had an IC50 value of 0.069 μM. The inclusion of N-(4-chlorobenzyl), 4-hydroxy, and 5,7-dimethoxy groups in the 2-naphthamide structure is preferable for increasing the effectiveness of the compound in fighting bacterial infections and tumours. In the docking simulation, compound 80 exhibited a binding affinity of −9.8 kcal mol−1 with VEGFR-2, which is greater than paclitaxel's affinity of −8.2 kcal mol−1 with VEGFR-2.163
M. Ihsan Han et al. (2021) came up with a new set of (S)-naproxen hydrazide-hydrazones that had strong inhibitory effects on VEGFR-2. Out of all, compound 81 was discovered to have the most effectiveness in inhibiting the growth of two types of human breast cancer cells (MDA-MB-231 and MCF-7). It also showed good selectivity, with IC50 values of 22.42 μM and 59.81 μM for each cell line, respectively. The incorporation of a trifluoromethoxy group at the second position enhances the activity but significantly diminishes it when added at the third position. The synthesised compound 81 showed a free energy (ΔG) of −9.77 kcal mol−1 when interacting with the active site of VEGFR-2. Compound 81 had potent antineoplastic effects and significantly reduced tumour size in mice with the Ehrlich acid tumour model, at both low (60 mg kg−1) and high (120 mg kg−1) doses.164
4.13 Pthalazine motifs
Fathalla Khedr et al. (2021) developed and synthesised derivatives of 4-phenylphthalazin-1-amine. Compound 82 was discovered to be the most powerful compound for inhibiting VEGFR-2, with an IC50 value of 0.11 ± 0.02 μM. This IC50 value is nearly equal to the value of sorafenib, which is 0.10 ± 0.02 μM. Compound 82 effectively suppressed the growth of three cancer cell lines, HepG2, HCT116, and MCF-7, with IC50 values of 11.23 ± 1.1 μM, 10.12 ± 1.0 μM, and 13.92 ± 1.2 μM, respectively. These results were superior to those of sorafenib, which had IC50 values of 9.18 ± 0.6 μM, 5.47 ± 0.3 μM, and 7.26 ± 0.3 μM, respectively. The estimated binding mode of compound 82 closely resembles that of sorafenib, which exhibited an affinity value of −101.98 kcal mol−1 and generated four hydrogen bonds. The binding energy of compound 82 is around −100.55 kcal mol−1.165
4.14 Benzothiophene analogues
Himalaya Singh et al. (2022) conducted experiments to assess the ability of cyanobenzo-thiophenes to suppress neovascularization in both ex vivo and in vivo angiogenic assays. Out of the compounds that were evaluated, derivative 83, which has a 4-hydroxyanilino substitution, demonstrated a high level of activity in inhibiting the formation of tubules and angiogenesis in HUVECs. This effect was observed at a concentration of 10 μM, ensuing in a complete halt of the process. The competitive binding experiment of compound 83 demonstrated its ability to prevent the phosphorylation of VEGFR2 induced by VEGF, resulting in the suppression of tubulogenesis. Furthermore, compound 83 effectively decreased the load in the xenograft model by suppressing the Akt/Src kinase activity and inducing reorganisation of the cytoskeleton in HUVECs (Fig. 24).166
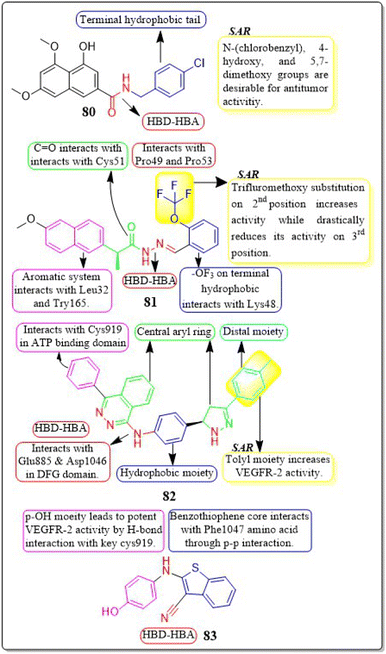 |
| Fig. 24 Pharmacophoric features and SAR of compounds 80 to 83. | |
4.15 Benzothiazole derivatives
Sahar M. Abou-Seri et al. (2021) introduced a new family of hydrazones based on isoxazole. Compound 84 showed great inhibition for VEGFR-2, with an IC50 value of 25.7 nM. This is slightly more effective than sorafenib, which had an IC50 value of 28.1 nM. The synthesised compounds were evaluated for their growth-inhibitory action against HepG2 cells. Compound 84 has shown superior efficacy with an IC50 value of 0.84 μM, surpassing the reference drug sorafenib with an IC50 value of 3.99 μM. The binding free energy for compound 84 at the VEGFR-2 binding site has been estimated to be −8.17 kcal mol−1. Compound 84's urea linker engages in H-bonding interactions with the crucial amino acids Glu-885 and Asp1046.167
Velma Ganga Reddy et al. (2019)168 performed the derivatization of pyrazolo-benzothiazole hybrids. Out of the compounds that were evaluated, compound 85 demonstrated noteworthy suppression of VEGFR-2 with an IC50 value of 97 nM. Compound 85 exhibits high potency against all examined cancer cell lines, with an IC50 in the range 3.17 μM to 6.77 μM. It performs even better than the reference medication axitinib, which has an IC50 in the range of 4.88 μM to 21.7 μM.
Hybrid molecule 85 exhibited the highest level of activity compared to the other compounds in the series. It displayed IC50 values of 3.17 μM (PC-3), 3.32 μM (HT-29), 3.87 μM (A549), and 6.77 μM (U87MG). Furthermore, it demonstrated greater activity than axitinib, which is a drug already used in clinical practice. Furthermore, compound 85 exhibited a selectivity towards cancer cells that was 9 to 15 times greater than that of axitinib. This clearly demonstrates the compound's high level of selectivity towards cancer cells. Compound 85 exhibited potent anti-angiogenic properties by effectively suppressing the development of intersegmental vessels in transgenic zebrafish.168
4.16 Benzodiazepines motifs
A new series of diazepam compounds with sulfonamide groups was synthesised and tested for their potential as anticancer activity by Nashwa M. Saleh et al. (2020). Compound 86 stood out as the most powerful derivative in its ability to inhibit VEGFR-2 at a concentration of (IC50 = 0.10 ± 0.01 μM), which is just as effective as sorafenib (IC50 = 0.10 ± 0.02 μM). It's worth noting that compound 86 demonstrated significant potency for the HepG2, HCT116, and MCF-7 cancer cell lines (IC50 = 8.98 ± 0.1, 7.77 ± 0.1, and 6.99 ± 0.1 μM, respectively). Compound 86 showed greater activity compared to sorafenib against HepG2 and MCF-7 cancer cell lines, with IC50 values of 9.18 ± 0.6 μM, 5.47 ± 0.3 μM, and 7.26 ± 0.3 μM, respectively. However, its activity against the HCT116 cell line was lower. Compound 86 was successfully docked at the ATP-binding site of the VEGFR-2 kinase enzyme, yielding a docking energy score of −116.78 kcal mol−1. The presence of the 4,6-dimethylpyrimidine moiety enhances its efficacy against VEGFR-2.169
4.17 Benzoxazole derivatives
Alaa Elwan and co-workers (2022) developed and synthesised benzoxazole-based compounds with the purpose of evaluating their effectiveness in inhibiting VEGFR-2 kinase and their potential as anticancer agents. Compound 87 had superior performance compared to sorafenib in both VEGFR-2 inhibition and anti-proliferative experiments, making it the most promising contender. The IC50 values for MCF-7, HCT116, HepG2 cell lines, and VEGFR-2 kinase were 3.43, 2.79, 2.43, and 0.0554 μM, respectively. However, the IC50 values of sorafenib were 4.21, 5.30, 3.40, and 0.0782 μM, respectively. Compound 87 demonstrated substantial suppression of TNF-a (90.54%) and IL-6 (92.19%) in comparison to dexamethasone (93.15%). The synthesised compound 87 exhibited a docking binding free energy of −7.65 kcal mol−1 against the VEGFR-2 active site (Fig. 25).170
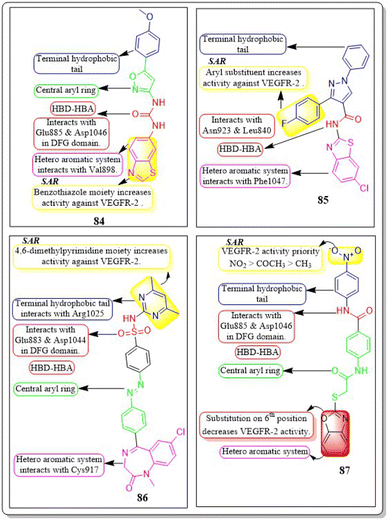 |
| Fig. 25 Pharmacophoric features and SAR of compounds 84 to 87. | |
4.18 Benzofuran analogues
A novel series of benzofuran derivatives was designed, synthesised, and evaluated by Omar A. El-Khouly et al. (2022). Compound 88 demonstrated potent inhibition of VEGFR-2, with an IC50 value of 68 nM. Compound 88 exhibited superior activity compared to other compounds against HePG2, MCF-7, HeLa, and PC3 cell lines, with IC50 values of 9.73 ± 0.7 μM, 11.58 ± 0.9 μM, 7.94 ± 0.5 μM, and 17.49 ± 1.3 μM respectively. It was discovered that the compound 88's docking score at the active binding site of VEGFR-2 was −7.0 kcal mol−1.171
4.19 Benzoquinone derivatives
Hayamitsu Adachi et al. (2021) developed vegfrecine a-pnalogues and assessed their effectiveness against VEGFR-2. Compound 89 was found to be a more powerful inhibitor than that of vegfrecine against the VEGFR-2 tyrosine kinase. Compound 89 demonstrated greater specificity for the VEGFR-2 kinase compared to VEGFR-1. The addition of halo- and alkoxy-substituents at the 5-position of the phenyl ring led to strong inhibition of the VEGFR-2 tyrosine kinases.172
4.20 Coumarin hybrids
In their study, Tahia K. Mohamed et al. (2021) successfully developed and synthesized novel derivatives of thiazolopyrazolyl coumarin. Compound 90 exhibited remarkable potency against VEGFR-2, with an IC50 value of 34 nM. Compound 90 exhibited remarkable cytotoxic activity for MCF-7, with an IC50 value of 5.41 μM, outperforming the reference drug doxorubicin (IC50 = 6.73 μM). The presence of the 4-chlorophenyldiazenyl moiety leads to increased VEGFR-2 activity. The binding pattern of compound 90 was enhanced by the hydrophobic interactions between the three moieties (p-chlorophenyl, phenyl, and dimethylaminophenyl) and the hydrophobic residues. This was explained by compound 90's superior docking score (−11.10 kcal mol−1) at the active site of VEGFR-2.173
A set of 3-thiazolyl-coumarins was modified and assessed by Tariq Z. Abolibda et al. (2023). Among all the compounds tested, 91 showed significant potential for inhibiting cancer growth in MCF-7 cells, with an IC50 value of 11.2 ± 0.80 μM. The molecular docking studies of the resulting derivatives were evaluated against VEGFR-2 and exhibited activities similar to sorafenib's, with compound 91 displaying the best binding score (−9.900 kcal mol−1). Substituting the solvent-accessible terminal phenyl ring leads to a decrease in VEGFR-2 activity (Fig. 26).174
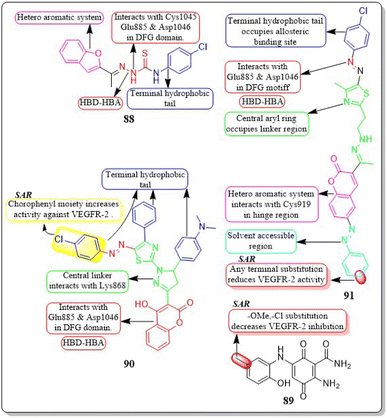 |
| Fig. 26 Pharmacophoric features and SAR of compounds 88 to 91. | |
4.21 Miscellaneous
4.21.1 Furan analogues. Mohamed H. Hekal and his co-workers (2021) developed a collection of new compounds, N-(1,3,4-thiadiazol-2-yl)furan-2-carboxamide derivatives. Compounds 92 and 93 exhibited the most advantageous orientation to VEGFR-2 based on the docking experiments. Additionally, they were the most effective inhibitors of the receptor, with IC50 values of 7.4 ± 0.8 nM and 7.6 ± 0.4 nM, respectively. The antiproliferative properties were assessed towards three human epithelial cell lines: breast (MCF-7), colon (HCT-116), and prostate (PC-3) using the MTT assay technique employing doxorubcin as a reference standard. The free energy of binding for compounds 92 and 93 at the VEGFR-2 binding site has been estimated to be −55.90 kcal mol−1 and −67.65 kcal mol−1, respectively. The presence of the carbonyl group in the carboxamide moiety is considered essential for the binding process and plays a role in enhancing the affinity of compound 92.175
4.21.2 Pyrazole derivatives. Fa-Qian Shen et al. (2019) synthesised a group of benzoyl amide compounds that contain a nitrogen heterocyclic ring. Compound 94 showed greater inhibition towards VEGFR-2, HeLa, A549, MCF-7, and HepG-2, with IC50 values of 0.34 ± 0.02 μM, 4.57 ± 0.30 μM, 15.57 ± 1.10 μM, 1.08 ± 0.06 μM, and 2.44 ± 0.15 μM, respectively. Substituting the chloro group with hydrogen on the phenyl ring that is connected to sulphonamide yielded a substantial decline in activity. The substitution of a toluyl group with a methyl group on the imidazole ring results in a comparable decrease in efficacy.176
4.21.3 Thiazolidinedione hybrids. In a recent study, Neha Upadhyay and co-workers (2021) developed a new set of diarylpyrazoline-thiazolidinediones and conducted both in vitro and in vivo assays to evaluate their biological properties. The results demonstrated that compound 95 showed great inhibition, with an IC50 value of 5 μM against VEGFR-2. When an electron-donating group is placed to the ortho-position of the pyrazole ring, it enhances the activity. The anti-angiogenic potential of 95 was clearly demonstrated through various assays, including HUVEC proliferation, migration, and tube formation. The in vivo assay showed a significant potency of 95 in reducing neovascularization in the developing CAM. Compound 95 exhibited superior scores on the active site of VEGFR-2 compared to its steric counterparts.177In a new study, Khaled El-Adl et al. (2020) developed a set of thiazolidine-2,4-diones and tested their effectiveness on HepG2, HCT-116, and MCF-7 cells. Compound 96 exhibits similar activities to sorafenib against HepG2 cells, with IC50 values of 9.18 ± 0.6, 5.47 ± 0.3, and 7.26 ± 0.3 μM, respectively. However, it shows lower inhibition for HCT-116 cells and slightly higher inhibition for MCF-7. Compound 96 showed strong inhibition of VEGFR-2, with IC50 values of 0.17 ± 0.02 μ. Compounds containing the 2,4-dichlorobenzylidene moiety demonstrated greater VEGFR-2 inhibition activities compared to those containing the 4-chlorobenzylidene moiety. The distal phenyl group, which had ethyl ester substitutions in either 2,4-dichlorobenzylidene or 4-chlorobenzylidene derivatives, showed the most potent activities with an IC50 value of 0.17 ± 0.02 μM. Compounds containing a distal phenyl group, like 96, exhibited greater activity compared to those with a distal aliphatic group. The binding modes of compounds 96 closely resemble those of sorafenib, with affinity values of −101.17 kcal mol−1 and −101.14 kcal mol−1, forming 5 and 6 H-bonds, respectively (Fig. 27).178
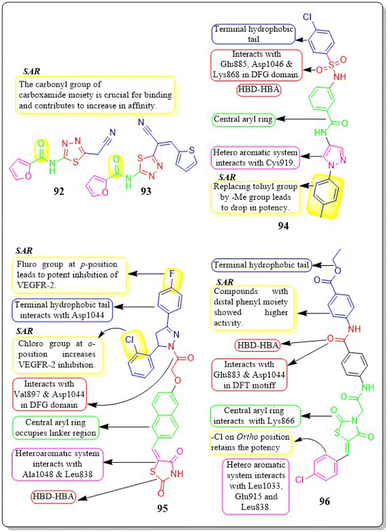 |
| Fig. 27 Pharmacophoric features and SAR of compounds 92 to 96. | |
4.21.4 2-Thioxoimidazolidin-4-ones derivatives. A novel series of 2-thioxoimidazolidin-4-ones was conceived and developed by Ahmed A.E. Mourad et al. (2021). Compounds 97 and 98 demonstrated higher inhibitory activity against VEGFR-2 (IC50 = 25.14 ± 1.9 nM and 19.78 ± 1.3 nM, respectively) compared to sorafenib (IC50 = 35.62 ± 2.2 nM). Compounds 97 and 98 exhibited superior inhibition compared to sorafenib and erlotinib towards the MCF-7, HepG2, and A549 cell lines. The inhibitory activity of compounds 97 (1.63 ± 0.03 μM, 2.44 ± 0.13 μM, and 1.27 ± 0.04 μM, respectively) and 98 (2.26 ± 0.12 μM, 5.18 ± 0.23 μM, 3.14 ± 0.15 μM, respectively) was found to be greater than that of sorafenib and erlotinib.179
4.21.5 1,2,4-Triazole analogues. Mohammed K. Abdelhameida et al. (2020) developed a set of new azole compounds that were synthesized and tested for their effectiveness against tumours. Compound 99 exhibited greater activity than the other compounds in terms of VEGFR-2 expression, β-TUB polymerization, and inhibition of the HepG2 cell line (IC50 = 19.82 ± 1.72 nM, 88.74 ± 9.27 μM, and 0.24 ± 0.06 μM, correspondingly). Compound 99 exhibited two hydrogen bond interactions with the amino acid Lys868, as well as a hydrophobic interaction.180
4.21.6 1,3,4-Thiadiazole motifs. A study conducted by Saad R. Atta-Allah et al. (2021) involved the synthesis and bio-evaluation of 1,3,4-thiadiazols. Compound 100 exhibited remarkable inhibitory activity against VEGFR-2, with an IC50 value of 8.2 nM, surpassing the potency of pazopanib (IC50 = 9.7 nM). The cytotoxic activity of compound 100 against HepG-2, MCF-7, HCT-116, and PC-3 cancer cell lines showed promising results (4.22 ± 0.94 μM, 8.45 ± 0.75 μM, 33.14 ± 6.52 μM, 7.76 ± 0.6 μM) when compared to the reference standard pazopanib. The ligand's binding mode displayed an energy of −9.363 kcal mol−1. Derivative 100 exhibited three hydrogen bond interactions with Glu 885 and Asp 1046, along with a hydrophobic interaction with Lys 868.181
4.21.7 N-Acylhydrazone derivatives. In their study, Fernanda P. Pauli et al. (2020) examined a modified N-acylhydrazone structure and evaluated its effectiveness in inhibiting VEGFR-2 activity. The derivative 101, which has a trifluoromethyl substituent on the para position of the phenyl group, successfully suppressed neovascularization caused by VEGF in the CAM experiment. The tube generation experiment conducted on HUVECs demonstrated a beneficial impact of compound 101 on the production of new blood vessels (neovascularization) (Fig. 28).182
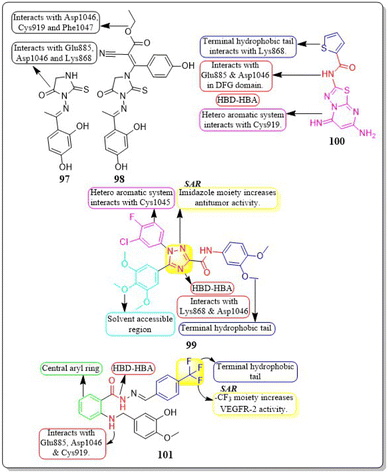 |
| Fig. 28 Pharmacophoric features and SAR of compounds 97 to 101. | |
5. Recent patents
5.1 WO2023040996A1
This patent explores an azaindazole macrocyclic compound about its effect of inhibiting the activity of a plurality of protein kinases, including HPK1, FLT3, and KDR (VEGFR-2). Compound 102 displayed inhibitory activity obtained using the four-coefficient nonlinear fitting formula with an IC50 around ≤5 nM against VEGFR-2 along with HPK1 and FLT3. Compound 102 also surpassed the MV-4-11 cell viability test with an EC50 of 3.8 nM, calculated by GraphPad Prism 5.0 software (Fig. 29).183
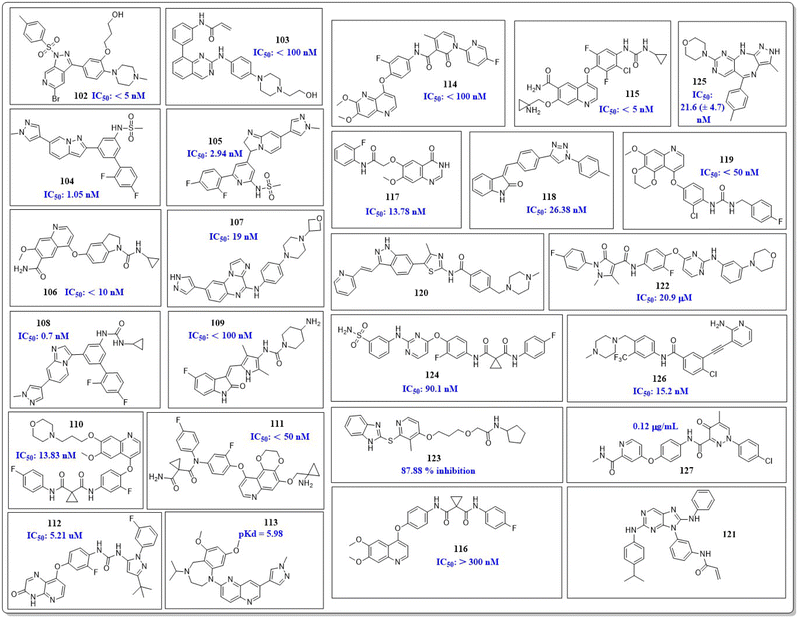 |
| Fig. 29 Compounds 102 to 127 with their VEGFR-2 activity from recent patents. | |
5.2 US20220354864A1
This patent contains essential information regarding substituted quinazolines for inhibiting kinase activity against EGFR, EGFR mutants, FGFR1 and 2, BTK, KDR (VEGFR-2), and KAK3. Compound 103 showed VEGFR-2 inhibition at an IC50 lower than 100 nM. Compound 103 significantly inhibited cancer cell lines: A549, A431, H1299, HCC827, H3255, and H1975.184
5.3 US20220315581A1
The current patent provides an explanation for fused ring compounds as FGFR and VEGFR dual inhibitors. Compound 104 exhibited excellent inhibitory activity of IC50 of 1.05 nM, 2.86 nM, and 2.66 nM against VEGFR-2, FGFR-1, and FGFR-2, respectively. IC50 data was obtained by parametric curve fitting (GraphPad Software). The compound 104 displayed a significant inhibitory effect on cell proliferation in the SNU-16 cell activity test with an IC50 of 14.5 nM. Upon a pharmacokinetic study, it was found that compound 104 can quickly reach a peak and exhibit a high oral absorption bioavailability of 73.2% after oral administration.185
5.4 US20220267324A1
This innovation discusses pyridine derivatives as FGFR and VEGFR dual inhibitors. The compound 105 demonstrated strong VEGFR-2 and FGFR-2 inhibition with IC50 = 2.94 nM and 3.22 nM, respectively. The pharmacokinetic activity revealed that the oral absorption bioavailability of compound 105 is 59.3%. The compound 105 shows significant anti-tumour activity of 69% against the SNU-16 cell line.186
5.5 US20210188806A1
This patent encompasses the process of preparing and the medical applications of the indoline-1-formamide substance. Compound 106 demonstrated potent inhibition of VEGFR-1, VEGFR-2, and VEGFR-3, with IC50 values below 10 nM and from 10 nM to 100 nM. The IC50 value of the substance is determined using the XLfit software (ID Business Solutions Ltd., UK) based on 8 concentration points.187
5.6 KR20220130747A
The patent informs in detail about 1H-pyrazole derivatives and their uses as Syk and VEGFR-2 dual target inhibitors. Compound 107 inhibited VEGFR-2 and Syk with IC50 = 19 nM and 17 nM, respectively. The compound has a high ocular blood ratio and is suitable for ocular administration. Compound 107 showed statistically significant drug efficacy in the scopolamine-induced mouse dry eye model.188
5.7 CN113490667A
This patent contains information about imidazopyridine derivatives as dual FGFR and VEGFR inhibitors. Compound 108 inhibited VEGFR-2 with IC50 = 0.7 nM. The compound 108 displayed more excellent SNU-16 cell activity (3–5-fold) than the control with IC50 = 10 nM. The compound of the present invention shows excellent tumour treatment effects at a lower dose in preclinical animal models.189
5.8 JP2021535931A
The pyrrole-substituted analogues were synthesized and tested for their potential for inhibitory activity against KDR (VEGFR-2), FLT3, and its mutants. Compound 109 is FLT3, FLT3-ITD, showed strong inhibitory activity against all FLT3D835Y, PDGFRβ, c-Kit, RET, KDR, showed certain inhibitory activity against AXL, etc., IC50 >100 nM, which was a selective FLT3 inhibitor.190
5.9 WO2018059022A1
The patent has explored the biological activities of multiple signal transduction kinases, such as C-MET and KDR. Compound 110 inhibits VEGFR-2 and C-MET (IC50 = 1.83 nM and 4.4 nM, respectively). The compound 110 successfully inhibited HCC78, U87MG, HUVEC, and MKN-45 cell lines (IC50 = 0.157 μM, 0.436 μM, 0.292 μM, and 1.015 μM). Compound 110 similarly inhibited SK-OV-3, HCT-116, and A549 cell lines with IC50 = 4.326 μM, 0.786 μM, and 1.881 μM, respectively.191
5.10 EP3750893B1
The patent reveals information about the dioxazoline compound and its potential for inhibiting VEGFR-2 and C-MET. The compound 111 inhibits the C-MET, VEGFR-2, and MHCC97H cell lines with an IC50 <50 nM.192
5.11 US20220135544A1
This invention pertains generally to the capability of pyridopyrazinone derivatives and their potential for inhibitory activity against multiple tyrosine kinases such as KDR, MET, CRAF, EGFR, PDGFR-α, PDGFR-β, FGFR-1, and Src. Compound 112 inhibited all those kinases involved in resistance to BRAF inhibitors. The VEGFR-2 inhibition of compound 112 was moderate, with IC50 = 0.12 μM.193
5.12 US011542247B2
This patent demonstrates that compounds of 1,4-benzodiazepines have the capability to inhibit FGFR domains and VEGFR-2. Compound 113 exhibited inhibitory effects on VEGFR-2 and FGFR-1, FGFR-2, FGFR-3, and FGFR-4 in an enzyme binding experiment conducted using KINOMEscan®. The compound 113 demonstrated pKd values of 5.98 and 6.85, 6.03, 6.53, and 6.72 for each respective target.194
5.13 US20230151003A1
This patent has explored the potential of naphthyridine derivatives against C-MET, Mer, KDR, and Axl. The compound 114 successfully inhibited all these kinases with an IC50 < 100 nM.195
5.14 US20230124784A1
This patent deals with the potential of quinoline derivatives against different kinases such as VEGFR-1, VEGFR-2, VEGFR-3, FGFR-1, and RET. Compound 115 inhibited VEGFR-1/2/3 and RET with IC50 <5 nM. Along with VEGFR kinases, compound 115 also inhibited FGFR-2 with an IC50 <50 nM. The cell line studies revealed that compound 115 inhibited the following cell lines BXPC3 (IC50 <5 μM), A549 (<2.5 μM), Caki-1 (<2.5 μM), Hep3B2.1–7 (<2.5 μM), SUN16 (<5 μM), HeLa (<5 μM), k562 (<5 μM), PC-3 (5–10 μM), and hERG (>30 μM) cells.196
5.15 WO2020154610A
Compound 116 is one of a series of substituted quinoline compounds that the patent describes as kinase inhibitors that target many kinases, including Axl, Mer, c-Met, and VEGFR-2. The IC50 values for the compounds in this disclosure fell within the following ranges: Axl's IC50 is less than 10 nM, Mer's is between 10 and 100 nM, c-Met's is between 100 and 300 nM, and KDR's (VEGFR-2) is greater than 300 nM. The outcomes of a test to determine the metabolic stability of liver microsome tissues from humans, mice, rats, and dogs were also provided.197
5.16 CN110903253A
Compound 117, derived from chalcones, has been developed as a multi-target receptor tyrosine kinase inhibitor in this invention. Compound 117 displayed greater inhibitory activity against EGFR, VEGFR-2, and FGFR1 kinases, with IC50 values of 8.35 nM, 13.78 nM, and 18.42 nM, respectively. Compound 117 demonstrated inhibitory effects on cell lines including MCF-7, A549, and K562, with IC50 values of 9.27 μM, 9.89 μM, and 7.61 μM, respectively.198
5.17 CN111153889A
This invention presents a range of 2-indolone-triazole compounds that have demonstrated potential as antitumour agents. Compound 118 demonstrated significant inhibition of human VEGFR-2 kinase and H460 cell lines, with an IC50 value of 26.38 nM compared to 223.0 nM for the reference drug sunitinib. It also effectively suppressed the proliferation of the human lung cancer H460 cell line, with an IC50 value of 1.02 μM in contrast to 3.65 μM for sunitinib.199
5.18 WO2020042972A1
This invention details the synthesis of dioxane and quinazoline or quinoline compounds that are connected to a urea-substituted aromatic ring, serving as inhibitors for VEGFR-2 and CSF1R. Compound 119 demonstrated potent inhibitory activity against VEGFR-2 kinase and M-NFS-60 cell proliferation, with IC50 values below 50 nM and 100 nM, respectively.200
5.19 CN111138426A
This patent describes a group of indazole compounds that act as inhibitors of kinases. These compounds can be used to prevent or treat disorders that are dependent on kinase activity, such as gastrointestinal tumours. Compound 120 had the highest level of effectiveness among the synthesized compounds in suppressing the activity of c-KIT, FLT3, PDGFR-α, PDGFR-β, and/or VEGFR2 kinases.201
5.20 CN109384788A
Purine derivatives manufacture and use as anticancer agents were revealed in this invention. The synthesised compounds showed potent inhibitory actions that target VEGFR2 and EGFR. Compound 121 among the examined substances reduced the development of the HCC827 and H1975 cell lines with IC50 values under 100 nM.202
5.21 CN107286140A
This patent reveals information about cabozantinib derivatives and their activity against multiple kinases. Compound 122 inhibited C-MET, EGFR, and VEGFR-2 with IC50 = 7.44 nM, 0.81 nM, and 20.9 μM, respectively as compared to that if cabozantinib's IC50 of 60.9 nM, 74.8 μM, and 3.74 nM, respectively. Upon cell line investigation, it was observed that the compound without terminal fluoro substitution exhibited better activity against HCT-116, Caki-1, HepG2, PC-3, PANC-1, and MRC-5 cell lines with IC50 = 1.37 μM, 0.53 μM, 1.34 μM, 1.33 μM, 1.00 μM, and 281.7 μM.203
5.22 CN110003176A
This patent includes details about benzimidazole derivatives and their inhibitory potential against different kinases, such as BRaf, VEGFR-2, PDGFR-β, and TOPK. The compound 123 inhibited BRaf, VEGFR-2, and PDGFR-β with significant inhibition of 90.96%, 87.88%, and 88.67%, respectively. Further cell line studies against A549, HCT116, and PC-3 cell lines disclosed that the most potent compound displayed better inhibitory activity (IC50 = 1.14 μM, 1.67 μM, and 2.64 μM) than reference standard, sorafenib (IC50 = 2.12 μM, 2.25 μM, 3.60 μM).204
5.23 CN110386901A
This patent discloses information about pyrimidine-sulphonilamide hybrids and their ability to inhibit multiple kinases. Compound 124 successfully inhibited c-Met, VEGFR-2, and EGFR with IC50 = 4.32 nM, 6.91 nM, and 58.6 nM, respectively as compared to reference standard cabozantinib (IC50 = 61.2 nM, 74.5 nM, and 3575 nM). The compound 124 also inhibited HCT-116, Caki-1, HepG2, PC-3, PANC-1, and hERG cell lines with IC50 = 2.09 μM, 0.86 μM, 6.98 μM, 0.86 μM, 7.61, μM and >30 μM, respectively, better than reference standard Cabozantinib (4.32 μM, 6.26 μM, 11.39 μM, 7.54 μM, 5.50 μM, 28.2 μM respectively).205
5.24 CN109020980A
Pyrazolo-pyrimido-diazepine derivatives were explored as Aurora and VEGFR-2 kinase inhibitors in this patent. The compound 125 inhibited aurora-A/B and KDR with IC50 = 46.2 ± 2.2 nM, 37.6 ± 13.3 nM, and 21.6 ± 4.7 nM. Cell line studies revealed that compound 125 inhibited MKN-45, MKN-74, SGC-7901, BGC-823 cell lines with IC50 = 1255.42 ± 558.98 nM, 3137.86 ± 408.39 nM, 10 μM, and 10 μM, respectively.206
5.25 CN108456163A
This patent discovers about piperazine derivates and their potential for inhibitory action against FGFR1, RET, and KDR. Compound 126 displayed inhibitory activity against FGFR1, RET, and KDR with IC50 = 45.1 nM, 40.1 nM, and 15.2 nM, respectively.207
5.26 CN109096250A
The invention investigated the inhibitory potential of 4-phenoxypyridine-pyridazinone derivatives on VEGFR-2. The compound 127 had a VEGFR-2 inhibitory activity (IC50) of 0.12 μg mL−1, making it the most powerful derivative. The compound 127 showed stronger inhibitory effects on the BGC-823, MKN-45, H460, HT-29, and A549 cell lines compared to the reference standard sorafenib. The IC50 values (in μg mL−1) for compound 127 were 0.82, 1.29, 2.49, 3.54, and 0.37, respectively, while the IC50 values (in μg mL−1) for sorafenib were 1.51, 1.43, 1.73, 2.01, and 1.25, respectively.208
6. Essentials for developing VEGFR-2 inhibitor
The VEGFR-2 kinase has been extensively investigated as a cancer target over the past decade, with a wide variety of distinct structural motifs. Although many compounds have been synthesized to block VEGFR-2 at micromolar concentration, there is still potential for the development of more powerful molecules. While gathering data for this review, we noticed a consistent trend in the molecules. Heterocyclic nuclei that contain nitrogen as a heteroatom are commonly observed to have a higher prevalence in inhibiting VEGFR-2. The Asp-Phe-Gly (DFG) motif plays an important role in the regulation of VEGFR-2 and most of kinase activity. The solvent accessibility of the molecule is enhanced by further substitution on the heterocyclic nucleus utilizing methyl/ethyl ester, nitro, and 1-(ethoxymethyl)cyclopropan-1-amine group. The core aromatic ring connected to a heterocyclic aromatic ring enhances the Pi–Pi interaction of the molecule. The pharmacophore typically consists of an amide linkage, which acts as a hydrogen-bond acceptor and donor group. Its bio-isosteres are typically connected adjacent to the core aromatic ring. The terminal hydrophobic tail of the molecule often consists of an aromatic ring with various replacements, such as halogens, ether, nitro groups, and so on.
Quinazolines have consistently outperformed the reference standard sorafenib in inhibiting VEGFR-2 during the experiment. The quinoxalines, indole, thiazolidinediones, and benzothiazoles analogues are heterocyclic aromatic systems that exhibited superior inhibition of the VEGFR-2 enzyme compared to the reference drugs. The compounds that showed considerable activity against VEGFR-2 included a terminal hydrophobic tail portion consisting of methoxyphenyl, trimethoxyphenyl, trifluromethyl halogen/alkyl/nitro modified aromatic moieties. The core aryl linker, which connects heteroaromatic systems and HBD–HBA, consists of an aromatic ring (ideally benzene) and an amide bond, with an optimal spacing of three to five atoms. Molecules bearing the amide and cyclopropane-1,1-dicarboxamide linkage, acting as hydrogen bond donor–hydrogen bond acceptor, have been found to be more effective VEGFR-2 inhibitors compared to those with sulphonamide or thioamide linkages. Although most effective compounds follow a general pattern, there are notable exceptions, such as fused molecules (PROTAC degraders), that show strong VEGFR-2 inhibitory action.
7 Conclusions
While the fight against cancer is ongoing, the inhibition of angiogenesis has become a viable approach for cancer treatment with the identification of new genes, transcription factors, signalling pathways, and mechanisms linked to it. VEGF and VEGFRs are the key component in controlling angiogenesis. As cancer progresses, VEGF has been found to be widely distributed and overexpressed. When VEGF binds to the protein kinase VEGFR-2, it triggers the production of blood capillaries and mediates the signalling pathway. Blocking VEGFR-2 signalling is therefore seen to be one of the most promising ways to prevent tumour-induced angiogenesis. Although many drugs targeting VEGFR-2 has been approved by USFDA for the treatment various cancers; they evolved with their own set of side effects and drug resistance over the course of treatment and hence there is still an urgent need of more effective anticancer molecules.
This review focuses on the structure of VEGFR-2, physiological role, and involvement of VEGF/VEGFR-2 system in the onset and progress of cancer. The design and structure–activity relationship of small-molecule VEGFR-2 inhibitors published through papers and patents in last five years is discussed that have shown improved anticancer attributes in recent years. The efforts taken by researchers to enhance the potencies of the molecules towards inhibiting VEGFR-2 in comparison to reference standard drugs such as sorafenib, sunitinib, or pazopanib is focused. The structural framework features requisite for developing new drug candidate are well explored in the present paper. The Asp-Phe-Gly (DFG) motif plays an important role in the regulation of VEGFR-2 and most of kinase activity. Heteroaromatic system is required for the interaction with crucial amino acid residues such as Cys919, Asp1046, Asn921, and/or Glu885 within the ATP-binding domain of VEGFR-2. The pharmacophoric element in majority ligands typically consists of an amide linkage, which acts as an HBD–HBA region interacts with Glu885 and Asp1046 in DFG domain of target receptor. Central aryl ring is necessary for occupying linker region and variety of bio-isostere could enhance the VEGFR-2 inhibition. Solvent-accessible region of the ligand molecule interacts with Asn923. Terminal hydrophobic tail consisting of an aromatic ring with variable substitutions occupies allosteric binding site. Five bond spacers between heteroaromatic region and HBD–HBA region in the molecule has always provided better result towards VEGFR-2 inhibition mirroring the behaviours of sorafenib or reference standard. Although most effective compounds follow a general pattern, there are notable exceptions, such as fused molecules (PROTAC degraders), that show strong VEGFR-2 inhibitory action.
This paper will help prospective synthetic and medicinal chemists to explore VEGFR-2 inhibitors utilising a molecular or pharmacophore hybridization approach. Combining different molecular fragments and pharmacophoric features, researchers can enhance the potency, selectivity, and therapeutic efficacy of novel hybrid molecules against VEGFR-2 targeting various cancers. This strategy will allow the generations of a library of compounds with diverse chemical structures and properties, offering a wide range of options for optimizing anticancer or antiangiogenic drug candidates and overcoming resistance mechanisms.
Abbreviations
VEGFR | Vascular Endothelial Growth Factor Receptor |
KDR | Kinase Insert Domain Receptor |
FLK-1 | Foetal-Liver Kinase 1 |
HBD–HBA | Hydrogen Bond Donor–Hydrogen Bond Acceptor |
MM-PBSA | Molecular Mechanics Poisson–Boltzmann Surface Area |
MMGBSA | Molecular Mechanics Generalized-Born Surface Area |
MD | Molecular Dynamic |
DFG | d-Asp-Phe-Gly Motif |
HUVEC | Human Umbilical Vein Endothelial Cells |
bFGF/FGF2 | Basic Fibroblast Growth Factor |
CAM | Chorioallantoic Membrane |
VHL | Von Hippel–Lindau |
PROTAC | Proteolysis Targeting Chimera |
PLC/PKC pathway | Phospholipase C/Protein Kinase C Pathway |
Syk | Splenic Tyrosine Kinase |
TIE2 | Tyrosine Kinase with Immunoglobulin and Epidermal Growth Factor Homology Domains 2 |
USFDA | United States Food and Drug Administration |
TK | Tyrosine Kinase |
eNOS | Endothelial Nitric Oxide Synthase |
PI3K | Phosphoinositide 3-Kinase |
MAPK/ERK | Mitogen Activated Protein Kinase/Extracellular-Signal-Regulated Kinase |
NCI | National Cancer Institute |
TGI | Tumour Growth Inhibition |
ELISA | Enzyme-Linked Immunosorbent Assay |
SAR | Structure–Activity Relationship |
p38MAPK | p38 Mitogen-Activated Protein Kinases |
PI3K/PBK-Akt | Phosphoinositide-3-Kinase/Protein Kinase B-Akt |
PLCγ | Phospholipase C Gamma |
FLK | Focal Adhesion Kinase |
PlGF | Placental Growth Factor |
Src | Sarcoma Oncoprotein |
Shc | Src Homology 2 |
Akt | Serine-Threonine Protein Kinase |
IL-8 | Interleukin 8 |
IL-6 | Interleukin 6 |
Ras | Rat sarcoma (guanosine-nucleotide-binding protein) |
Raf | Rapidly Accelerated Fibrosarcom |
MEK1 | MAPK/ERK Kinase |
Erk1 | Extracellular Signal-Regulated Kinase 1 |
PKB | Protein Kinase B |
vWF | von Willebrand factor |
BAD | BCL2 Associated agonist of Cell Death |
DNA | Deoxyribonucleic Acid |
V-FITC/PI | V-fluorescein Isothiocyanate (FITC)/Propidium Iodide (PI) |
VHL-L | von Hippel–Lindau-like protein |
mTOR | Mammalian Target of Rapamycin |
β-TUB | β-Tubulin |
TNF-α | Tumour Necrosis Factor Alpha |
HPK1 | Hematopoietic Progenitor Kinase |
hERG | human Ether-a-go-go-Related Gene |
FLT3 | FMS-like Tyrosine Kinase 3 |
FLT3-ITD | FMS-like Tyrosine Kinase 3-Internal Tandem Duplication |
FGFR-1 | Fibroblast Growth Factor Receptor 1 |
C-MET | mesenchymalepithelial transition factor |
EGFR | Epidermal growth factor receptor |
PDGFR-α | Platelet-Derived Growth Factor Receptor Type alpha |
RET | Rearranged During Transfection |
Syk | Spleen tyrosine kinase |
AXL | Anexelekto |
Data availability
No primary research results, software or code have been included and no new data were generated or analysed as part of this review.
Author contributions
Prashant J. Chaudhari: funding acquisition, conceptualizing, data curation, supervision, formal analysis, validation, writing – original draft, writing – review, and editing. Aditya R. Nemade: data curation, formal analysis, writing – original draft Atul A. Shirkhedkar: validation, formal analysis, writing – review, and editing.
Conflicts of interest
Authors declare no conflict of interest.
Acknowledgements
Corresponding author PJC acknowledge financial support from the Science and Engineering Research Board (SERB)-Department of Science and Technology (DST), New Delhi, India File No SIR/2022/000916. Corresponding author PJC grateful to Danith H. Ly, Professor, Department of Chemistry, Carnegie Mellon University, Pittsburgh, Pennsylvania, USA for providing necessary laboratory facilities. All authors are also thankful to Prof. Sanjay J. Surana, Director, R. C. Patel Institute of Pharmaceutical Education and Research, Shirpur, MS, India, for providing necessary facilities.
Notes and references
- World Health Organization, Fact Sheet 3 February 2022, Cancer, 2022, https://www.who.int/news-room/fact-sheets/detail/cancer, accessed on 12 August 2023 Search PubMed.
- P. J. Chaudhari, S. B. Bari, S. J. Surana, A. A. Shirkhedkar, C. G. Bonde, S. C. Khadse, V. G. Ugale, A. A. Nagar and R. S. Cheke, ACS Omega, 2022, 7(20), 17270–17294 CrossRef CAS PubMed.
- P. J. Chaudhari, S. B. Bari, S. J. Surana, A. A. Shirkhedkar, S. Wakode, S. Shelar, R. Racharla, V. G. Ugale and M. Ghodke, J. Mol. Struct., 2022, 1247, 1–280 CrossRef.
- P. L. Bedard, D. M. Hyman, M. S. Davids and L. L. Siu, Lancet, 2020, 395(10229), 1078–1088 CrossRef CAS PubMed.
- L. N. Puls, M. Eadens and W. Messersmith, Oncologist, 2011, 16(5), 566–578 CrossRef CAS PubMed.
- G. V. Long, D. Stroyakovskiy, H. Gogas, E. Levchenko, F. de Braud, J. Larkin, C. Garbe, T. Jouary, A. Hauschild, J. J. Grob and V. Chiarion Sileni, N. Engl. J. Med., 2011, 371(20), 1877–1888 CrossRef PubMed.
- A. D. Boran and R. Iyengar, Curr. Opin. Drug Discovery Dev., 2010, 13(3), 297 CAS.
- D. Hanahan and J. Folkman, Cell, 1996, 86(3), 353–364 CrossRef CAS PubMed.
- P. Lenzi, G. Bocci and G. Natale, Angiogenesis, 2016, 19(2), 255–256 CrossRef PubMed.
- E. Fagiani and G. Christofori, Cancer Lett., 2013, 328(1), 18–26 CrossRef CAS PubMed.
- W. Wu, X. Li, G. Zuo, J. Pu, X. Wu and S. Chen, Curr. Pharm. Des., 2018, 24(4), p451–p464 CrossRef PubMed.
- K. El-Adl, A. G. A. El-Helby, H. Sakr, R. R. Ayyad, H. A. Mahdy, M. Nasser, H. S. Abulkhair and S. S. El-Hddad, Pharm., 2021, 354(2), 2000279 CAS.
- T. Adair and J. Montani, Angiogenesis, Morgan & Claypool Life Sciences, 2010 Search PubMed.
- S. Vandekeere, M. Dewerchin and P. Carmeliet, Microcirculation, 2015, 22(7), 509–517 CrossRef PubMed.
- N. P. Omorphos, C. Gao, S. S. Tan and M. S. Sangha, Mol. Biol. Rep., 2021, 48, 941–950 CrossRef CAS PubMed.
- N. Kuol, L. Stojanovska, V. Apostolopoulos and K. Nurgali, Cancer Microenviron., 2018, 11, 1–11 CrossRef CAS PubMed.
- A. El-Fiqi, N. Mandakhbayar, S. B. Jo, J. C. Knowles, J. H. Lee and H. W. Kim, Bioact. Mater., 2021, 6(1), 123–136 CAS.
- X. Zheng, Q. Liu, M. Yi, S. Qin and K. Wu, OncoTargets Ther., 2018, 6479–6487 CrossRef CAS PubMed.
- M. Huang, Y. Lin, C. Wang, L. Deng, M. Chen, Y. G. Assaraf, Z. S. Chen and W. Ye, Drug Resistance Updates, 2022, 100849 CrossRef CAS PubMed.
- P. Chaudhari, S. Bari, S. Surana, A. Shirkhedkar, S. Wakode, S. Shelar, S. Racharla, V. Ugale and M. Ghodke, An overview, J. Mol. Struct., 2022, 1247, 131280 CrossRef CAS.
- Z. L. Liu, H. H. Chen, L. L. Zheng, L. P. Sun and L. Shi, r cancer, Signal Transduction Targeted Ther., 2023, 8(1), 198 CrossRef CAS PubMed.
- P. J. Keck, S. D. Hauser, G. Krivi, K. Sanzo, T. Warren, J. Feder and D. T. Connolly, Science, 1989, 246(4935), 1309–1312 CrossRef CAS PubMed.
- N. Ferrara, Int. J. Dev. Biol., 2011, 55(4–5), 383–388 Search PubMed.
- D. R. Senger, S. J. Galli, A. M. Dvorak, C. A. Perruzzi, V. S. Harvey and H. F. Dvorak, Science, 1983, 219(4587), 983–985 CrossRef CAS PubMed.
- C. Selvam, C. D. Mock, O. P. Mathew, K. Ranganna and R. Thilagavathi, Mol. Inf., 2020, 39(7), 1900150 CrossRef CAS PubMed.
- Y. Zhao and A. A. Adjei, Oncologist, 2015, 20(6), 660–673 CrossRef CAS PubMed.
- S. Li, R. Zhang and H. Du, J. Chem., 2013, 1, 374804 CrossRef.
- S. Pathania, O. T. Pentikäinen and P. K. Singh, Biochim. Biophys. Acta, Rev. Cancer, 2021, 1876(2), 188631 CrossRef CAS PubMed.
- X. Jiang, J. Wang, X. Deng, F. Xiong, S. Zhang, Z. Gong, X. Li, K. Cao, H. Deng, Y. He and Q. Liao, J. Exp. Clin. Cancer Res., 2020, 39(1), 1–19 CrossRef PubMed.
- M. Zajkowska, E. Lubowicka, M. Szmitkowski, S. Ławicki and Z. Czynniki, Diagn. Lab., 2018, 54, 105–112 CrossRef.
- S. J. Harper and D. O. Bates, Nat. Rev. Cancer, 2008, 8(11), 880–887 CrossRef CAS PubMed.
- D. Dakowicz, M. Zajkowska and B. Mroczko, Int. J. Mol. Sci., 2022, 23(6), 3375 CrossRef CAS PubMed.
- M. Lohela, M. Bry, T. Tammela and K. Alitalo, Curr. Opin. Cell Biol., 2009, 21(2), 154–165 CrossRef CAS PubMed.
- C. S. Melincovici, A. B. Boşca, S. Şuşman, M. Mărginean, C. Mihu, M. Istrate, I. M. Moldovan, A. L. Roman and C. M. Mihu, Rom. J. Morphol. Embryol., 2018, 59(2), 455–467 Search PubMed.
- H. Ueno, H. Mochizuki, Y. Akagi, T. Kusumi, K. Yamada, M. Ikegami, H. Kawachi, S. Kameoka, Y. Ohkura, T. Masaki and R. Kushima, J. Clin. Oncol., 2012, 30(13), 1519–1526 CrossRef PubMed.
- K. K. Brogowska, M. Zajkowska and B. Mroczko, J. Clin. Med., 2023, 12(6), 2412 CrossRef CAS PubMed.
- D. Dakowicz, M. Zajkowska and B. Mroczko, Int. J. Mol. Sci., 2022, 23(6), 3375 CrossRef CAS PubMed.
- M. Kanda, S. Nomoto, Y. Nishikawa, H. Sugimoto, N. Kanazumi, S. Takeda and A. Nakao, J. Surg. Oncol., 2008, 23(6), 190–196 CrossRef PubMed.
- J. Wang, Y. Huang, J. Zhang, B. Xing, W. Xuan, H. Wang, H. Huang, J. Yang and J. Tang, Cancer Lett., 2018, 23(6), 176–184 CrossRef PubMed.
- M. Zajkowska, M. Szmitkowski, S. Ławicki, E. Lubowicka and J. Mol, Diagn., 2019, 23(6), 105–112 Search PubMed.
- T. B. Kristensen, M. L. Knutsson, M. Wehland, B. E. Laursen, D. Grimm, E. Warnke and N. E. Magnusson, Int. J. Mol. Sci., 2014, 23(6), 23024–23041 CrossRef PubMed.
- M. Çelenk, H. Yıldırım, A. Tektemur, M. Balbaba and M. Erdağ, Int. Ophthalmol., 2023, 1–9 Search PubMed.
- L. Lian, X. L. Li, M. D. Xu, X. M. Li, M. Y. Wu, Y. Zhang, M. Tao, W. Li, X. M. Shen, C. Zhou and M. Jiang, BMC Cancer, 2019, 23(6), 1–15 Search PubMed.
- L. M. Ellis and D. J. Hicklin, Nat. Rev. Cancer, 2019, 23(6), 579–591 Search PubMed.
- N. E. Kaufman, S. Dhingra, S. D. Jois and M. D. G. H. Vicente, Molecules, 2021, 23(6), 1076 CrossRef PubMed.
- M. Jeltsch, V. M. Leppänen, P. Saharinen and K. Alitalo, Cold Spring Harbor Perspect. Biol., 2013, 23(6), 009183 Search PubMed.
- F. W. Peng, D. K. Liu, Q. W. Zhang, Y. G. Xu and L. Shi, Expert Opin. Ther. Pat., 2017, 23(6), 987–1004 CrossRef PubMed.
- K. B. Chandler, D. R. Leon, J. Kuang, R. D. Meyer, N. Rahimi and C. E. Costello, J. Biol. Chem., 2019, 23(6), 13117–13130 CrossRef PubMed.
- T. W. Chung, E. Y. Kim, H. J. Choi, C. W. Han, S. B. Jang, K. J. Kim, L. Jin, Y. J. Koh and K. T. Ha, Exp. Mol. Med., 2019, 23(6), 1–13 Search PubMed.
- X. Wang, A. M. Bove, G. Simone and B. Ma, Front. Cell Dev. Biol., 2020, 23(6), 599281 CrossRef PubMed.
- S. J. Modi and V. M. Kulkarni, Med. Drug Discovery, 2019, 2, 100009 CrossRef.
- A. A. Shah, M. A. Kamal and S. Akhtar, Curr. Drug Metab., 2021, 22(1), 50–59 CAS.
- K. Holmes, O. L. Roberts and A. M. Thomas, et al, Cell Signal, 2007, 19(10), 2003–2012 CrossRef CAS PubMed.
- L. Claesson-Welsh, Cold Spring Harbor Perspect. Biol., 2012, 2, 1–21 Search PubMed.
- K. El-Adl, A. G. A. El-Helby, H. Sakr, I. H. Eissa, S. S. El-Hddad and F. M. homan, Bioorg. Chem., 2020, 102, 104059 CrossRef CAS PubMed.
- A. G. A. El-Helby, H. Sakr, I. H. Eissa, A. A. Al-Karmalawy and K. El-Adl, Arch. Pharm., 2012, 352(12), 1900178 CrossRef PubMed.
- N. Moradi-Gharibvand and B. Hashemibeni, Adv. Biomed. Res., 2023, 12(1), 124 CAS.
- M. Shibuya, Cell Struct. Funct., 2001, 26(1), 25–35 CrossRef CAS PubMed.
- D. Basagiannis, S. Zografou, C. Murphy, T. Fotsis, L. Morbidelli, M. Ziche, C. Bleck, J. Mercer and S. Christoforidis, J. Cell Sci., 2016, 129(21), 4091–4104 CrossRef CAS PubMed.
- C. S. Melincovici, A. B. Boşca, S. Şuşman, M. Mărginean, C. Mihu, M. Istrate, I. M. Moldovan, A. L. Roman and C. M. Mihu, Rom. J. Morphol. Embryol., 2018, 59(2), 455–467 Search PubMed.
- M. Shibuya, BMB Rep., 2006, 39(5), 469–478 CrossRef CAS PubMed.
- G. D. Yancopoulos, S. Davis, N. W. Gale, J. S. Rudge and S. J. Wiegand, Nature, 2000, 407(6801), 242–248 CrossRef CAS PubMed.
- N. P. Omorphos, C. Gao, S. S. Tan and M. S. Sangha, Mol. Biol. Rep., 2021, 48, 941–950 CrossRef CAS PubMed.
- L. Ji, M. Wu and Z. Li, Molecules, 2018, 23(8), 2047 CrossRef PubMed.
- S. M. Wilhelm, C. Carter, L. Tang, D. Wilkie, A. McNabola, H. Rong, C. Chen, X. Zhang, P. Vincent, M. McHugh and Y. Cao, Cancer Res., 2004, 64(19), 7099–7109 CrossRef CAS PubMed.
- S. Nakao, S. Zandi, Y. Hata, S. Kawahara, R. Arita, A. Schering, D. Sun, M. I. Melhorn, Y. Ito, N. Lara-Castillo and T. Ishibashi, J. Am. Soc. Hematol., 2011, 117(3), 1081 CAS.
- V. L. Bodiga, J. Bathula, M. R. Kudle, P. K. Vemuri and S. Bodiga, Bioorg. Med. Chem., 2020, 28(23), 115809 CrossRef CAS PubMed.
- J. A. D. Pablo-Moreno, L. J. Serrano, L. Revuelta, M. J. Sánchez and A. Liras, Int. J. Mol. Sci., 2022, 23(15), 8283 CrossRef CAS PubMed.
- M. Shibuya, BMB Rep., 2006, 39(5), 469–478 CrossRef CAS PubMed.
- R. Lugano, M. Ramachandran and A. Dimberg, Cell. Mol. Life Sci., 2020, 77, 1745–1770 CrossRef CAS PubMed.
- R. Luck, S. Urban, A. Karakatsani, E. Harde, S. Sambandan, L. Nicholson, S. Haverkamp, R. Mann, A. Martin-Villalba, E. M. Schuman and A. Acker-Palmer, eLife, 2019, 8, 49818 CrossRef PubMed.
- R. O. Smith, T. Ninchoji, E. Gordon, H. André, E. Dejana, D. Vestweber, A. Kvanta and L. Claesson-Welsh, eLife, 2020, 9, 54056 CrossRef PubMed.
- K. Kajal, S. Mondal, S. K. Wahan and P. Patel, Chem. Biodiversity, 2023, 202200847–e202200847 Search PubMed.
- N. Tripathi, N. Bhardwaj, B. Singh and S. K. Jain, Chem. Pap., 2024, 78(7), 4347–4360 CrossRef CAS.
- S. Koch, S. Tugues, X. Li, L. Gualandi and L. Claesson-Welsh, Biochem. J., 2011, 437(2), 169–183 CrossRef CAS PubMed.
- K. Kajal, S. Mondal, S. K. Wahan and P. Patel, Chem. Biodiversity, 2023, 202200847–e202200847 Search PubMed.
- C. Liang, X. Yuan, Z. Shen, Y. Wang and L. Ding, Mol. Ther.--Oncolytics, 2022, 17(24), 577–584 CrossRef PubMed.
- X. Zhang, m. Zhu, L. Xie, X. Sun, J. Xu, Y. Guo, D. Liu, Y. Shi, X. Xu and E. Song, J. Ophthalmol., 2020, 20(1), 5905269 Search PubMed.
- L. Li, F. Kong, L. Zhang, X. Li, X. Fu, X. Wang, j. Wu, F. Zhang, L. Ren and M. Zhang, Invest. New Drugs, 2020, 38, 1847–1853 CrossRef CAS PubMed.
- E. Allen, B. Walters and D. Hanahan, Brivanib, Clin. Cancer Res., 2011, 17(16), 5299–5310 CrossRef CAS PubMed.
- Q. Liang, J. Wang, L. Zhao, J. Hou, Y. Hu and J. Shi, Eur. J. Med. Chem., 2021, 214, 113–205 CrossRef PubMed.
- Y. Luo, F. Jiang, T. B. Cole, V. P. Hradil, D. Reuter, A. Chakravartty, D. H. Albert, S. K. Davidsen, B. F. Cox, E. M. McKeegan and G. B. Fox, Cancer Chemother. Pharmacol., 2012, 69, 911–921 CrossRef CAS PubMed.
- F. H. Tan, T. L. Putoczki, S. S. Stylli and R. B. Luwor, OncoTargets Ther., 2019, 18, 635–645 CrossRef PubMed.
- Z. Hao and I. Sadek, OncoTargets Ther., 2016, 8, 5495–5505 CrossRef PubMed.
- S. Zschäbitz and C. Grüllich, Mol. Oncol., 2018, 187–198 Search PubMed.
- C. Orbegoso, G. Marquina, A. George and S. Banerjee, Expert Opin. Pharmacother., 2017, 18(15), 1637–1648 CrossRef CAS PubMed.
- Y. Gao, F. Li, X. Ni, S. Yang, H. Liu, X. Wu, J. Liu and J. Ma, RSC Adv., 2023, 13(41), 28462–28480 RSC.
- T. J. Ettrich and T. Seufferlein, Recent Results in Cancer Research, Springer, 2018, vol. 211 Search PubMed.
- T. A. Farghaly, W. A. Al-Hasani and H. G. Abdulwahab, Expert Opin. Ther. Pat., 2021, 31(11), 989–1007 CrossRef CAS PubMed.
- S. Ishihara, N. Onoda, S. Noda, Y. Asano, Y. Tauchi, T. Morisaki, S. Kashiwagi, T. Takashima and M. Ohira, Int. J. Oncol., 2019, 55(5), 1069–1076 CAS.
- M. H. Langenberg, P. O. Witteveen, J. Roodhart, M. P. Lolkema, H. M. Verheul, M. Mergui-Roelvink, E. Brendel, J. Krätzschmar, B. Loembé, A. Nol-Boekel and O. Christensen, Ann. Oncol., 2011, 22(11), 2508–2515 CrossRef CAS PubMed.
- D. G. IJzendoorn, S. Sleijfer, H. Gelderblom, F. A. Eskens, G. J. Van Leenders, K. Szuhai and J. V. Bovée, Clin. Cancer Res., 2018, 24(11), 2678–2687 CrossRef PubMed.
- B. D. Smith, M. D. Kaufman, C. B. Leary, B. A. Turner, S. C. Wise, Y. M. Ahn, R. J. Booth, T. M. Caldwell, C. L. Ensinger, M. M. Hood and W. P. Lu, Mol. Cancer Ther., 2015, 14(9), 2023–2034 CrossRef CAS PubMed.
- S. Miyamoto, S. Kakutani, Y. Sato, A. Hanashi, Y. Kinoshita and A. Ishikawa, Jpn. J. Clin. Oncol., 2018, 48(6), 503–513 CrossRef PubMed.
- C. Carmichael, C. Lau, D. Y. Josephson and S. K. Pal, Clin. Adv. Hematol. Oncol., 2012, 10(5), 307–314 Search PubMed.
- E. Rijavec, C. Genova, G. Barletta, F. Biello, M. G. Dal Bello, S. Coco, A. Truini, I. Vanni, A. Alama, F. Boccardo and F. Grossi, Expert Opin. Pharmacother., 2014, 15(12), 1771–1780 CrossRef CAS PubMed.
- H. M. Chen, C. H. Tsai and W. C. Hung, Oncotarget, 2015, 6(17), 14940 CrossRef PubMed.
- C. Zhang, C. Tan, H. Ding, T. Xin and Y. Jiang, Curr. Pharm. Des., 2012, 18(20), 2921–2935 CrossRef CAS PubMed.
- L. Stitzlein, P. S. Rao and R. Dudley, Expert Opin. Invest. Drugs, 2019, 28(2), 121–130 CrossRef CAS PubMed.
- F. Ciardiello and G. Tortora, N. Engl. J. Med., 2008, 358(11), 1160–1174 CrossRef CAS PubMed.
- I. H. Eissa, M. K. Ibrahim, A. M. Metwaly, A. Belal, A. B. Mehany, A. A. Abdelhady, M. A. Elhendawy, M. M. Radwan, M. A. ElSohly and H. A. Mahdy, Bioorg. Chem., 2021, 107, 104532 CrossRef CAS PubMed.
- K. El-Adl, A. G. A. El-Helby, R. R. Ayyad, H. A. Mahdy, M. M. Khalifa, H. A. Elnagar, A. B. Mehany, A. M. Metwaly, M. A. Elhendawy, M. M. Radwan and M. A. ElSohly, Bioorg. Med. Chem., 2021, 29, 115872 CrossRef CAS PubMed.
- S. Choudhary, A. Doshi, L. Luckett-Chastain, M. Ihnat, E. Hamel, S. L. Mooberry and A. Gangjee, Bioorg. Med. Chem., 2021, 35, 116061 CrossRef CAS PubMed.
- A. E. Abdallah, S. I. Eissa, M. M. S. Al Ward, R. R. Mabrouk, A. B. Mehany and M. A. El-Zahabi, Bioorg. Chem., 2021, 109, 104695 CrossRef CAS PubMed.
- S. R. Abd El Hadi, D. S. Lasheen, D. H. Soliman, E. Z. Elrazaz and K. A. Abouzid, Bioorg. Chem., 2020, 101, 103961 CrossRef PubMed.
- I. H. Eissa, A. G. A. El-Helby, H. A. Mahdy, M. M. Khalifa, H. A. Elnagar, A. B. Mehany, A. M. Metwaly, M. A. Elhendawy, M. M. Radwan, M. A. ElSohly and K. El-Adl, Bioorg. Chem., 2020, 105, 104380 CrossRef CAS PubMed.
- H. Fan, D. Wei, K. Zheng, X. Qin, L. Yang, Y. Yang, Y. Duan, Y. Xu and L. Hu, Eur. J. Med. Chem., 2019, 175, 349–356 CrossRef CAS PubMed.
- M. G. El-Gazzar, R. M. El-Hazek, N. H. Zaher and M. A. El-Ghazaly, Bioorg. Chem., 2019, 92, 103251 CrossRef PubMed.
- R. Wang, H. Liu, Y. Y. You, X. Y. Wang, B. BLv, L. Q. Cao, J. Y. Xue, Y. G. Xu and L. Shi, Bioorg. Med. Chem. Lett., 2021, 36, 127788 CrossRef CAS PubMed.
- H. A. Mahdy, M. K. Ibrahim, A. M. Metwaly, A. Belal, A. B. Mehany, K. M. El-Gamal, A. M. El-Sharkawy, M. A. Elhendawy, M. M. Radwan, M. A. Elsohly and I. H. Eissa, Bioorg. Chem., 2020, 94, 103422 CrossRef CAS PubMed.
- H. A. Abuelizz, M. Marzouk, A. H. Bakheit, H. M. Awad, M. M. Soltan, A. M. Naglah and R. Al-Salahi, Molecules, 2020, 25(24), 5944 CrossRef CAS PubMed.
- A. S. Altamimi, A. S. El-Azab, S. G. Abdelhamid, M. A. Alamri, A. H. Bayoumi, S. M. Alqahtani, A. S. Alabbas, A. I. Altharawi, A. A. Alossaimi and M. A. Mohamed, Molecules, 2021, 26(10), 2992 CrossRef CAS PubMed.
- D. Wei, H. Fan, K. Zheng, X. Qin, L. Yang, Y. Yang, Y. Duan, Q. Zhang, C. Zeng and L. Hu, Bioorg. Chem., 2019, 88, 102916 CrossRef CAS PubMed.
- N. A. Alsaif, M. A. Dahab, M. M. Alanazi, A. J. Obaidullah, A. A. Al-Mehizia, M. M. Alanazi, S. Aldawas, H. A. Mahdy and H. Elkady, Bioorg. Chem., 2021, 110, 104807 CrossRef CAS PubMed.
- N. A. Alsaif, M. S. Taghour, M. M. Alanazi, A. J. Obaidullah, W. A. Alanazi, A. Alasmari, A. Albassam, H. Dahab and H. A. Mahdy, Bioorg. Med. Chem., 2021, 46, 116384 CrossRef CAS PubMed.
- K. El-Adl, H. M. Sakr, R. G. Yousef, A. B. Mehany, A. M. Metwaly, M. A. Elhendawy, M. M. Radwan, M. A. ElSohly, H. S. Abulkhair and I. H. Eissa, Bioorg. Chem., 2021, 114, 105105 CrossRef CAS PubMed.
- M. M. Alanazi, H. A. Mahdy, N. A. Alsaif, A. J. Obaidullah, H. M. Alkahtani, A. A. Al-Mehizia, S. M. Alsubaie, A. M. Dahab and I. H. Eissa, Bioorg. Chem., 2021, 112, 104949 CrossRef CAS PubMed.
- X. Li, C. Wu, X. Lin, X. Cai, L. Liu, G. Luo, Q. You and H. Xiang, Eur. J. Med. Chem., 2019, 161, 445–455 CrossRef CAS PubMed.
- Y. Yao, Z. Liu, M. Zhao, Z. Chen, P. Li, Y. Zhang, Y. Wang, C. Zhao, C. Long, X. Chen and J. Yang, Acta Pharm. Sin. B, 2020, 10(8), 1453–1475 CrossRef CAS PubMed.
- M. J. Mphahlele, M. M. Maluleka, S. T. Lerooibaaki and Y. S. Choong, J. Mol. Struct., 2020, 1222, 128907 CrossRef CAS.
- A. Hassan, M. Badr, H. A. Hassan, D. Abdelhamid and G. E. D. A. Abuo-Rahma, Bioorg. Med. Chem., 2021, 40, 116168 CrossRef CAS PubMed.
- M. S. Taghour, H. Elkady, W. M. Eldehna, N. M. El-Deeb, A. M. Kenawy, E. B. Elkaeed, A. A. Alsfouk, M. S. Alesawy, A. M. Metwaly and I. H. Eissa, J. Enzyme Inhib. Med. Chem., 2022, 37(1), 1903–1917 CrossRef CAS PubMed.
- M. A. Abdelgawad, A. M. Hayallah, S. N. A. Bukhari, A. Musa, M. Elmowafy, H. M. Abdel-Rahman and M. K. A. El-Gaber, Pharmaceuticals, 2021, 15(11), 1416 CrossRef PubMed.
- S. A. Al-Hussain, T. A. Farghaly, M. E. Zaki, H. G. Abdulwahab, N. T. Al-Qurashi and Z. A. Muhammad, Bioorg. Chem., 2020, 105, 104330 CrossRef CAS PubMed.
- D. P. Wang, K. L. Liu, X. Y. Li, G. Q. Lu, W. H. Xue, X. H. Qian and F. H. Meng, Eur. J. Med. Chem., 2021, 211, 113083 CrossRef CAS PubMed.
- Y. Chang, Y. Yuan, Q. Zhang, Y. Rong, Y. Yang, M. Chi, Z. Liu, Y. Zhang, P. Yu and Y. Teng, RSC Adv., 2020, 10(2), 1191–1197 RSC.
- R. M. Sbenati, S. O. Zaraei, M. I. El-Gamal, H. S. Anbar, H. Tarazi, M. M. Zoghbor, N. A. Mohamood, M. M. Khakpour, D. M. Zaher, H. A. Omar and N. N. Alach, Design, Eur. J. Med. Chem., 2021, 210, 113081 CrossRef CAS PubMed.
- S. Sana, V. G. Reddy, S. Bhandari, T. S. Reddy, R. Tokala, A. P. Sakla, S. K. Bhargava and N. Shankaraiah, Eur. J. Med. Chem., 2020, 200, 112457 CrossRef CAS PubMed.
- H. M. Roaiah, I. A. Ghannam, I. H. Ali, A. M. El Kerdawy, M. M. Ali, S. E. S. Abbas and S. S. El-Nakkady, Arch. Pharm., 2018, 351(2), 1700299 CrossRef PubMed.
- H. K. Mahmoud, T. A. Farghaly, H. G. Abdulwahab, N. T. Al-Qurashi and M. R. Shaaban, Eur. J. Med. Chem., 2020, 208, 112752 CrossRef CAS PubMed.
- X. R. Wang, S. Wang, W. B. Li, K. Y. Xu, X. P. Qiao, X. L. Jing, Z. X. Wang, C. J. Yang and S. W. Chen, Eur. J. Med. Chem., 2021, 213, 113192 CrossRef CAS PubMed.
- X. R. Wang, S. Wang, H. X. Mu, K. Y. Xu, X. T. Wang, J. T. Shi, Q. H. Cui, L. W. Zhang and S. W. Chen, Eur. J. Med. Chem., 2022, 244, 114821 CrossRef CAS PubMed.
- N. Wei, J. Liang, S. Peng, Q. Sun, Q. Dai and M. Dong, Molecules, 2018, 23(4), 747 CrossRef PubMed.
- A. Srivastava, K. Fatima, E. Fatima, A. Singh, A. Singh, A. Shukla, S. Luqman, K. Shanker, D. Chanda, F. Khan and A. S. Negi, Eur. J. Med. Chem., 2020, 154, 105513 CAS.
- Y. El-Dash, E. Elzayat, A. M. Abdou and R. A. Hassan, Bioorg. Chem., 2021, 114, 105137 CrossRef CAS PubMed.
- S. A. El-Metwally, M. M. Abou-El-Regal, I. H. Eissa, A. B. Mehany, H. A. Mahdy, H. Elkady, A. Elwan and E. B. Elkaeed, Bioorg. Chem., 2021, 112, 104947 CrossRef CAS PubMed.
- E. Z. Elrazaz, R. A. Serya, N. S. Ismail, A. Albohy, D. A. Abou El Ella and K. A. Abouzid, Bioorg. Chem., 2021, 113, 105019 CrossRef CAS PubMed.
- A. Faraji, T. O. Bakhshaiesh, Z. Hasanvand, R. Motahari, E. Nazeri, M. A. Boshagh, L. Firoozpour, H. Mehrabi, A. Khalaj, R. Esmaeili and A. Foroumadi, Eur. J. Med. Chem., 2021, 209, 112942 CrossRef CAS PubMed.
- A. Ghith, K. M. Youssef, N. S. Ismail and K. A. Abouzid, Bioorg. Chem., 2019, 83, 111–128 CrossRef CAS PubMed.
- R. Motahari, M. A. Boshagh, S. Moghimi, F. Peytam, Z. Hasanvand, T. Oghabi Bakhshaiesh, R. Foroumadi, H. Bijanzadeh, L. Firoozpour, A. Khalaj and R. Esmaeili, Sci. Rep., 2022, 12(1), 9683 CrossRef CAS PubMed.
- A. M. Sayed, F. A. Taher, M. R. Abdel-Samad, M. S. El-Gaby, K. El-Adl and N.
M. Saleh, Bioorg. Chem., 2021, 108, 104669 CrossRef CAS PubMed.
- G. H. Al-Ansary, T. Nasr, H. Taha, W. Fayad and S. Mahgoub, Bioorg. Chem., 2021, 107, 104640 CrossRef CAS PubMed.
- G. Luo, Z. Tang, K. Lao, X. Li, Q. You and H. Xiang, Eur. J. Med. Chem., 2018, 150, 783–795 CrossRef CAS PubMed.
- M. K. Sobhy, S. Mowafy, D. S. Lasheen, N. A. Farag and K. A. Abouzid, Bioorg. Chem., 2019, 89, 102988 CrossRef CAS PubMed.
- W. Sun, S. Hu, S. Fang and H. Yan, Design, Bioorg. Chem., 2018, 78, 393–405 CrossRef CAS PubMed.
- H. T. Abdel-Mohsen, M. A. Omar, A. M. El Kerdawy, A. E. Mahmoud, M. M. Ali and H. I. El Diwani, Eur. J. Med. Chem., 2019, 179, 707–722 CrossRef CAS PubMed.
- A. A. Marzouk, S. A. Abdel-Aziz, K. S. Abdelrahman, A. S. Wanas, A. M. Gouda, B. G. Youssif and M. Abdel-Aziz, Bioorg. Chem., 2020, 102, 104090 CrossRef CAS PubMed.
- M. Adel and C. K. A. valuation, Bioorg. Chem., 2022, 127, 106006 CrossRef CAS PubMed.
- D. X. Ying, J. Wang, X. F. Li, W. Zhang and G. W. Rao, Future Med. Chem., 2022, 14(22), 1649–1662 CrossRef CAS PubMed.
- M. H. Mahnashi, F. F. El-Senduny, M. A. Alshahrani and M. A. Abou-Salim, Pharmaceuticals, 2022, 15(2), 246 CrossRef CAS PubMed.
- Z. Ruzi, K. Bozorov, L. Nie, J. Zhao and H. A. Aisa, Biomed. Pharmacother., 2022, 156, 113948 CrossRef CAS PubMed.
- Y. Wang, S. Wan, Z. Li, Y. Fu, G. Wang, J. Zhang and X. Wu, Eur. J. Med. Chem., 2018, 155, 210–228 CrossRef CAS PubMed.
- Q. Zhang, Q. Shen, L. Gao, L. Li, J. Tong, Y. Chen and W. Lu, Eur. J. Med. Chem., 2018, 158, 428–441 CrossRef CAS PubMed.
- M. M. Abd El-Mageed, A. A. Eissa, A. E. S. Farag and E. E. A. Osman, Bioorg. Chem., 2021, 116, 105336 CrossRef CAS PubMed.
- M. F. Ahmed and E. Y. Santali, Bioorg. Chem., 2021, 111, 104842 CrossRef CAS PubMed.
- D. R. Parmar, J. Y. Soni, R. Guduru, R. H. Rayani, R. V. Kusurkar, A. G. Vala, S. N. Talukdar, I. H. Eissa, A. M. Metwaly, A. Khalil and V. Zunjar, Bioorg. Chem., 2021, 115, 105206 CrossRef CAS PubMed.
- E. B. Elkaeed, R. G. Yousef, H. Elkady, I. M. Gobaara, B. A. Alsfouk, D. Z. Husein, I. M. Ibrahim, A. M. Metwaly and I. H. Eissa, Molecules, 2022, 27(14), 4606 CrossRef CAS PubMed.
- A. Abdel Haleem, A. O. Mansour, M. AbdelKader and R. K. Arafa, Bioorg. Chem., 2020, 103, 104222 CrossRef CAS PubMed.
- I. H. Ali, H. T. Abdel-Mohsen, M. M. Mounier, M. T. Abo-Elfadl, A. M. El Kerdawy and I. A. Ghannam, Bioorg. Chem., 2022, 105883 CrossRef CAS PubMed.
- A. A. Elmaaty, K. M. Darwish, A. Chrouda, A. A. Boseila, M. A. Tantawy, S. S. Elhady, A. B. Shaik, M. Mustafa and A. A. Al-Karmalawy, ACS Omega, 2021, 7(1), 875–899 CrossRef PubMed.
- A. S. Mostafa, R. M. Gomaa and M. A. Elmorsy, Chem. Biol. Drug Des., 2019, 93(4), 454–463 CrossRef CAS PubMed.
- X. Yuan, Q. Yang, T. Li, K. Liu, Y. Liu, C. Zhu, Z. Li, L. Zhang, C. Zhang, M. Xie and J. Lin, Eur. J. Med. Chem., 2019, 179, 147–165 CrossRef CAS PubMed.
- E. Canh Pham and T. N. Truong, ACS Omega, 2022, 7(37), 33614–33628 CrossRef CAS PubMed.
- M. I. Han, P. Atalay, C. Ü. Tunç, G. Ünal, S. Dayan, Ö. Aydın and Ş. G. Küçükgüzel, Bioorg. Med. Chem., 2021, 37, 116097 CrossRef CAS PubMed.
- F. Khedr, M. K. Ibrahim, I. H. Eissa, H. S. Abulkhair and K. El-Adl, Arch. Pharm., 2021, 354(11), 2100201 CrossRef CAS PubMed.
- H. Singh, A. Thirupathi, B. Das, M. Janni, R. Kumari, S. Singh, M. Rashid, M. Wahajuddin, M. M. Balamurali, K. Jagavelu and S. Peruncheralathan, J. Med. Chem., 2021, 65(1), 120–134 CrossRef PubMed.
- S. M. Abou-Seri, A. A. Eissa, M. G. Behery and F. A. Omar, Synthesis, Bioorg. Chem., 2021, 116, 105334 CrossRef CAS PubMed.
- V. G. Reddy, T. S. Reddy, C. Jadala, M. S. Reddy, F. Sultana, R. Akunuri, S. K. Bhargava, D. Wlodkowic, P. Srihari and A. Kamal, Eur. J. Med. Chem., 2019, 182, 111609 CrossRef PubMed.
- N. M. Saleh, M. S. El-Gaby, K. El-Adl and N. E. Abd El-Sattar, Bioorg. Chem., 2020, 104, 104350 CrossRef CAS PubMed.
- A. Elwan, A. E. Abdallah, H. A. Mahdy, M. A. Dahab, M. S. Taghour, E. B. Elkaeed, A. B. Mehany, A. Nabeeh, M. Adel, A. A. Alsfouk and H. Elkady, Molecules, 2022, 27(15), 5047 CrossRef CAS PubMed.
- O. A. El-Khouly, M. A. Henen, M. A. A. El-Sayed and S. M. El-Messery, Sci. Rep., 2022, 12(1), 17104 CrossRef CAS PubMed.
- H. Adachi, C. Nosaka, S. Atsumi, K. Nakae, Y. Umezawa, R. Sawa, Y. Kubota, C. Nakane, M. Shibuya and Y. Nishimura, J. Antibiot., 2021, 74(10), 734–742 CrossRef CAS PubMed.
- T. K. Mohamed, R. Z. Batran, S. A. Elseginy, M. M. Ali and A. E. Mahmoud, Bioorg. Chem., 2019, 85, 253–273 CrossRef CAS PubMed.
- T. Z. Abolibda, M. Fathalla, B. Farag, M. E. Zaki and S. M. Gomha, Molecules, 2023, 28(2), 689 CrossRef CAS PubMed.
- M. H. Hekal, P. S. Farag, M. M. Hemdan and W. M. El-Sayed, Bioorg. Chem., 2021, 115, 105176 CrossRef CAS PubMed.
- F. Q. Shen, L. Shi, Z. F. Wang, C. R. Wang, J. J. Chen, Y. Liu, H. Y. Qiu and H. L. Zhu, Bioorg. Med. Chem., 2019, 17, 3813–3824 CrossRef PubMed.
- N. Upadhyay, K. Tilekar, S. Safuan, A. P. Kumar, M. Schweipert, F. J. Meyer-Almes and C. S. Ramaa, Bioorg. Chem., 2021, 116, 105350 CrossRef CAS PubMed.
- K. El-Adl, A. G. A. El-Helby, H. Sakr, R. R. Ayyad, H. A. Mahdy, M. Nasser, H. S. Abulkhair and S. S. El-Hddad, Arch. Pharm., 2021, 354(2), 2000279 CrossRef CAS PubMed.
- A. A. Mourad, N. A. Farouk, E. S. H. El-Sayed and A. R. Mahdy, Life Sci., 2021, 277, 119531 CrossRef CAS PubMed.
- M. K. Abdelhameid, I. Zaki, M. R. Mohammed and K. O. Mohamed, Bioorg. Chem., 2020, 101, 103995 CrossRef CAS PubMed.
- S. R. Atta-Allah, A. M. AboulMagd and P. S. Farag, Bioorg. Chem., 2021, 112, 104923 CrossRef CAS PubMed.
- F. P. Pauli, J. R. Martins, T. Paschoalin, M. Ionta, M. L. C. Barbosa and E. J. Barreiro, Arch. Pharm., 2020, 353(11), 2000130 CrossRef CAS PubMed.
- D. Gonggong, X. Zhang, M. Li, et al., Azaindazole macrocyclic compound and use thereof PCT Int. Appl., WO2023040996A1 20230323, 2023.
- X. Qian, Y. L. Zhu et.al., Substituted quinazolines for inhibiting kinase activity, U.S. Pat. Appl. Publ., US20220315581A1 20221006, 2022 Search PubMed.
- Z. Chen, T. Haizong et al., Fused ring compound as fgfr and vegfr dual inhibitor, U.S. Pat.et al. Appl. Publ., US 20220315581 A1 20220610, 2022 Search PubMed.
- Z. Chen, M. Dei et al., Pyridine derivative as fgfr and vegfr dual, U.S. Pat. Appl. Publ., US 20220267324 A1 20220825, 2022 Search PubMed.
- X. Chen, Y. Pan et al., Indoline-1-formamide compound, preparation method therefor, and medical use thereof, U.S. Pat. Appl. Publ., US 20210188806 A1 20210624, 2020 Search PubMed.
- Q. Wenyuan, L. Wang, S. C. Tan et al., 1H-pyrazole derivatives and uses as Syk and VEGFR2 dual target inhibitors, Kor. Pat. Appl. KR 20220130747 A 20220927, 2022.
- M. Dai, W. Zhang, Z. Chen, et al., Imidazopyridine derivatives as dual FGFR and VEGFR inhibitors, CN 113490667 A, 2021.
- H. Tachihiro et al., Pyrrole-substituted indolon derivatives or pharmaceutically acceptable salts thereof, and methods for preparing them and their use, Jap. Pat. Appl. JP 2021535931 A 20221018, 2021.
- F. Sun, N. Possess et al., Tyrosine kinase inhibitor and application thereof, PCT Int. Appl. WO 2018059022 A1 20180405, 2018.
- Q. Zhang, Y. Shannan et al., Dioxazoline compound, preparation method therefor, and uses thereof, Eur. Pat. Appl. EP 3750893 B1 20230419, 2023.
- G. Saxty, C. W. Murray et al., Pyrazolyl quinoxaline kinase inhibitors, U.S. Pat. Appl. Publ, US 20220135544 A1 20220505 2022.
- P. R. Angibaud, et al., Bi-heteroaryl substitute 1,4-benzodiazepines and uses thereof for the treatment of cancer, U.S. Pat. Appl. Publ. US 011542247 B2 20230103, 2023.
- L. Bannen, W. Xu, Y. Wang et al., Compounds and methods of use, U.S. Pat. Appl. Publ, US 20230151003 A1 20230518, 2023.
- Z. Yun, J. Zhan, Multi-targeted tyrosine kinase inhibitors and their pharmaceutical uses, U.S. Pat. Appl. Publ. US 20230124784 A1 20230220, 2023.
- L. C. Bannen, F. Jiang, K. Tso, et al., Preparation of substituted N-[(quinolin-4-yl)-phenyl]-N’-phenylcyclopropane-1,1-dicarboxamides and analogs for the treatment of Axl and Mer receptor tyrosine kinase-dependent disorders.
- Y. Shan, S. Hu, Y. Dong, et al., Preparation of chalcone-based quinazolinone compound from isovanillin as multi-target RTKs inhibitors for anti-tumour drugs, Faming Zhuanli Shenqing. CN 110903253 A 20200324, 2020.
- F. Meng, L. Guoqing, L. Xinyang, et al., Preparing method and application of 2-indolone-triazole antitumour compound, Faming Zhuanli Shenqing. CN 111153889 A 20200515, 2020.
- Q. Zhang, S. Yu, Y. Sun, et al., Urea-substituted aromatic ring-linked dioxane and quinazoline or quinoline compound, composition and application thereof, PCT Int. Appl. WO 2020042972 A1 20200305, 2020.
- J. Liu, Q. Liu, X. Liu, et al., Indazole kinase inhibitor and application, Faming Zhuanli Shenqing. CN 111138426 A 20200512. 2020.
- L. Zhao, X. Gou, Purine series derivative and its preparation method and application as antitumour agents, Faming Zhuanli Shenqing. CN 109384788 A 20190226, 2019.
- J. Li, D. Huang, W. Wang, et al., Preparation of pyrazole derivatives as antitumour drugs, Faming Zhuanli Shenqing. CN 107286140 A 20171024, 2017.
- C. Hu, S. Sun, C. Zhang, et al., 1-Aryl-3-{4-[(pyridin-2-ylmethyl)thio] phenyl}urea compound, its preparation and application, Faming Zhuanli Shenqing. CN 110003176 A 20190712, 2019.
- J. Li, Q. Zhang, B. Lu, et al., Preparation of sulfonylaniline pyrimidine derivatives used as antitumour drugs, Faming Zhuanli Shenqing. CN 110386901 A 20191029, 2019.
- W. Lv, Q. Zhang, D. Xiao, et al., Prepn. of pyrazolopyrimidodiazepine derivs. as multi-targeted inhibitors of Aurora A/B and KDR for the treatment of cancer, Faming Zhuanli Shenqing. CN 109020980 A 20181218, 2018.
- Y. Hu, M. Geng, W. Ren, et al., Preparation of the o-aminoheteroaryl alkynyl-containing compound and their application as anticancer agents, Faming Zhuanli Shenqing. CN 108456163 A 20180828, 2018.
- J. Liu, Y. Chen, Y. Liu, et al., Pyridazinone-4-phenoxypyridine compounds as VEGFR2 inhibitors and their preparation, pharmaceutical compositions and use in the treatment of cancer, Faming Zhuanli Shenqing. CN 109096250 A 20181228, 2018.
|
This journal is © The Royal Society of Chemistry 2024 |
Click here to see how this site uses Cookies. View our privacy policy here.