DOI:
10.1039/D3SC04431A
(Edge Article)
Chem. Sci., 2024,
15, 6178-6183
Visible-light-driven alkene dicarboxylation with formate and CO2 under mild conditions†
Received
23rd August 2023
, Accepted 18th March 2024
First published on 21st March 2024
Abstract
Low-cost formate salt was used as the reductant and part of the carboxyl source in a visible-light-driven dicarboxylation of diverse alkenes, including simple styrenes. The highly competing hydrocarboxylation side reaction was successfully overridden. Good yields of products were obtained under mild reaction conditions at ambient temperature and pressure of CO2. The dual role of formate salt may stimulate the discovery of a range of new transformations under mild and friendly conditions.
Introduction
Over the past decade, great progress has been made in the catalytic utilization of carbon dioxide (CO2) using visible light.1 However, many of these transformations require the use of stoichiometric reductant. Recently, we developed an arylcarboxylation of alkenes with CO2 using formate salt as a low-cost terminal reductant and also as part of the CO2 source.2 In this study, it was first realized that the CO2 radical anion (CO2˙−) (E1/2(CO2/CO2˙−) = −2.2 V vs. SCE),3 readily available from the formate via hydrogen atom transfer (HAT), could be used as a potent reductive intermediate for direct reduction of substrates in visible-light-induced organic synthesis, avoiding the need to generate a highly reductive photocatalyst (PC). This discovery has been employed in a series of photocatalytic reactions involving challenging reduction of substrates recently.4–6 Moreover, in the control experiments of this work, we also discovered dicarboxylation and hydrocarboxylation of alkene 1a in the absence of an aryl halide (Scheme 1a).2 Afterwards, the groups of Jui,4a Wickens,7 Li,8 and Mita9 also proved that CO2˙− generated from formate could act as a source of carboxyl via Giese-type radical addition to alkenes and (hetero)aromatics to deliver hydrocarboxylation reactions.10,11 Since formate could potentially be produced from CO2, e.g. via hydrogenation, photoreduction and electroreduction,12 the use of formate for the dicarboxylation of alkenes to produce diacids can be considered as an attractive indirect method for CO2 fixation (Scheme 1b).13
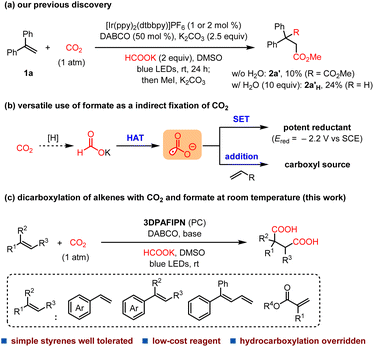 |
| Scheme 1 The development of dicarboxylation of alkenes with formate and CO2. | |
Diacids such as succinic acid derivatives are important core structures of many bioactive molecules and useful monomers for polymers.14 The production of diacids using CO2 as the carboxyl source is a sustainable strategy, but only limited methods have been developed to date.15 Traditionally, electrochemical dicarboxylation of alkenes with CO2 has been investigated, but these processes generally require a sacrificial anode.15,16 In addition, Martin and co-workers reported a Ni-catalyzed site-selective dicarboxylation of 1,3-dienes with CO2, which used stoichiometric Mn as the reductant.17 In 2021, the group of Yu reported an elegant dicarboxylation of alkenes with CO2via a sequential single electron transfer (SSET) process, in which the reduction of the alkene substrate to a radical anion intermediate was the key step.18 Recently, the same group disclosed a remote dicarboxylation of alkene and benzylic C(sp3)–H bond via an intramolecular HAT.19 However, amine terminal reductants were necessary for these two transformations. Given the challenge of direct CO2 reduction3,15,20 and the low cost of the formate salt that possessed a dual role in our preliminary results of dicarboxylation (Scheme 1a and b),2 we envisioned using the formate for alkene dicarboxylation to obtain diacids in a redox-economical and practical manner.21
Herein, we report a visible-light-induced dicarboxylation of diverse alkenes with CO2 using formate as a low-cost reductant and part of the carboxyl source. Notably, simple styrenes were well tolerated, and the reaction could be performed under mild conditions at ambient temperature and pressure of CO2. Importantly, the highly competing hydrocarboxylation side reaction was successfully overridden.
Results and discussion
Our investigation began with using 1,1-diphenylethylene (1a) as the model substrate, which was treated with 30 W blue LED irradiation in the presence of a PC and an atmospheric pressure of CO2 (Table 1). After extensive investigation of the reaction conditions, the desired dicarboxylation product 2a was generated in an 88% isolated yield using 3DPAFIPN as the PC in the presence of the HAT catalyst DABCO with HCOOK as the terminal reductant and Cs2CO3 and K3PO4 as the cooperative bases in DMSO at ambient temperature (entry 1).22 No product was found in the absence of either visible light or the PC, indicating the reaction was a photocatalytic reaction (entries 2 and 3). Importantly, HCOOK was essential for the reaction (entry 4). Surprisingly, in the absence of DABCO, a modest yield of the desired product 2a was obtained along with some hydrocarboxylation side-product 2aH, suggesting that more than one HAT process might be involved in this reaction (entry 5). Interestingly, only the hydrocarboxylation product was obtained under a nitrogen atmosphere without adding CO2 (entry 6), suggesting that HCOOK was also one of the carboxyl sources. Moreover, the addition of suitable bases was important to achieve a high yield of the desired product (entries 7–9, also see ESI† for screening of other bases) and avoid the generation of hydrocarboxylation side-product 2aH as well (entry 9), possibly by inhibiting direct protonation of the benzylic anion of the reaction intermediate. Other formates such as HCOONa were also used, but they were less effective than HCOOK (entry 10). Other HAT catalysts such as quinuclidine were also evaluated, but they were inferior to DABCO (entry 11).23 Other PCs such as 4DPAIPN, 4CzIPN and [Ir(ppy)2(dtbbpy)]PF6 were also tested, but worse results were obtained (entries 12–14, also see ESI† for more PC structures). The exact reason for the disparity in photocatalyst performance is not clear at present. One possible reason for this might be that 3DPAFIPN has a strong reduction potential (Ered(PC*/PC˙−) = −1.59 V vs. SCE in MeCN for 3DPAFIPN) to facilitate the reduction of the benzylic radical of the reaction intermediate.24 In addition, lower yields were delivered while reducing the loading of 3DPAFIPN (entries 15 and 16). Finally, DMSO was the best solvent of all those used such as THF and DMA (entries 17 and 18).
Table 1 Optimization of reaction conditionsa
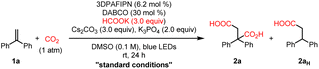
|
Entry |
Deviations from standard conditions |
2a yield [%] |
2aH yield [%] |
Reaction conditions: 1a (0.2 mmol), 3DPAFIPN (6.2 mol%), DABCO (0.06 mmol), Cs2CO3 (0.6 mmol), K3PO4 (0.4 mmol), HCOOK (0.6 mmol) in DMSO (2 mL), 1 atm CO2, 30 W blue LEDs, rt (25–33 °C), 24 h; then treated with 2 mL of HCl (2 N). Yield was determined by 1H NMR with CH2Br2 as the internal standard.
Isolated yield was 88%.
[Ir(ppy)2(dtbbpy)]PF6 (5 mol%). 3DPAFIPN = 2,4,6-tris(diphenylamino)-5-fluoroisophthalonitrile; DABCO = triethylenediamine.
|
1 |
None |
91%b |
— |
2 |
In the dark |
— |
— |
3 |
w/o 3DPAFIPN |
— |
— |
4 |
w/o HCOOK |
— |
— |
5 |
w/o DABCO |
32% |
28% |
6 |
w/o CO2 (N2 atmosphere) |
— |
76% |
7 |
w/o K3PO4 |
40% |
12% |
8 |
w/o Cs2CO3; K3PO4 (3 equiv.) |
44% |
50% |
9 |
w/o Cs2CO3 and K3PO4 |
11% |
66% |
10 |
HCOONa instead of HCOOK |
70% |
— |
11 |
Quinuclidine instead of DABCO |
40% |
— |
12 |
4DPAIPN instead of 3DPAFIPN |
65% |
— |
13 |
4CzIPN instead of 3DPAFIPN |
39% |
— |
14c |
[Ir(ppy)2(dtbbpy)]PF6 |
7% |
— |
15 |
3DPAFIPN (2.0 mol%) |
70% |
— |
16 |
3DPAFIPN (4.0 mol%) |
86% |
— |
17 |
THF instead of DMSO |
16% |
— |
18 |
DMA instead of DMSO |
17% |
— |
With the optimized reaction conditions in hand, we first examined this protocol with a variety of 1,1-disubstituted ethylenes (Table 2). Good to excellent yields of desired dicarboxylation products were obtained with model substrate 1a and mono- or di-substituted 1,1-diarylethylenes bearing a list of functional groups at ortho-, meta- or para-positions of the phenyl groups (2a–2k). Notably, a 6 mmol scale reaction with 1a also provided 85% yield of desired product. It is worth mentioning that it is generally recommended to set up the reaction by weighing the chemical in a glovebox to reduce the possible hydrocarboxylation side product, but setting-up the reaction outside the glovebox could also deliver similar results (89% yield with 1a) if the reactants were kept anhydrous (see ESI†). Both electron-donating methyl (2b, 2d, 2f, and 2g) and methoxy (2h, 2i, and 2k) substituents and electron-withdrawing fluoro (2c and 2j) and trifluoromethyl (2e) ones were tolerated. Moreover, heteroaryl containing substrates were also compatible with this method, though lower yields were obtained (2l–2n). Other di-substituted ethylenes (2o, 2p, and 2q) and even a tri-substituted one (2r) were also viable to deliver acceptable yields of products. Note that the reaction with a diene substrate (1s) would give both 3,4-dicarboxylation and 1,4-dicarboxylation products. In addition, good results were obtained with methacrylates (2t and 2u), although only a modest yield was obtained with a 2-benzylacrylate (2v). Of note, only the hydrocarboxylation product was isolated with an acrylamide substrate such as 2-benzyl-N-methyl-N-phenylacrylamide.
Table 2 Scope of 1,1-disubstituted ethylenes and acrylatesa
Reaction conditions: 1 (0.2 mmol), 3DPAFIPN (6.2 mol%), DABCO (0.06 mmol), Cs2CO3 (0.6 mmol), K3PO4 (0.4 mmol), HCOOK (0.6 mmol) in DMSO (2 mL), 1 atm CO2, 30 W blue LEDs, rt (25–33 °C), 24 h; then treated with 2 mL of HCl (2 N). Isolated yields.
Yield of 6 mmol scale reaction (52 h).
The product was methylated with SOCl2/MeOH before isolation.
Major product 3,4-dicarboxylation product (2sa, 37%) displayed; minor product: 1,4-dicarboxylation product (2sb, 17%).
|
|
We then moved on to investigate the scope of the more challenging simple styrenes, leading to generally good yields with a variety of substituted styrenes regardless of the electronic properties and the position of the substituent on the aromatic ring. It should be noted that in Zhu's work, only a very narrow scope of simple styrenes were tolerated with low yields.22 As shown in Table 3, the un-substituted styrene 3a and the ortho-substituted styrenes with electron-donating methyl (3b) and methoxyl (3c) groups proceeded smoothly and gave good yields of the desired products. Notably, the yield of 4c could be increased to 94% by slightly elevating the reaction temperature to 40–45 °C. Moderate yield was obtained with substrate 3d bearing an ortho-chloro group, since some de-chlorination occurred. In addition, both electron donating (4e) and withdrawing (4f and 4g) groups were well tolerated at the meta-position. Good results were also obtained with substrates containing several representative substituents at the para-position of the phenyl ring (4h–4l). Finally, heteroaryl containing substrates were also tested, but the yields were much lower (4m and 4n). It is noteworthy that the hydrocarboxylation side product was not detected or only in trace amounts, and only substrate 3g led to 6% of the hydrocarboxylation side product.
Table 3 Scope of simple styrenesa
Reaction conditions: 3 (0.2 mmol), 3DPAFIPN (6.2 mol%), DABCO (0.06 mmol), Cs2CO3 (0.6 mmol), K3PO4 (0.4 mmol), HCOOK (0.6 mmol) in DMSO (2 mL), 1 atm CO2, 30 W blue LEDs, rt (25–33 °C), 24 h; then treated with 2 mL of HCl (2 N). Isolated yields.
Reaction temperature: 40–45 °C.
Desired product displayed; de-chlorination product (18% for 4d, 16% for 4f) not shown.
About 6% hydrocarboxylation side product.
The product was methylated with SOCl2/MeOH before isolation.
|
|
To obtain insights into the reaction mechanism, several control experiments were then carried out (Scheme 2). Ring-opening product 6 was afforded in the radical clock experiment, indicating a benzyl radical might be generated after CO2˙− addition to the alkene (Scheme 2a). When the reaction was run in the presence of D2O, hydrocarboxylation product 2aH-DH was produced instead of dicarboxylation with traces of reduction product 2aHH, indicating the formation of a benzylic anion intermediate and that the formation of anions at the alkene terminal position was trivial (Scheme 2b(i)). Similar results were obtained when the reaction was carried out without the addition of CO2 gas (Scheme 2b(i)). The low deuteration ratio at the benzylic position in these two reactions (49% and 32%, respectively) might suggest that a HAT process between the benzylic radical and formate was also involved except the protonation of the benzylic anion under current reaction conditions. In addition, four possible dicarboxylation products were obtained when using 13C-labelled formate (i.e. commercially available H13COONa),25 suggesting that formate was also part of the carboxyl source (Scheme 2c). Moreover, no carboxylation products were detected when the reaction was performed using a stoichiometric amount of DABCO without formate, indicating that the reduced 3DPAFIPN could not reduce the substrate 1a or CO2 to initiate the reaction (Scheme 2d). Furthermore, the Stern–Volmer quenching experiments showed that the activated catalyst (PC*) was mainly quenched by DABCO, but it could also be slightly quenched by HCOOK (see ESI†). Finally, a one-step procedure was developed for the synthesis of nervous system drug phensuximide (Scheme 2e) to demonstrate the application of the dicarboxylation product.
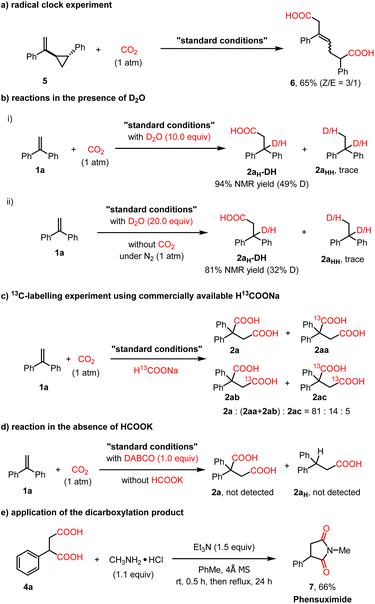 |
| Scheme 2 Mechanistic study experiments and product application. | |
Based on the above mechanistic studies, a possible catalytic cycle was proposed (Scheme 3). Upon blue light irradiation, the excited PC* (I) is generated and then quenched by DABCO to form PC˙− (II) (E1/2(PC*/PC˙−) = +1.09 V vs. SCE in MeCN)24 and the radical cation of DABCO (III). A HAT between radical III and HCO2− produces CO2˙− and cation IV. Alternatively, CO2˙− can be partially generated by a HAT process between HCO2− and
that is produced by reducing PC* with HCO2−
,4b considering the results of Stern–Volmer quenching experiments with formate (see Fig. S5† of SI) and the fact that some product could be obtained without DABCO (see entry 5 in Table 1). Subsequently, CO2˙− may reduce the substrate (Eo = −2.25 V vs. SCE for 1a)26 to give radical anion V, considering that product 2a was the major product in the 13C-labelling experiment of Scheme 2c. However, the direct addition of CO2˙− to the double bond of 1a to afford intermediate VI is more likely based on the results in Scheme 2b. In this scenario, a potential facile electron transfer/equilibration between 13CO2˙− and the excess CO2 gas may account for the observation of 2a as the main product in Scheme 2c.27 Moreover, since the reduction of simple styrenes (E1/2 = −2.58 V vs. SCE in DMF for 3a)28 is more demanding than 1a, direct CO2˙− addition is possibly the predominant pathway for substrates in Table 3. The radical intermediate VI was then reduced by PC˙− (II) to give the anion VII, which undergoes nucleophilic attack of CO2 to afford the final product 2a after acidification of the salt intermediate VIII.
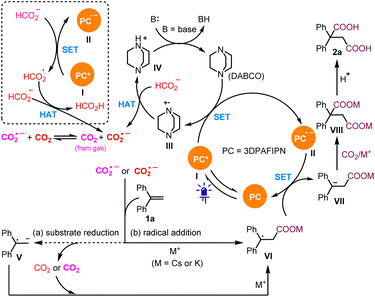 |
| Scheme 3 Proposed catalytic cycle. | |
Conclusions
In conclusion, we have developed a visible-light-driven alkene dicarboxylation using formate and CO2, overriding the highly competing hydrocarboxylation side reaction successfully. Good yields of products were obtained with diverse alkenes including simple styrenes under mild reaction conditions at ambient temperature. The dual role of the low-cost formate as a reductant and the C1 source may open up the discovery of a range of new transformations including indirect utilization of CO2 under mild and friendly conditions.
Data availability
Experimental procedures, characterisation data, and NMR spectra for new compounds can be found in the ESI.†
Author contributions
F. Zhang performed the experiments and developed the method. X. Wu, P. Gao and S. Ai prepared some of the substrates and catalyst. H. Zhang and Z. Li repeated and checked the results of some of the reactions. G. Li conceived the method, directed the project and wrote the manuscript with the feedback from the other authors.
Conflicts of interest
There are no conflicts to declare.
Acknowledgements
We gratefully acknowledge the financial support from NSFC (Grant No. 22022111, 21871257), and the Fundamental Research Funds for the Central Universities (22X010201631).
Notes and references
-
(a) Y. Cao, X. He, N. Wang, H.-R. Li and L.-N. He, Chin. J. Chem., 2018, 36, 644 CrossRef CAS;
(b) F. Tan and G. Yin, Chin. J. Chem., 2018, 36, 545 CrossRef CAS;
(c) Z. Zhang, J.-H. Ye, T. Ju, L. L. Liao, H. Huang, Y. Y. Gui, W. J. Zhou and D.-G. Yu, ACS Catal., 2020, 10, 10871 CrossRef CAS;
(d) X. He, L.-Q. Qiu, W.-J. Wang, K.-H. Chen and L.-N. He, Green Chem., 2020, 22, 7301 RSC;
(e) Z. Fan, Z. Zhang and C. Xi, ChemSusChem, 2020, 13, 6201 CrossRef CAS PubMed;
(f) M. Schmalzbauer, T. D. Svejstrup, F. Fricke, P. Brandt, M. J. Johansson, G. Bergonzini and B. König, Chem, 2020, 6, 2658 CrossRef CAS;
(g) J.-H. Ye, T. Ju, H. Huang, L.-L. Liao and D.-G. Yu, Acc. Chem. Res., 2021, 54, 2518 CrossRef CAS PubMed;
(h) B. Cai, H. W. Cheo, T. Liu and J. Wu, Angew. Chem., Int. Ed., 2021, 60, 18950 CrossRef CAS PubMed;
(i) J. Davies, J. R. Lyonnet, D. P. Zimin and R. Martin, Chem, 2021, 7, 2927 CrossRef CAS;
(j) S. Wang and B. Konig, Angew. Chem., Int. Ed., 2021, 60, 21624 CrossRef CAS PubMed;
(k) G. Bertuzzi, A. Cerveri, L. Lombardi and M. Bandini, Chin. J. Chem., 2021, 39, 3116 CrossRef CAS;
(l) J. Jung and S. Saito, Synthesis, 2021, 53, 3263 CrossRef CAS;
(m) Q. Dou, T. Wang, S. Li, L. Fang, H. Zhai and B. Cheng, Chin. J. Org. Chem., 2022, 42, 4257 CrossRef CAS;
(n) Y. Gao, H. Wang, Z. Chi, L. Yang, C. Zhou and G. Li, CCS Chem., 2022, 4, 1565 CrossRef CAS;
(o) Y. Pan, X. Meng, Y. Wang and M. He, Chin. J. Org. Chem., 2023, 43, 1416 CrossRef CAS;
(p) J. Majhi and G. A. Molander, Angew. Chem., Int. Ed., 2023, 63, e202311853 CrossRef PubMed;
(q) W. Xiao, J. Zhang and J. Wu, ACS Catal., 2023, 13, 15991 CrossRef CAS.
- H. Wang, Y. Gao, C. Zhou and G. Li, J. Am. Chem. Soc., 2020, 142, 8122 CrossRef CAS PubMed.
- W. H. Koppenol and J. D. Rush, J. Phys. Chem., 1987, 91, 4429 CrossRef CAS.
- For direct reduction of substrates with CO2˙− generated from formate:
(a) C. M. Hendy, G. C. Smith, Z. Xu, T. Lian and N. T. Jui, J. Am. Chem. Soc., 2021, 143, 8987 CrossRef CAS PubMed;
(b) A. F. Chmiel, O. P. Williams, C. P. Chernowsky, C. S. Yeung and Z. K. Wickens, J. Am. Chem. Soc., 2021, 143, 10882 CrossRef CAS PubMed;
(c) M. W. Campbell, V. C. Polites, S. Patel, J. E. Lipson, J. Majhi and G. A. Molander, J. Am. Chem. Soc., 2021, 143, 19648 CrossRef CAS PubMed;
(d) L.-L. Liao, L. Song, S.-S. Yan, J.-H. Ye and D.-G. Yu, Trends Chem., 2022, 4, 512 CrossRef CAS;
(e) Z.-Y. Bo, S.-S. Yan, T.-Y. Gao, L. Song, C.-K. Ran, Y. He, W. Zhang, G.-M. Cao and D.-G. Yu, Chin. J. Catal., 2022, 43, 2388 CrossRef CAS;
(f) J. H. Ye, P. Bellotti, C. Heusel and F. Glorius, Angew. Chem., Int. Ed., 2022, 61, e202115456 CrossRef CAS PubMed;
(g) M. Zhang, L. Yang, C. Zhou, L. Fu and G. Li, Asian J. Org. Chem., 2022, 11, e202200087 CrossRef CAS;
(h) P. Xu, X. Y. Wang, Z. Wang, J. Zhao, X. D. Cao, X. C. Xiong, Y. C. Yuan, S. Zhu, D. Guo and X. Zhu, Org. Lett., 2022, 24, 4075 CrossRef CAS PubMed;
(i) C. Liu, N. Shen and R. Shang, Nat. Commun., 2022, 13, 354 CrossRef CAS PubMed;
(j) C. Zhou, X. Wang, L. Yang, L. Fu and G. Li, Green Chem., 2022, 24, 6100 RSC;
(k) B. Wang, C. T. Wang, X. S. Li, W. H. Sun, X. Y. Liu and Y.-M. Liang, Org. Chem. Front., 2023, 10, 3341 RSC;
(l) L. Zeng, J. H. Qin, G. F. Lv, M. Hu, Q. Sun, X. H. Ouyang, D. L. He and J. H. Li, Chin. J. Chem., 2023, 41, 1921 CrossRef CAS.
- For previous studies on using the formate as a reductant for PC rather than the substrate to produce highly reductive PC:
(a) J. D. Nguyen, E. M. D'Amato, J. M. Narayanam and C. R. Stephenson, Nat. Chem., 2012, 4, 854 CrossRef CAS PubMed;
(b) E. H. Discekici, N. J. Treat, S. O. Poelma, K. M. Mattson, Z. M. Hudson, Y. Luo, C. J. Hawker and J. Read de Alaniz, Chem. Commun., 2015, 51, 11705 RSC;
(c) H. Wang and N. T. Jui, J. Am. Chem. Soc., 2018, 140, 163 CrossRef CAS PubMed;
(d) A. J. Boyington, C. P. Seath, A. M. Zearfoss, Z. Xu and N. T. Jui, J. Am. Chem. Soc., 2019, 141, 4147 CrossRef CAS PubMed;
(e) D. B. Vogt, C. P. Seath, H. Wang and N. T. Jui, J. Am. Chem. Soc., 2019, 141, 13203 CrossRef CAS PubMed.
- For generation of CO2˙−via hydride-transfer reduction and HAT: S.-S. Yan, S.-H. Liu, L. Chen, Z.-Y. Bo, K. Jing, T.-Y. Gao, B. Yu, Y. Lan, S.-P. Luo and D.-G. Yu, Chem, 2021, 7, 3099 CAS.
-
(a) S. N. Alektiar and Z. K. Wickens, J. Am. Chem. Soc., 2021, 143, 13022 CrossRef CAS PubMed;
(b) M. Mikhael, S. N. Alektiar, C. S. Yeung and Z. K. Wickens, Angew. Chem., Int. Ed., 2023, 62, e202303264 CrossRef CAS PubMed;
(c) S. N. Alektiar, J. Han, Y. Dang, C. Z. Rubel and Z. K. Wickens, J. Am. Chem. Soc., 2023, 145, 10991 CrossRef CAS PubMed.
-
(a) Y. Huang, J. Hou, L.-W. Zhan, Q. Zhang, W.-Y. Tang and B.-D. Li, ACS Catal., 2021, 11, 15004 CrossRef CAS;
(b) Y. Huang, Q. Zhang, L.-L. Hua, L.-W. Zhan, J. Hou and B.-D. Li, Cell Rep. Phys. Sci., 2022, 3, 100994 CrossRef CAS.
- S. R. Mangaonkar, H. Hayashi, H. Takano, W. Kanna, S. Maeda and T. Mita, ACS Catal., 2023, 13, 2482 CrossRef CAS.
- For hydrocarboxylation by generating CO2˙−via other methods rather than using formate:
(a) H. Seo, A. Liu and T. F. Jamison, J. Am. Chem. Soc., 2017, 139, 13969 CrossRef CAS PubMed;
(b) A. Alkayal, V. Tabas, S. Montanaro, I. A. Wright, A. V. Malkov and B. R. Buckley, J. Am. Chem. Soc., 2020, 142, 1780 CrossRef CAS PubMed;
(c) H. Huang, J.-H. Ye, L. Zhu, C.-K. Ran, M. Miao, W. Wang, H. Chen, W.-J. Zhou, Y. Lan, B. Yu and D.-G. Yu, CCS Chem., 2021, 3, 1746 CrossRef CAS;
(d) G. Kang and D. Romo, ACS Catal., 2021, 11, 1309 CrossRef CAS;
(e) A. M. Sheta, A. Alkayal, M. A. Mashaly, S. B. Said, S. S. Elmorsy, A. V. Malkov and B. R. Buckley, Angew. Chem., Int. Ed., 2021, 60, 21832 CrossRef CAS PubMed;
(f) Z. G. Wu, M. Y. Wu, K. Zhu, J. Wu and Y. X. Lu, Chem, 2023, 9, 978 CrossRef CAS;
(g) T. Yuan, Z. Wu, S. Zhai, R. Wang, S. Wu, J. Cheng, M. Zheng and X. Wang, Angew. Chem., Int. Ed., 2023, 62, e202304861 CrossRef CAS PubMed.
- For other hydrocarboxylation reactions:
(a) C. Zhu, J. Takaya and N. Iwasawa, Org. Lett., 2015, 17, 1814 CrossRef CAS PubMed;
(b) K. Murata, N. Numasawa, K. Shimomaki, J. Takaya and N. Iwasawa, Chem. Commun., 2017, 53, 3098 RSC;
(c) J. Takaya, K. Miyama, C. Zhu and N. Iwasawa, Chem. Commun., 2017, 53, 3982 RSC;
(d) M. Gaydou, T. Moragas, F. Julia-Hernandez and R. Martin, J. Am. Chem. Soc., 2017, 139, 12161 CrossRef CAS PubMed;
(e) Q. Y. Meng, S. Wang, G. S. Huff and B. Konig, J. Am. Chem. Soc., 2018, 140, 3198 CrossRef CAS PubMed;
(f) W. Ren, J. Chu, F. Sun and Y. Shi, Org. Lett., 2019, 21, 5967 CrossRef CAS PubMed.
-
(a) M. He, Y. Sun and B. Han, Angew. Chem., Int. Ed., 2013, 52, 9620 CrossRef CAS PubMed;
(b) A. Alvarez, A. Bansode, A. Urakawa, A. V. Bavykina, T. A. Wezendonk, M. Makkee, J. Gascon and F. Kapteijn, Chem. Rev., 2017, 117, 9804 CrossRef CAS PubMed;
(c) M. F. Philips, G.-J. M. Gruter, M. T. M. Koper and K. J. P. Schouten, ACS Sustainable Chem. Eng., 2020, 8, 15430 CrossRef CAS;
(d) R. N. Sampaio, D. C. Grills, D. E. Polyansky, D. J. Szalda and E. Fujita, J. Am. Chem. Soc., 2020, 142, 2413 CrossRef CAS PubMed.
-
(a) O. S. Bushuyev, P. De Luna, C. T. Dinh, L. Tao, G. Saur, J. van de Lagemaat, S. O. Kelley and E. H. Sargent, Joule, 2018, 2, 825 CrossRef CAS;
(b) S.-M. Xia, Z.-W. Yang, K.-H. Chen, N. Wang and L.-N. He, Chin. J. Catal., 2022, 43, 1642 CrossRef CAS;
(c) W.-B. Huang, M. Yang and L.-N. He, J. Org. Chem., 2022, 87, 3775 CrossRef CAS PubMed;
(d) L. Wang, H. Neumann and M. Beller, Angew. Chem., Int. Ed., 2018, 57, 6910 CrossRef CAS PubMed.
-
(a) M. Whittaker, C. D. Floyd, P. Brown and A. J. H. Gearing, Chem. Rev., 1999, 99, 2735 CrossRef CAS PubMed;
(b) M. A. Carnahan and M. W. Grinstaff, Macromolecules, 2001, 34, 7648 CrossRef CAS;
(c) J. Liu, K. Dong, R. Franke, H. Neumann, R. Jackstell and M. Beller, J. Am. Chem. Soc., 2018, 140, 10282 CrossRef CAS PubMed.
-
(a) C.-K. Ran, H.-Z. Xiao, L.-L. Liao, T. Ju, W. Zhang and D.-G. Yu, National Science Open, 2022, 2, 20220024 CrossRef;
(b) Z. Yu and M. Shi, Chem. Commun., 2022, 58, 13539 RSC;
(c) X.-F. Liu, K. Zhang, L. Tao, X.-B. Lu and W.-Z. Zhang, Green Chemical Engineering, 2022, 3, 125 CrossRef;
(d) L.-L. Liao, Z. H. Wang, K. G. Cao, G. Q. Sun, W. Zhang, C. K. Ran, Y. Li, L. Chen, G. M. Cao and D.-G. Yu, J. Am. Chem. Soc., 2022, 144, 2062 CrossRef CAS PubMed. For an early example:
(e) G. F. Wright, J. Am. Chem. Soc., 1939, 61, 2106 CrossRef CAS.
-
(a) H. Senboku, H. Komatsu, Y. Fujimura and M. Tokuda, Synlett, 2001, 2001, 0418 CrossRef;
(b) H. Wang, M.-Y. Lin, H.-J. Fang, T.-T. Chen and J.-X. Lu, Chin. J. Chem., 2007, 25, 913 CrossRef CAS;
(c) G.-Q. Yuan, H.-F. Jiang, C. Lin and S.-J. Liao, Electrochim. Acta, 2008, 53, 2170 CrossRef CAS;
(d) C.-H. Li, G.-Q. Yuan, X.-C. Ji, X.-J. Wang, J.-S. Ye and H.-F. Jiang, Electrochim. Acta, 2011, 56, 1529 CrossRef CAS;
(e) Y. You, W. Kanna, H. Takano, H. Hayashi, S. Maeda and T. Mita, J. Am. Chem. Soc., 2022, 144, 3685 CrossRef CAS PubMed;
(f) W. Zhang, L. L. Liao, L. Li, Y. Liu, L. F. Dai, G. Q. Sun, C. K. Ran, J. H. Ye, Y. Lan and D.-G. Yu, Angew. Chem., Int. Ed., 2023, 62, e202301892 CrossRef CAS PubMed.
- A. Tortajada, R. Ninokata and R. Martin, J. Am. Chem. Soc., 2018, 140, 2050 CrossRef CAS PubMed.
- T. Ju, Y.-Q. Zhou, K.-G. Cao, Q. Fu, J.-H. Ye, G.-Q. Sun, X.-F. Liu, L. Chen, L.-L. Liao and D.-G. Yu, Nat. Catal., 2021, 4, 304 CrossRef CAS.
- L. Song, W. Wang, J.-P. Yue, Y.-X. Jiang, M.-K. Wei, H.-P. Zhang, S.-S. Yan, L.-L. Liao and D.-G. Yu, Nat. Catal., 2022, 5, 832 CrossRef CAS.
- H. Seo, M. H. Katcher and T. F. Jamison, Nat. Chem., 2017, 9, 453 CrossRef CAS PubMed.
- For selected difunctionalization of alkenes with CO2:
(a) V. R. Yatham, Y. Shen and R. Martin, Angew. Chem., Int. Ed., 2017, 56, 10915 CrossRef CAS PubMed;
(b) J.-H. Ye, M. Miao, H. Huang, S. S. Yan, Z. B. Yin, W. J. Zhou and D.-G. Yu, Angew. Chem., Int. Ed., 2017, 56, 15416 CrossRef CAS PubMed;
(c) J. Hou, A. Ee, H. Cao, H. W. Ong, J. H. Xu and J. Wu, Angew. Chem., Int. Ed., 2018, 57, 17220 CrossRef CAS PubMed;
(d) B. Zhang, Y. Yi, Z.-Q. Wu, C. Chen and C. Xi, Green Chem., 2020, 22, 5961 RSC;
(e) W. Zhang and S. Lin, J. Am. Chem. Soc., 2020, 142, 20661 CrossRef CAS PubMed;
(f) L.-L. Liao, G. M. Cao, Y. X. Jiang, X. H. Jin, X. L. Hu, J. J. Chruma, G. Q. Sun, Y. Y. Gui and D.-G. Yu, J. Am. Chem. Soc., 2021, 143, 2812 CrossRef CAS PubMed;
(g) W. Hang and C. Xi, Chin. J. Org. Chem., 2021, 41, 425 CrossRef CAS.
- When we were close to finish our study, Gao and Zhu's group disclosed a closely related study with 4CzIPN as the PC based on our previous study. In this work, only 3 examples of simple styrenes substrates were viable with low (about 40%) yields, and higher reaction temperature (50 °C) was required: P. Xu, S. Wang, H. Xu, Y.-Q. Liu, R.-B. Li, W.-W. Liu, X.-Y. Wang, M.-L. Zou, Y. Zhou, D. Guo and X. Zhu, ACS Catal., 2023, 13, 2149 CrossRef CAS.
- W. Xiao, X. Wang, R. Liu and J. Wu, Chin. Chem. Lett., 2021, 32, 1847 CrossRef CAS.
- M. A. Bryden and E. Zysman-Colman, Chem. Soc. Rev., 2021, 50, 7587 RSC.
- H13COOK was not commercially available.
- M. L. Czyz, M. S. Taylor, T. H. Horngren and A. Polyzos, ACS Catal., 2021, 11, 5472 CrossRef CAS.
- A. Malandain, M. Molins, A. Hauwelle, A. Talbot, O. Loreau, T. D'Anfray, S. Goutal, N. Tournier, F. Taran, F. Caillé and D. Audisio, J. Am. Chem. Soc., 2023, 145, 16760 CrossRef CAS PubMed.
- G. Filardo, S. Gambino, G. Silvestri, A. Gennaro and E. Vianello, J. Electroanal. Chem. Interfacial Electrochem., 1984, 177, 303 CrossRef CAS.
|
This journal is © The Royal Society of Chemistry 2024 |
Click here to see how this site uses Cookies. View our privacy policy here.