DOI:
10.1039/D4RA06317A
(Paper)
RSC Adv., 2024,
14, 38547-38559
Efficiency of microbial desalination cells in treating wastewater, desalinating saltwater, and generating bioelectricity in Bangladesh†
Received
1st September 2024
, Accepted 15th November 2024
First published on 6th December 2024
Abstract
Desalination ensures the provision of potable water to those living in coastal areas, thereby guaranteeing access to safe drinking water. Urbanization and industrialization pollute natural water sources with untreated and partially treated wastewater. International researchers have been searching for cost-effective and environmentally friendly solutions to the above-highlighted difficulties. Developed countries efficiently treat wastewater and desalinate seawater at a minimal expense while reducing nonrenewable energy consumption using microbial desalination cells (MDCs). The use of ion exchange resin-based MDCs is expected to remove salt from seawater and produce bioelectricity. This research aimed to build MDCs with two types of electrodes and determine their efficiency in the desalination of seawater and generation of bioelectricity while treating wastewater. Results showed that MDCs with Zn–Cu and carbon fiber cloth electrodes effectively treated textile and tannery effluents. Calculations encompassed several parameters such as current, voltage, power, current density, power density, desalination efficiency, rate, COD reduction, and TDS reduction. MDCs containing Zn–Cu electrodes generated energy and removed 65% COD and 33% TDS while treating wastewater. The most efficient MDC (Zn–Cu electrode-based MDC) reduced the salinity of seawater by 85%. At the same time, using the carbon fiber cloth electrode-based MDC, about 78% salinity could have been reduced from seawater. The maximum and minimum desalination rates for this experiment were 1.24 and 1.096 ppt per day, respectively. MDCs efficiently desalinated saltwater, treated wastewater, and generated bioelectricity. Therefore, for countries such as Bangladesh, this method is economically viable.
1 Introduction
Modern population expansion requires more water and energy. Most people need access to safe drinking water, but natural supplies and groundwater are scarce.1 Many countries, especially developing ones, face water scarcity. Freshwater is the most vital renewable resource for life, food, and industry, and the demand for freshwater has tripled in 50 years.2 Although abundant, 97% of Earth's water comprises oceans, and seawater cannot be used for drinking. So, the freshwater sources are very scarce in our earth. Again, these sources are getting depleted due to anthropogenic interferences and activities. MDCs can desalinate seawater or brackish water to provide freshwater.3 Desalination delivers freshwater in areas lacking potable water but abundant in seawater or brackish water.4 In seaside settings, desalination can produce drinking water. Desalination uses considerable energy. MDCs desalinate, generate power, and treat wastewater.5 Ma and Hou (2019)6 state that MDC technology fits the water-energy nexus matrix. MDCs are studied as bio-electrochemical energy, wastewater, and saltwater desalination systems. New bio-electrochemical systems (BESs) treat wastewater and create power while maintaining water sustainability. Exoelectrogen metabolism converts the chemical energy of organic matter into electricity in BESs.6 Salt is removed by microorganisms in microbial desalination cells. It generates power and handles wastewater.7 It may reduce global water and energy shortages.8 One m3 of saltwater requires 2–15 kW h of thermal distillation or membrane-based RO. Global population growth and energy depletion require alternative energy9 and capturing renewable energy from wastewater could meet expanding energy needs. In the approaching decades, green energy production might fulfill energy needs and produce potable water from saline water using MDC at a low cost while treating wastewater in the same cell. It produces bioelectricity, cleanses wastewater with the help of microorganisms, and separates salt from water better than conventional methods.10 Due to its year-round organic content and nutrients, wastewater is an ideal bioremediation substrate. Power is mainly generated from municipal, industrial, and other effluents.11 Traditional wastewater treatment includes high operational costs, energy waste, and environmental impact. Traditional wastewater treatment costs 3% of the world's power, and sludge disposal 50%.12–14 MDC is used for desalination and wastewater treatment since it is energy-efficient and environmentally friendly.15
Innovation and sustainability characterize microbial desalination cells (MDCs). Low energy utilization helps MDC desalinate saltwater and brackish water.16 The MDC technology will help attain SDGs 6 and 7 in the long run.17 For that, MDCs should be used and introduced at the commercial scale. MDC technology can convert plenty of ocean water into drinking water, which may reduce our water crisis globally. This can help everyone get clean water, especially desert people. Treatment of wastewater enhances environmental sanitation. A commercialized MDC can be used to generate bioelectricity in rural areas where national grid systems are uneconomical or unsustainable for SDG 7.17 The desalination process (reverse osmosis and distillation) and wastewater treatment (primarily aerobic procedures) are energy-intensive and expensive. MDC desalinate the saline water and treat the wastewater simultaneously in an effective operation. Inefficient labor and land use may emerge from this separation. Saline-water desalination and energy-efficient wastewater treatment are intriguing.18,19 The MFC technology has been enhanced and transformed into the microbial desalination cell (MDC) technology. MDCs employ one or more supplementary chambers positioned between the anode and cathode, which are filled with saline solution instead of MFCs. MDC harnesses the electrical potential generated by the oxidation of microorganisms at the anode to drive the movement of salt ions and, consequently, achieve desalination. Over time, the bacteria in the wastewater consume organic matter, purifying the waste while simultaneously generating electricity and removing salt from the water.20 The MDC uses bacteria-generated energy to desalinate. Exoelectrogens present in wastewater degrade organic matter and subsequently pass the newly generated electrons to the anode to produce energy. In the desalination chamber, negative ions (Cl−) are attracted to the anode in order to equalize the charge, as the anode chamber accumulates protons. The transfer of cations (Na+) from the desalination chamber to the cathode chamber is facilitated by the movement of electrons at the surface of the anode through an external wire. This process results in the reduction of oxidized species (electron acceptors) on the cathode.7,19
MDC can treat wastewater and desalinate seawater while generating energy, according to various research studies.4,15,20–22 This research is new to Bangladesh. Thus, Bangladeshi MDC efficiency is unique and its adaptability is intriguing. Cost-benefit efficiency is this study's most significant gap. The primary literature gap showed that MDC ion exchange membranes are expensive. High cost makes ion exchange membrane sheet MDCs questionable regarding usability and cost efficiency. However, most desalination experiments employ ion exchange membrane sheets.2–4,15,17,20–25 Underdeveloped nations cannot afford ion exchange membrane sheets to desalinate saltwater. Researchers have used carbon electrodes to create power.4,15,17,20–22 Many researchers used different types of materials as electrodes to precisely depict the strength and performance of MDC. Only sodium chloride was removed from synthetic saline water in most tests. The literature on seawater desalination is limited by a few studies.5,26,27
An innovative and resource-efficient approach for treating wastewater and desalinating saltwater while creating bioelectricity was presented in the study. Hence, this research aimed to desalinate seawater and treat wastewater while getting bioelectricity in MDC. This study hypothesized that ion exchange resin-based MDCs are solely efficient enough for wastewater treatment, saltwater desalination and bioelectricity generation. An innovative concept has been applied to treat wastewater and desalinate saltwater in the MDCs using ion exchange resin as an ion exchange membrane. This study covered the literature gaps regarding MDCs. It provided an efficient mechanism that can be applied in countries like Bangladesh at a lower cost than in other countries. This study will help implement the steps to improve water management and the desalination approach in developing countries, which will be economically viable for applications.
2 Materials and methods
2.1 Design of MDC
The design idea of MDC was taken from the literature7,15,19,21 and MDC was developed based on those ideas. Fig. 1 shows the MDC design used in this research. This design comprises three chambers: an anode chamber, a desalination chamber, and a cathode chamber. This design demonstrates an ion exchange resin-based membrane, which functions as an anion and cation exchange membrane. The desalination chamber displays the compartment containing seawater. The anode chamber is the compartment where wastewater is stored, whereas the cathode chamber is the section where distilled water is stored. An external electrical circuit connects the anode and cathode, enabling the movement of electrons produced during microbial metabolism to generate an electrical current. The compartments have been interconnected using copper wire. The design incorporates electrodes (Zn–Cu and carbon fiber cloth) that complete the cell and commence the flow of electrons, generating electricity.
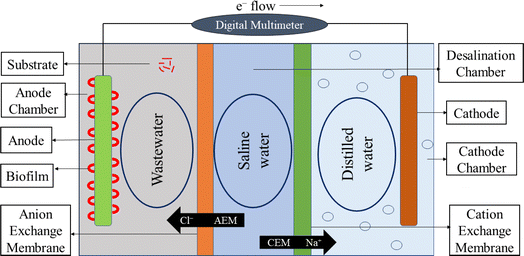 |
| Fig. 1 Design of an MDC. | |
2.2 Mechanism of MDC
Desalination at the MDC is defined as being reliant on energy generated by bacteria (exoelectrogens). Exoelectrogens in wastewater generate energy by decomposing organic matter and transferring the resulting electrons to the anode. As a result, negative ions (Cl−) are attracted to the anode chamber from the desalination chamber to restore electrical neutrality.19 When electrons are transmitted from the anode to the cathode, they travel over a wire before being used to neutralize oxidized species at the cathode.7 Na+ cations are transferred from the desalination chamber to the cathode chamber as a result of this process. These processes desalinate saltwater, treat wastewater, and generate bioelectricity. Fig. 1 provides an illustration of the entire concept.
2.3 Construction of MDC
Six glass containers have been used to make three-chambered MDCs for each wastewater type, one for Zn–Cu electrodes and one for carbon fiber electrodes. The MDC compared two different anode materials (Zn/carbon fiber cloth) and cathode materials (Cu/carbon fiber fabric) and determined which was more effective. The MDC's three chambers (anode, desalination, and cathode) were separated by cation exchange membranes and anion exchange membranes. Before being inserted into the MDC, the electrodes underwent deionization. The electrodes were submerged in distilled water for 24 hours. Wastewater was used in the anode chamber, while distilled water was used in the cathode chamber. Distilled water is used in the cathode chamber because of its purity and lack of ion conductivity, as well as to avoid any contaminants in the cathode chamber. The desalination chamber was located in the middle room. 2 L volumes were used in each chamber, and the volume ratio for the three chambers was determined randomly. Copper wires were used to establish electrical contact between the electrodes and the chambers, allowing electrons to flow from the anode to the cathode. A 2-foot-long wire was installed in each cell. No air could get into the anode chambers since they were properly sealed. In contrast, the cathode chambers had a hole in them and were periodically ventilated using an air pump to keep the air and oxygen flowing through them. Distilled water was used to thoroughly rinse all components before assembly. For 20 days, its electricity output was monitored while it was kept at room temperature. Fig. S1† shows a flow diagram of this process.
2.4 Ion exchange membrane preparation
Ion exchange resin has been used in this study as an ion exchange membrane. For this, 200 g ion exchange resin (cation & anion) was measured and taken in a 20-inch-long cotton pad in a uniform way. Subsequently, it was fully wrapped with Markin cloth and used as an ion exchange membrane in the MDC.
2.5 Sample collection
This research employed two wastewater samples and seawater for desalination. Fig. S2† shows the sampling location of wastewater. Sterilized sample bottles were used to collect the samples of wastewater from sampling locations – Pride Textile Ltd and Anjuman Trading Corporation Ltd. The wastewater sample bottles were transported in ice boxes from the site to the lab. After collection, different physicochemical parameters were measured in the laboratory. The wastewater was kept at 4 °C until it was ready to be used in the MDC, at which point it was brought under room temperature. Seawater was collected from the Bay of Bengal (Cox's Bazar, District Bangladesh).
2.6 Analysis and calculation
In this study, different electrochemical parameters such as power, current density and power density were measured using eqn (1)–(3). The COD and TDS removal efficiency were measured using eqn (4) and (5). The salt removal efficiency and desalination rate were measured using eqn (6) and (7). |
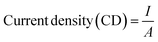 | (2) |
|
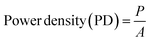 | (3) |
|
 | (4) |
|
 | (5) |
|
 | (6) |
|
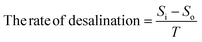 | (7) |
In eqn (1), ‘I’ is the current in mA, ‘V’ is the voltage in mV and ‘P’ is the power in mW. In eqn (2), ‘A’ is the surface area of electrodes used in m2 and ‘CD’ is the current density in mA m−2. In eqn (3), ‘PD’ is the power density in mW m−2. In eqn (4), ‘Ci’ is the initial COD value in mg L−1 and ‘Co’ is the final value of COD in mg L−1. In eqn (5), ‘Ti’ is the initial TDS value in ppm and ‘To’ is the final value of TDS in ppm. In eqn (6), ‘Si’ is the initial salinity of seawater in ppt and ‘So’ is the final value of salinity after treatment in ppt. In eqn (7), ‘T’ is the hydraulic retention time. The voltage and current data were obtained from MDCs using a digital multimeter. The COD, TDS and salinity removal rates were measured using multimeters in the laboratory. For data analysis, MS Excel and Origin Pro software were used.
3 Results
3.1 Bioelectricity generation
All of the MDCs were able to generate power while effectively cleaning the wastewater. Each batch of the experiment was conducted for a total of 20 days. Measurements were taken every 8 hours, with the MDC running in the experiment. The effectiveness of MDCs with either Zn–Cu or carbon fiber cloth electrodes was evaluated by measuring their voltage and current output as well as their ability to remove COD and TDS. The effectiveness of the MDCs in desalinating saltwater was evaluated by measuring the reduction in salinity. Desalinating seawater and producing electricity at the same time is shown to be possible in experiments. As the day went on, nutritional depletion in the MDC caused power, voltage, current density, and power density to decrease.
3.1.1 Bioelectricity generation in MDC from textile wastewater. Different electrical properties obtained from the Zn–Cu electrode and carbon fiber cloth electrode MDC of textile wastewater are shown in Fig. 2 and 3, respectively, which indicates the bioelectricity generation rate from textile wastewater containing MDCs. Graphs showing the mean and standard deviation (n = 3) for the voltage and current measured at the textile wastewater MDC are provided. In the instance of the Zn–Cu electrode MDC, the rate of voltage generation from textile wastewater was adequate. Over the course of a day, the Zn–Cu electrode's rate of voltage generation rose. The voltage measured in the Zn–Cu electrode was typically between 0.4 V and 0.8 V during the time period (20 days). The voltage range was from 0.321 V to 0.903 V. Using a carbon fiber cloth electrode and textile wastewater MDC, researchers typically measured voltages between 0.28 V to 0.51 V. Voltages ranged from a high of 0.515 V to a low of 0.203 V. As the amount of organic matter in the textile wastewater decreased throughout the course of the experiment, the pace at which voltage was generated from the wastewater began to slow.
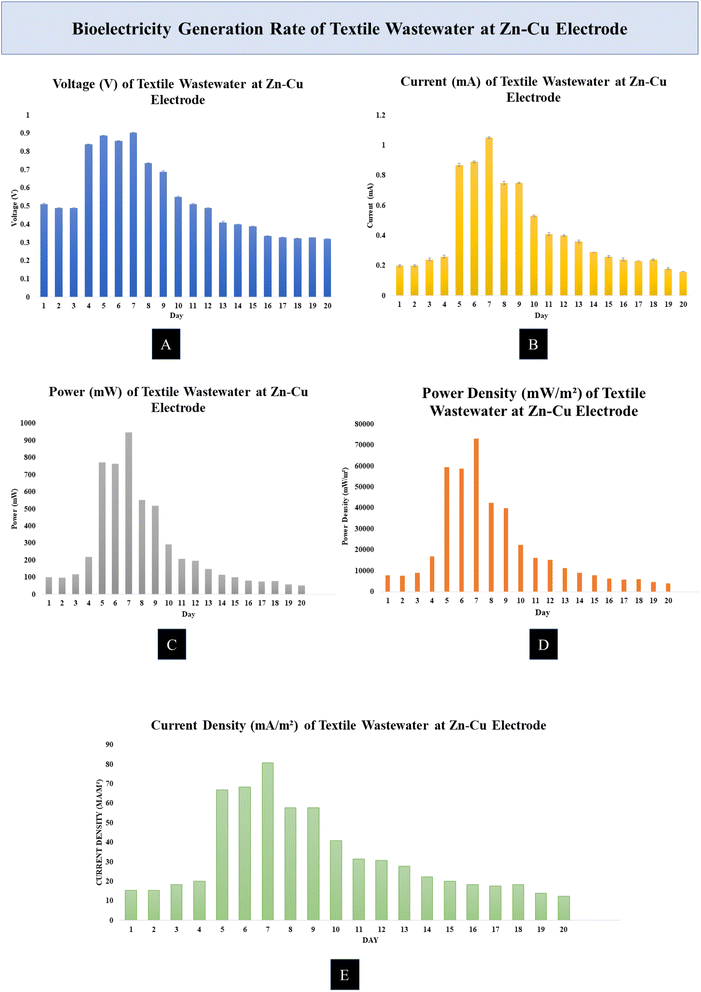 |
| Fig. 2 Bioelectricity generation rate of textile wastewater: (A) voltage (V), (B) current (mA), (C) power (mW), (D) power density (mW m−2), and (E) current density (mA m−2) at the Zn–Cu electrode MDC. | |
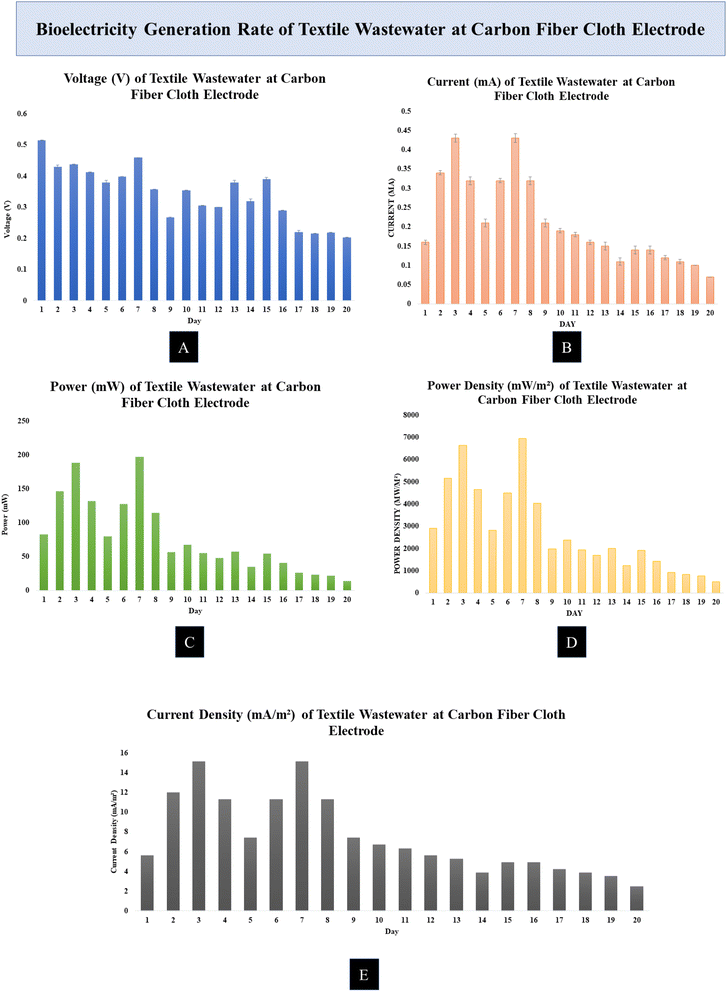 |
| Fig. 3 Bioelectricity generation rate of textile wastewater: (A) voltage (V), (B) current (mA), (C) power (mW), (D) power density (mW m−2), and (E) current density (mA m−2) at the carbon fiber cloth electrode MDC. | |
The rate of current generation of the MDC cell increased during the day. The Zn–Cu electrode with MDC produced a current of about 0.5 mA to 1 mA during the experiment. The range of values of the current generation was from 0.16 mA to 1.05 mA during the experimental time period. Using a carbon fiber cloth electrode, the value of the current generated by the textile wastewater electrode was measured to be around 0.4 mA to 0.18 mA. We measured a maximum of 0.43 mA and a minimum of 0.07 mA.
The Zn–Cu electrode had a high power output per unit time. The average power increase at the Zn–Cu electrode was 948.5 mW. The Zn–Cu electrode produced significantly more power on day two than on day one. The power output of the carbon fiber cloth electrode was rather low. The carbon fiber cloth electrode produced the most power, 197.37 mW. 14.21 mW was the minimum value.
The current generated in MDC was divided by the surface area of the electrode to determine the current density. The amount of current flowing through an electrode changes with its surface area. At the Zn–Cu electrode, a relatively high current density was measured. Most measurements were taken between 50 and 80 mA m−2. It points to the Zn–Cu electrode with MDC, generating a sizable amount of current. At the carbon fiber cloth electrode, the current density was typically between 5 and 11 mA m−2.
The power density was calculated by dividing the MDC output by the effective area of the electrode. The Zn–Cu electrode had 72951.45 mW m−2, the maximum power density, and the readings ranged from 40
000 to 90
000 mW m−2. After day 3, the power density of the Zn–Cu electrode began to rise, and it continued to rise until day 14. The maximum power density was measured at 6954.55 mW m−2 at the carbon fiber cloth electrode. Most of the readings from the carbon fiber cloth electrode ranged from 2900 to 6900 mW m−2.
3.1.2 Bioelectricity generation in MDC from tannery wastewater. Different electrical properties obtained from the Zn–Cu electrode and carbon fiber cloth electrode MDC of tannery wastewater are shown in Fig. 4 and 5, indicating the bioelectricity generation rate from tannery wastewater containing MDCs. Graphs showing the mean and standard deviation (n = 3) for the voltage and current measured at the tannery wastewater MDCs are provided. In the instance of the Zn–Cu electrode MDC, the rate of voltage generation from tannery wastewater was adequate. On day 8, the voltage generation rate at the Zn–Cu electrode began to decline after having grown over the previous days. The Zn–Cu electrode voltage was measured at 0.37 V to 0.6 V on the majority of days. Voltages ranged from a high of 0.634 V to a low of 0.314 V. However, the majority of the time, the voltage measured in the carbon fiber cloth electrode was between 0.227 V and 0.427 V. Voltages ranged from a high of 0.427 V to a low of 0.219 V.
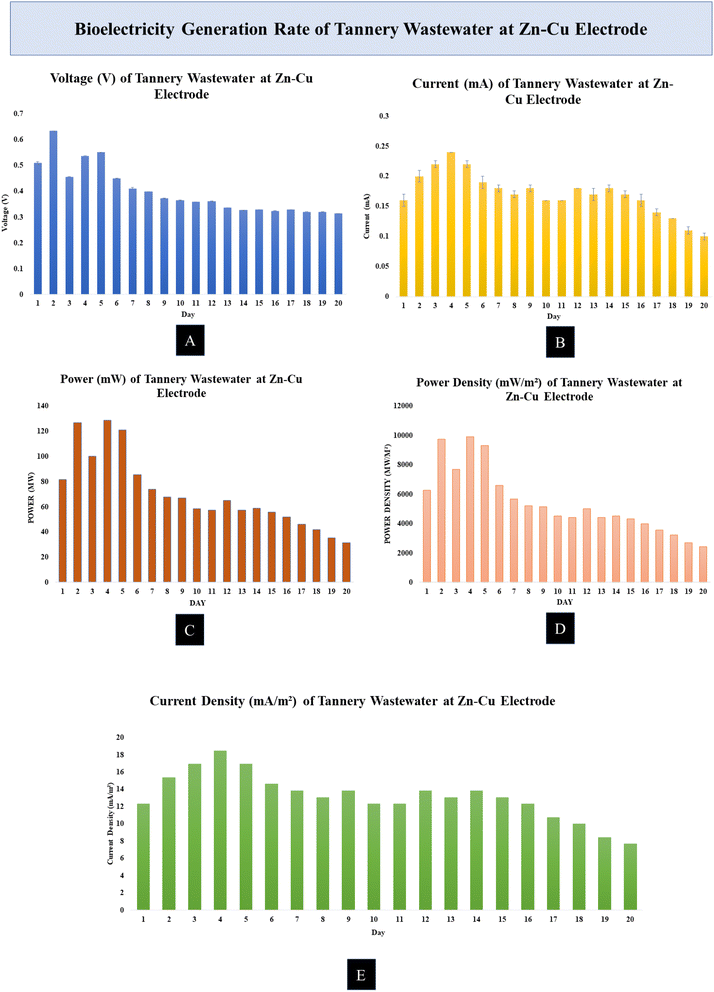 |
| Fig. 4 Bioelectricity generation rate of aannery wastewater: (A) voltage (V), (B) current (mA), (C) power (mW), (D) power density (mW m−2), and (E) current density (mA m−2) at the Zn–Cu electrode MDC. | |
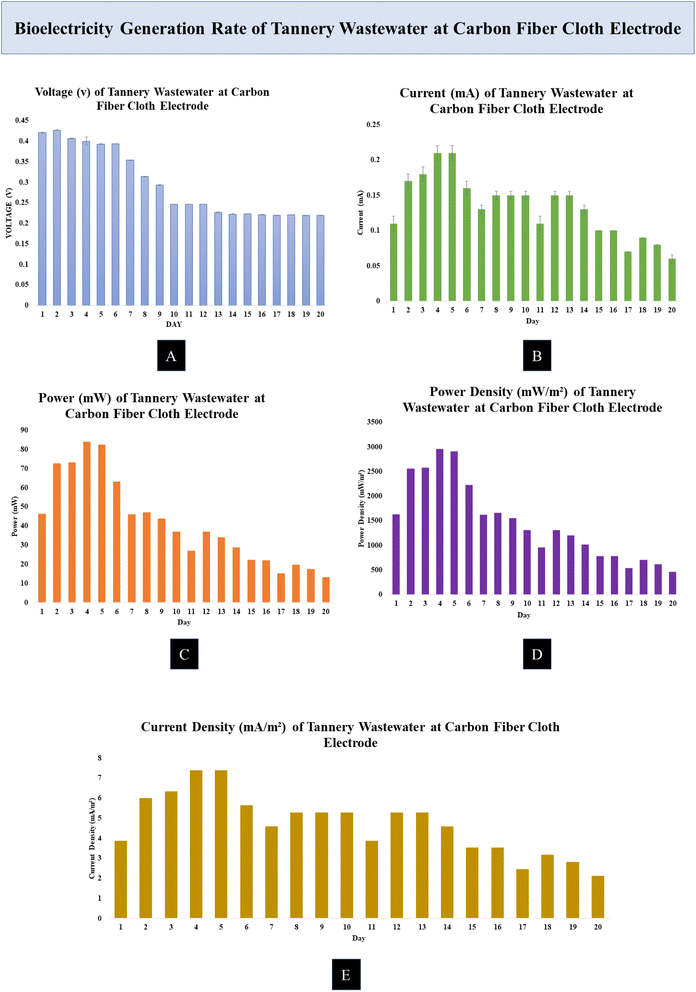 |
| Fig. 5 Bioelectricity generation rate of tannery wastewater: (A) voltage (V), (B) current (mA), (C) power (mW), (D) power density (mW m−2), and (E) current density (mA m−2) at the carbon fiber cloth electrode MDC. | |
Over the course of a day, the current generation rate of the MDC cell increased. The tannery wastewater was found to generate a current of 0.13–0.2 mA at the Zn–Cu electrode and 0.15–0.21 mA at the carbon fiber cloth electrode.
The Zn–Cu electrode produced a gain of about 128.88 mW. The Zn–Cu electrode produced a lot of power on day 2; however, production dropped off after day 9. The carbon fiber cloth electrode had a somewhat slow rate of power generation. The carbon fiber cloth electrode produced the most power, at 82.53 mW. The minimum value was 13.14 mW.
The Zn–Cu electrode was found to have a rather high current density. The majority of the time, the current density was between 10 and 18 mA m−2 at the Zn–Cu electrode and between 3 and 5 mA m−2 at the carbon fiber cloth electrode.
The Zn–Cu electrode had a maximum power density of 9916.13 mW m−2. The Zn–Cu electrode readings typically ranged from 4400 to 9900 mW m−2. The maximum power density was 2959.83 mW m−2 at the carbon fiber cloth electrode. Most of the readings taken with an electrode made of carbon fiber fabric fell within the range of 1300–2900 mW m−2.
3.2 Wastewater treatment efficiency
3.2.1 COD removal efficiency. After 20 days of MDC wastewater treatment, COD decrease was assessed. Monitoring COD removal from all MDCs throughout the operation assessed the wastewater treatment capabilities of the desalination cell. All wastewater samples demonstrated COD removal potential, supporting the idea that wastewater bacteria metabolize carbon sources to supply electrons. Experimental results suggest that current generation and COD reduction are compatible. All MDCs removed COD continuously.Fig. 6A shows the percentage of COD reduction from wastewater using MDC with Zn–Cu and carbon fiber electrodes. The graph shows that textile wastewater treatment removed the most COD. Also, the Zn–Cu electrode proved much more efficient in COD removal from both textile and tannery wastewater, with the highest removal efficiency of 65%. Though the COD removal rate is low, as only 2 L volume was used to conduct the experiment, it is not that insignificant. Also, the pollution level of wastewater in Bangladesh is much higher than that of other developed nations.
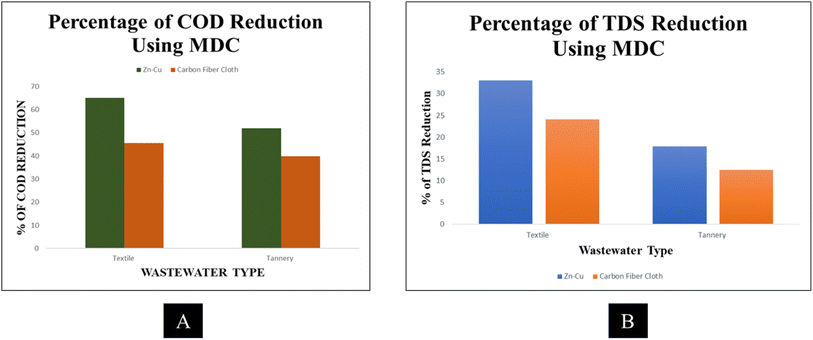 |
| Fig. 6 (A) Percentage of the COD removal rate from wastewater using an MDC and (B) percentage of TDS reduction from wastewater using an MDC. | |
3.2.2 TDS removal efficiency. The TDS elimination rate was assessed after 20 days of treatment. All MDCs reduced TDS. TDS decreased in all MDCs after 20 days of treatment.Fig. 6B illustrates the percentage of TDS removal by Zn–Cu and carbon fiber cloth electrodes from textile and tannery wastewaters using MDC. The textile wastewater treatment removed the most TDS. Tannery wastewater treatment reduced the lowest TDS. The Zn–Cu electrode removed 33% of wastewater TDS content most efficiently.
3.3 Desalination efficiency and desalination rate
After 20 days of treatment, salinity reduction efficiency was assessed. All MDCs removed saltwater saltiness. Salinity levels dropped significantly in all MDCs following 20 days of therapy.
Zn–Cu and carbon fiber cloth electrodes reduced salinity from MDC seawater, as seen in Fig. 7A. The MDC-containing textile wastewater reduced 85% of ocean salinity. The Zn–Cu electrodes reduced seawater salinity the best. Both electrodes (Zn–Cu and carbon fiber loth) satisfactorily removed salinity from seawater in MDC. The Zn–Cu electrode was the most efficient in reducing the salinity level concentration from the seawater.
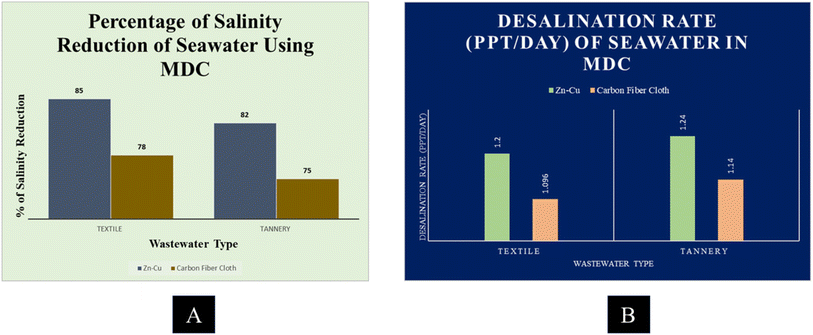 |
| Fig. 7 (A) Percentage of salinity reduction from seawater using an MDC and (B) desalination rate of seawater using an MDC. | |
The desalination rate was also measured at the end of 20 days of the operating period of the treatment process. Fig. 7B shows the graph of the desalination rate of all MDCs. It was observed that among all MDCs, the desalination rate was higher in tannery wastewater MDC containing Zn–Cu electrode and it was 1.24 ppt per day.
3.4 Polarization curve
Polarization curves show the voltage–current density relationships. It is used to study electrochemical fuel cells. The polarization curve shows the electrode functionality, electrochemical reaction speed, and efficacy. The electrochemical operations of a system can be revealed by its polarization curve. The fuel cell polarization curve has three distinctive areas. At low power concentrations, the cell potential decreases due to activation polarization; at moderate current densities, the cell potential decreases linearly with current; and at high current densities, the cell potential decrease deviates from this linear relationship due to more prominent concentration polarization. In Fig. S3,† these three areas are clearly stated. With the changes in obtained current densities, there was also a significant change in the voltage. The voltage increased or decreased depending on the changes in the current density of the MDC.
4 Discussion
There is a pressing demand for desalination facilities worldwide, including in Bangladesh. The problem of freshwater scarcity will eventually affect the entire planet. Since Bangladesh is prone to the effects of climate change, saline intrusion is a typical occurrence. The southern region of Bangladesh is being hit particularly hard by the rising water levels. Once again, Bangladesh lags behind wealthy countries in its wastewater management and treatment.28 The results of this investigation, however, suggest that the wastewater utilized in the experiment could be used to generate power. The goal of this study was to develop an MDC that could desalinate saltwater while also treating wastewater and producing power.
Few studies have documented real seawater desalination, with complex ion composition (monovalent and divalent ions) being crucial.5,26,27,29 The experiment in this study was conducted with real seawater. The salinity of seawater was successfully lowered by roughly 85% in this experiment. However, in a study conducted by R. Kalankesh et al. (2019),29 it was determined that the highest and lowest rates of desalination efficiency for water from the Caspian Sea and a solution containing 5 g L−1 of NaCl were 48% ± 1% and 65% ± 1%, respectively. Contrarily, Moruno et al. (2018)27 found that MDC achieved a maximum decrease in salinity of 78.6% ± 2.0%. In another research performed by Sevda and Abu-Reesh (2017),4 the desalination efficiency of seawater in MDC was 60.9%. Desalinating seawater to obtain freshwater is shown to be possible in this study. MDC accomplished three goals at once. It did more than just generate bioelectricity; it desalinated seawater and treated wastewater at the same time. While the current level of electricity generation is low, it has the potential to improve in the future. Because of its ability to control ohmic resistance, reduce energy consumption, and boost charge transfer efficiency and desalination rate,15 the ion exchange resin-based membrane has been produced and employed exclusively in this MDC. Ion exchange resin is inexpensive and widely available, making it a good long-term solution for poor nations like Bangladesh. Seawater contains calcium and magnesium ions, which can build up on the resin and cause scaling. This would cause the internal resistance of the cells to increase, blocking the passage of ions.15 From day one to day twenty, electricity production was measured using a multi-meter while an ion exchange resin membrane linked the anode and cathode. As the day wore on, the plant's ability to produce electricity decreased as nutrients were consumed. MDC is essentially an anaerobic treatment process due to the fact that bacteria grow in a chamber on an electrode when oxygen is not present. Bacteria in the wastewater oxidize organic molecules, transferring electrons to the cathode to generate electricity. Electricity is produced when electrons are transferred to the electrode.30,31 The MDC relies on the potential difference between the cathode and anode chambers to generate a current. Electricity production is proportional to the amount of electrons liberated by wastewater. As a result, the amount of power produced in the MDC changed as a function of the number of electrons created.32 After a period of time, less energy was generated since the microorganisms in the substrate had perished. Using dead microorganisms as a substrate in wastewater diminishes current and voltage. The reduction in COD, TDS, current, voltage, and power output is determined by the microbial activity on the substrate in the anode chamber and the electrolyte in the cathode chamber. The voltage generated by MDC increases with both the microbiological activity in the anode chamber and the electrolyte liberation activity in the cathode chamber.14
All MDCs were successful in producing electricity. This result was achieved by only treating 2 L of wastewater in MDC. Desalinating seawater, purifying wastewater, and producing bioelectricity using a Zn–Cu electrode were shown to be more effective than using a carbon fiber cloth electrode in this experiment. The Zn–Cu electrode in the MDC generated a significant quantity of current and voltage. Textile wastewater was shown to be significantly more effective in creating bioelectricity and desalinating seawater than municipal wastewater. The MDC also improved the rate at which COD and TDS were eliminated. Only one study found that MDC could reduce COD by more than 25%.2 Only 52% COD reduction was achieved in a research study by Luo et al. (2012).24 In contrast to a previous study in which only about 60% COD reduction was achieved by stacked MDC, this one produced close to 70% COD reduction. The TDS value likewise dropped when the wastewater was cleaned up. The Zn–Cu electrode achieved a greater TDS removal rate. The TDS reduction in the treated textile wastewater was greater than in the other. Treated tannery wastewater had the lowest removal rate because of its high TDS levels, which contained a variety of metals, components, etc. One study found that a multi-chamber MDC was able to remove 77% of COD and 85% of TDS.33 Clearance rates for both COD and TDS were reported to be 68%.34 This study thus removes COD and TDS more effectively than previous ones.
Most MDCs have recorded desalination efficiencies of at least 50%, and chemical oxygen demand (COD) is the most studied wastewater parameter in MDC.17 This study indicated that the desalination efficiency of both types of MDCs was greater than 50%, which is consistent with the theoretical data that is already available. Meng et al. (2014)2 observed that desalination efficiency was close to 46%. Qu et al. (2012)25 reported a desalination efficiency of around 37% in their investigation. However, the efficiency of desalination was 85% in this investigation. The pace at which saltwater is transformed into potable water was also quantified by measuring the desalination rate. This desalination rate basically indicates how much fresh water a certain cell can generate in a given amount of time. Desalination typically requires a lot of power.35 The desalination rate we measured was not very energy-intensive because it was collected without any further energy input on our part. This research outlined a simple, low-cost method of treating tons of wastewater, making it possible to deal with the massive volume of wastewater generated by factories. Nawaz et al. (2022)14 postulated that the initial rise in current and voltage can be traced back to the components easily digested by the mixed microorganisms present in the wastewater. When these quickly biodegradable substrates were used up, production levels dropped. Meanwhile, the degradation of more complex components led to a drop in current flow.30,36 Since textile wastewater contained more organic matter than tannery wastewater, it was found to be a more effective feedstock for bioelectricity generation. Power densities of over 8000 mW m−2 were attained with this MDC, which is a significant improvement over the 20.25 mW m−2 found in a previous study by Zamanpour et al. (2016).37
In addition, the polarization curve explains that dependable voltage/current curves require a stable environment in which the predetermined values for temperature, pressure, humidity, and flow rates are maintained throughout the duration of the test or tests. In unstable environments, the voltage/current characteristics could shift. The polarization curve in Fig. S3† is expected to exhibit a linear trend, typically showing a gradual decrease in voltage with increasing current density. The non-linear pattern, nevertheless, points to variations from the predicted behavior. Diffusion constraints or problems with insufficient electrolyte flow could cause this, as they can result in non-linear polarization curves, in addition to the deterioration of the electrodes, changes in pressure, temperature, or surface condition, etc. Future experiments will regulate and closely study these elements in order to omit these issues. It is possible that the fuel cell, in addition to the testing environment, will need some time to settle down. It may take up to 30 minutes for a fuel cell to stabilize following an adjustment to the current or voltage.38 However, the MDC method is not without its drawbacks. The proton exchange mechanism, which results in a reduced pH in the anode chamber and an increased pH in the cathode chamber, causes a pH imbalance.23,39 Additional research is required to effectively address this constraint.
The effluent used in this study, however, has been suggested as a potential energy source and waste treatment method. The rate of population growth in Bangladesh is extremely high. There has been a tremendous increase in the rate of urbanization and industrialization. These will cause an increase in industrial effluents, necessitating the use of substantial amounts of nonrenewable energy for wastewater treatment. Bangladesh can benefit appreciably from this MDC technology. While doing so, it will also generate bioelectricity from the wastewater it processes. The fact that desalination is technically feasible is crucial, as the people of Bangladesh, especially those in the southern regions, are in dire need of clean drinking water. This groundbreaking study holds the potential to renew faith in humanity's ability to persevere in the long run. There is a pressing demand for renewable energy sources in Bangladesh right now. The energy industry is feeling the strain of a growing population. Future energy management can only be achieved through the use of renewable energy sources. Utilizing potential bacteria in the treatment of waste organics, MDC generates electricity that is both renewable and environmentally friendly. Energy costs in Bangladesh could be reduced and new, low-cost methods of treating wastewater could be made possible by investigating microbial desalination cells. This research shows that MDCs can generate electricity from various types of wastewater while also cleaning the wastewater and desalinizing the seawater.
5 Conclusion
In this research, the microbial desalination cell technique reduced wastewater COD and TDS while generating energy. Wastewater already contains power-generating and COD-removing bacteria. As our dependence on nonrenewable energy sources grows, we will need new renewable energy sources in the coming decades. As populations grow, waste integration overwhelms natural systems. Bangladesh's water deficit requires this MDC's cheap, eco-friendly technology, along with affordable, fast, and efficient renewable energy and wastewater treatment. The main findings of this study reveal that an MDC using ion exchange resins can desalinate saltwater and generate bioelectricity while treating wastewater from various sources in Bangladesh. The study supports the idea. It made wastewater treatment and desalination cheaper. MDC employing an ion exchange resin membrane is cost-effective. Common ion exchange resin membrane MDC is affordable for countries like Bangladesh, but more testing is needed before using this technology.
Data availability
The data supporting this article have been included as part of the ESI.†
Author contributions
Sadia Sikder: idea generation, study design, methodology, visualization, data collection, calculation, data analysis, software, writing – original draft, Md. Toha: writing original draft, Mahmudul Hasan Sikder: methodology, data collection, Md. Mostafizur Rahman: conceptualization, reviewing, editing, supervision.
Conflicts of interest
The authors declare that they have no known competing financial interests or personal relationships that could have appeared to influence the work reported in this paper.
Acknowledgements
The funding for conducting this research has been provided by the Ministry of Science and Technology, Dhaka, Bangladesh, through the National Science and Technology (NST) Fellowship 2022–23. The authors greatly acknowledge the Environmental Chemistry Lab and Environmental Biology Lab, BUP, for the logistics support to carry out this research.
References
- S. Sevda, X. Dominguez-Benetton, K. Vanbroekhoven, H. De Wever, T. R. Sreekrishnan and D. Pant, High strength wastewater treatment accompanied by power generation using air cathode microbial fuel cell, Appl. Energy, 2013, 105, 194–206 CrossRef CAS.
- F. Meng, J. Jiang, Q. Zhao, K. Wang, G. Zhang and Q. Fan, et al., Bioelectrochemical desalination and electricity generation in microbial desalination cell with dewatered sludge as fuel, Bioresour. Technol., 2014, 157, 120–126 CrossRef CAS.
- Q. Ping, C. Zhang, X. Chen, B. Zhang, Z. Huang and Z. He, Mathematical Model of Dynamic Behavior of Microbial Desalination Cells for Simultaneous Wastewater Treatment and Water Desalination, Environ. Sci. Technol., 2014, 48(21), 13010–13019 CrossRef CAS.
- S. Sevda, H. Yuan, Z. He and I. M. Abu-Reesh, Microbial desalination cells as a versatile technology: Functions, optimization and prospective, Desalination, 2015, 371, 9–17 CrossRef CAS . Available from: https://www.sciencedirect.com/science/article/pii/S0011916415003422.
- S. Sevda, I. M. Abu-Reesh, H. Yuan and Z. He, Bioelectricity generation from treatment of petroleum refinery wastewater with simultaneous seawater desalination in microbial desalination cells, Energy Convers. Manage., 2017, 141, 101–107 CrossRef CAS.
- C. Y. Ma and C. H. Hou, Enhancing the water desalination and electricity generation of a microbial desalination cell with a three-dimensional macroporous carbon nanotube-chitosan sponge anode, Sci. Total Environ., 2019, 675, 41–50 CrossRef CAS PubMed.
- X. Cao, X. Huang, P. Liang, K. Xiao, Y. Zhou and X. Zhang, et al., A New Method for Water Desalination Using Microbial Desalination Cells, Environ. Sci. Technol., 2009, 43(18), 7148–7152 CrossRef CAS PubMed.
- H. Luo, P. E. Jenkins and Z. Ren, Concurrent Desalination and Hydrogen Generation Using Microbial Electrolysis and Desalination Cells, Environ. Sci. Technol., 2010, 45(1), 340–344 CrossRef PubMed.
- E. Elakkiya and M. Matheswaran, Comparison of anodic metabolisms in bioelectricity production during treatment of dairy wastewater in Microbial Fuel Cell, Bioresour. Technol., 2013, 136, 407–412 CrossRef CAS PubMed.
- V. G. Gude, B. Kokabian and V. Gadhamshetty, Beneficial Bioelectrochemical Systems for Energy, Water, and Biomass Production, J. Microb. Biochem. Technol., 2013, S6, 005 Search PubMed.
- A. D. Tharali, N. Sain and W. J. Osborne, Microbial fuel cells in bioelectricity production, Front. Life Sci., 2016, 9(4), 252–266 CrossRef CAS.
- B. Saba, A. D. Christy, Z. Yu and A. C. Co, Sustainable power generation from bacterio-algal microbial fuel cells (MFCs): An overview, Renewable Sustainable Energy Rev., 2017, 73, 75–84 CrossRef CAS.
- Y. Ye, H. H. Ngo, W. Guo, S. W. Chang, D. D. Nguyen and Y. Liu, et al., Microbial fuel cell for nutrient recovery and electricity generation from municipal wastewater under different ammonium concentrations, Bioresour. Technol., 2019, 292, 121992 CrossRef CAS PubMed . Available from: https://opus.lib.uts.edu.au/bitstream/10453/137229/1/Accepted%20Manuscript%20Microbial%20fuel%20cell%20for%20nutrient%20recovery%20and%20electricity%20generation%20%28BITE%20121992%29.pdf.
- A. Nawaz, I. ul Haq, K. Qaisar, B. Gunes, S. I. Raja and K. Mohyuddin, et al., Microbial fuel cells: Insight into simultaneous wastewater treatment and bioelectricity generation, Process Saf. Environ. Prot., 2022, 161, 357–373 CrossRef CAS . Available from: https://www.sciencedirect.com/science/article/pii/S095758202200252X.
- H. M. Saeed, G. A. Husseini, S. Yousef, J. Saif, S. Al-Asheh and A. A. Fara, et al., Microbial desalination cell technology: A review and a case study, Desalination, 2015, 359, 1–13 CrossRef CAS.
- K. S. Brastad and Z. He, Water softening using microbial desalination cell technology, Desalination, 2013, 309, 32–37 CrossRef CAS.
- A. Z. Imoro, M. Mensah and R. Buamah, Developments in the microbial desalination cell technology: A review, Water-Energy Nexus, 2021, 4, 76–87 CrossRef CAS.
- B. Zhang and Z. He, Improving water desalination by hydraulically coupling an osmotic microbial fuel cell with a microbial desalination cell, J. Membr. Sci., 2013, 441, 18–24 CrossRef CAS.
- Z. I. Abubakari, M. Mensah, R. Buamah and R. C. Abaidoo, Assessment of the electricity generation, desalination and wastewater treatment capacity of a plant microbial desalination cell (PMDC), Int. J. Energy Water Resour., 2019, 3(3), 213–218 CrossRef.
- H. Jingyu, D. Ewusi-Mensah and E. Norgbey, Microbial desalination cells technology: a review of the factors affecting the process, performance and efficiency, Desalin. Water Treat., 2017, 87, 140–159 CrossRef.
- M. Tawalbeh, A. Al-Othman, K. Singh, I. Douba, D. Kabakebji and M. Alkasrawi, Microbial desalination cells for water purification and power generation: A critical review, Energy, 2020, 209(C), 118493 CrossRef , Available from: https://ideas.repec.org/a/eee/energy/v209y2020ics0360544220316017.html.
- S. Rahman, T. Jafary, A. Al-Mamun, M. S. Baawain, M. R. Choudhury and H. Alhaimali, et al., Towards upscaling microbial desalination cell technology: A comprehensive review on current challenges and future prospects, J. Cleaner Prod., 2021, 288, 125597 CrossRef CAS.
- H. Luo, P. Xu, P. E. Jenkins and Z. Ren, Ionic composition and transport mechanisms in microbial desalination cells, J. Membr. Sci., 2012, 409–410, 16–23 CrossRef CAS.
- H. Luo, P. Xu, T. M. Roane, P. E. Jenkins and Z. Ren, Microbial desalination cells for improved performance in wastewater treatment, electricity production, and desalination, Bioresour. Technol., 2012, 105, 60–66 CrossRef CAS PubMed.
- Y. Qu, Y. Feng, X. Wang, J. Liu, J. Lv and W. He, et al., Simultaneous water desalination and electricity generation in a microbial desalination cell with electrolyte recirculation for pH control, Bioresour. Technol., 2012, 106, 89–94 CrossRef CAS.
- H. Alhimali, T. Jafary, A. Al-Mamun, M. S. Baawain and G. R. Vakili-Nezhaad, New insights into the application of microbial desalination cells for desalination and bioelectricity generation, Biofuel Res. J., 2019, 6(4), 1090–1099 CrossRef.
- F. L. Moruno, J. Carlos, C. Santoro, P. Atanassov, J. M. Cerrato and C. G. Arges, Investigation of patterned and non-patterned poly(2,6-dimethyl 1,4-phenylene) oxide based anion exchange membranes for enhanced desalination and power generation in a microbial desalination cell, Solid State Ionics, 2018, 314, 141–148 CrossRef CAS PubMed.
- K. Mostakim, M. A. Arefin, M. T. Islam, K. M. Shifullah and M. A. Islam, Harnessing energy from the waste produced in Bangladesh: evaluating potential technologies, Heliyon, 2021, 7(10), e08221 CrossRef CAS PubMed.
- L. R. Kalankesh, S. Rodríguez-Couto and M. A. Zazouli, Desalination and power generation of caspian sea by applying new designed microbial desalination cells in batch operation mode, Environ. Prog. Sustainable Energy, 2019, 38(5), 13205 CrossRef.
- S. Sikder and Md M. Rahman, Efficiency of microbial fuel cell in wastewater (municipal, textile and tannery) treatment and bioelectricity production, Case Stud. Chem. Environ. Eng., 2023, 8(100421), 100421 CrossRef CAS . Available from: https://www.sciencedirect.com/science/article/pii/S2666016423001263.
- T. C. Saha, A. T. Protity, F. T. Zohora, M. Shaha, I. Ahmed and E. Barua, et al., Microbial Fuel Cell (MFC) Application for Generation of Electricity from Dumping Rubbish and Identification of Potential Electrogenic Bacteria, Adv. Ind. Biotechnol., 2019, 2(1), 1–8 Search PubMed.
- C. Koch, B. Korth and F. Harnisch, Microbial ecology-based engineering of Microbial Electrochemical Technologies, Microb. Biotechnol., 2017, 11(1), 22–38 CrossRef PubMed.
- H. Pradhan, S. C. Jain and M. M. Ghangrekar, Simultaneous Removal of Phenol and Dissolved Solids from Wastewater Using Multichambered Microbial Desalination Cell, Appl. Biochem. Biotechnol., 2015, 177(8), 1638–1653 CrossRef CAS PubMed.
- O. A. Shinde, A. Bansal, A. Banerjee and S. Sarkar, Bioremediation of steel plant wastewater and enhanced electricity generation in microbial desalination cell, Water Sci. Technol., 2018, 77(8), 2101–2112 CrossRef CAS PubMed.
- G. Caputo and A. Giaconia, Membrane technologies for solar-desalination plants, Membr. Clean Renew. Power Appl., 2014, 1, 347–364 Search PubMed.
- A. S. Mathuriya and V. N. Sharma, Bioelectricity production from various wastewaters through microbial fuel cell technology, J. Biochem. Technol., 2009, 2(1), 133–137 CAS.
- M. K. Zamanpour, H. R. Kariminia and M. Vosoughi, Electricity generation, desalination and microalgae cultivation in a biocathode-microbial desalination cell, J. Environ. Chem. Eng., 2017, 5(1), 843–848 CrossRef.
- C. Spiegel, Polarization Curves, 2017, available from: https://www.fuelcellstore.com/blog-section/polarization-curves Search PubMed.
- X. Chen, P. Liang, Z. Wei, X. Zhang and X. Huang, Sustainable water desalination and electricity generation in a separator coupled stacked microbial desalination cell with buffer free electrolyte circulation, Bioresour. Technol., 2012, 119, 88–93 CrossRef CAS PubMed.
|
This journal is © The Royal Society of Chemistry 2024 |
Click here to see how this site uses Cookies. View our privacy policy here.