DOI:
10.1039/D3SC04585D
(Perspective)
Chem. Sci., 2024,
15, 7811-7823
Supramolecular interaction in the action of drug delivery systems
Received
4th October 2023
, Accepted 27th April 2024
First published on 30th April 2024
Abstract
Complex diseases and diverse clinical needs necessitate drug delivery systems (DDSs), yet the current performance of DDSs is far from ideal. Supramolecular interactions play a pivotal role in various aspects of drug delivery, encompassing biocompatibility, drug loading, stability, crossing biological barriers, targeting, and controlled release. Nevertheless, despite having some understanding of the role of supramolecular interactions in drug delivery, their incorporation is frequently overlooked in the design and development of DDSs. This perspective provides a brief analysis of the involved supramolecular interactions in the action of drug delivery, with a primary emphasis on the DDSs employed in the clinic, mainly liposomes and polymers, and recognized phenomena in research, such as the protein corona. The supramolecular interactions implicated in various aspects of drug delivery systems, including biocompatibility, drug loading, stability, spatiotemporal distribution, and controlled release, were individually analyzed and discussed. This perspective aims to trigger a comprehensive and systematic consideration of supramolecular interactions in the further development of DDSs. Supramolecular interactions embody the true essence of the interplay between the majority of DDSs and biological systems.
1. Introduction
Diseases exhibit a wide range of complexity, as evidenced by their diverse etiologies, pathogeneses, and clinical manifestations.1 This complexity is further highlighted by variations in the location of the disease.2 The current paradigm in drug development primarily centers on designing agents effectively interacting with the intended biological target. Drug therapy, when utilized as a standalone intervention, has been found to be inadequate in addressing a wide range of clinical needs and may lead to various adverse effects and complications. Approximately 40% of approved drugs exhibit limitations such as inadequate water solubility, rapid metabolism, low permeability, and insufficient elimination.3 Thus, while various routes of administration (such as oral, parenteral, inhaled, transdermal, vaginal, rectal) exist, drug delivery is required for many diseases to meet complex clinical needs.4
Drug delivery encompasses the various methods, formulations, production methods, storage systems and technologies used to transport a pharmaceutical compound to its intended site of action to produce the desired therapeutic effect.5 Since the first controlled-release formulation was approved in the 1950s,6 drug delivery systems have been widely used in various medical fields, including cardiology, ophthalmology, endocrinology, oncology, pulmonology, immunology, and pain management.7 Drug delivery systems can increase efficacy by targeting specific locations and controlling drug release, improve patient compliance by reducing dosages and administration invasiveness, and potentially lower drug doses needed.8 Drug delivery is vital for human health and has rapidly expanded, benefiting millions of patients and becoming a multi-billion dollar industry.9 However, current drug delivery strategies have not yet reached the level of specificity and efficacy envisioned by Paul Ehrlich's concept of the “magic bullet”.10 The drug delivery system faces challenges stemming from the complex and diverse improvement needs in current clinical practice.11
Different disease conditions and drug requirements result in diverse, even opposite, needs for ongoing improvement of clinical treatment methods. Some clinical needs are general such as improving therapeutic efficacy, mitigating adverse effects and decreasing the necessary drug dosage. In some cases, new administration methods need to be devised as patients are uncomfortable due to invasive and/or too frequent dosing. Certain drugs, such as nucleic acid drugs, are highly unstable in vivo, necessitating developing new methods to improve their stability. The effective treatment of many kinds of heterogeneous tumours needs personalized medicine through combination therapy.
Different clinical needs require drug delivery materials possessing all or partial capabilities of biocompatibility, efficiently and controllably loading drugs, maintaining structure and protecting drugs in vivo, crossing numerous biological barriers, tuning the spatiotemporal biological distribution, and selectively and controllably releasing drugs. Drug delivery is a multidisciplinary field that involves contributions from various fields such as material science, engineering, biology, and pharmaceutical science to develop and apply important concepts in the clinical setting. Particularly, the examination of supramolecular interactions is crucial in comprehending relationships between materials, drugs, and organisms, which would facilitate the advancement of drug delivery systems in all aspects. Supramolecular interactions are present in every stage of drug delivery systems, even if not intentionally designed, and should be considered in the design of all drug delivery systems. Clear and comprehensive summation could allow understanding the role of supramolecular interactions in the presently used drug delivery and designing new efficient and smart drug delivery systems for next-generation medical intervention.
In this perspective, we summarize the contribution of supramolecular interactions in drug delivery system, focusing on its contribution to the multiple objectives of drug delivery. The key objectives of a drug delivery system include good biocompatibility, attaining efficient and precise drug loading, robust stability prior to in vivo drug release, overcoming biological barriers, optimizing the spatiotemporal biodistribution, and controlled drug release. The supramolecular interactions involved in achieving these objectives were summarized and deliberated upon. The opportunities for future contributions and advances would be highlighted. Personal perspectives on utilizing supramolecular principles in drug carrier analysis and design will be included as appropriate. It serves to introduce drug delivery strategies on the market, ongoing clinical trials and in laboratory research. Currently, most drug delivery systems on the market or in clinical trials used the supramolecular unconsciously. Drug delivery systems via exquisite supramolecular design carried out by supramolecular chemists, to date, have not been fully evaluated clinically. Hence, the examples discussed in this perspective predominantly encompass liposomes and polymers, which widely employed in clinical applications, characterized by the involvement of supramolecular interactions. In contrast, macrocyclic delivery systems are comparatively less frequent. This review aims to call attention to the fields and act as an intellectual catalyst that takes current advances to the next step by, e.g., attracting the attention of pharmaceutical chemists, physicians and preclinical researchers. Expectedly, researchers in the fields of molecular imaging and theranostics could also learn important lessons from the advances in supramolecular interactions in drug formulations as outlined in this perspective.
2. Supramolecular interactions between carriers and biomolecules influence biocompatibility
Biocompatibility denotes the capacity of a biomaterial to elicit the appropriate cellular or tissue response from the host in a given circumstance.12 Introducing a material in body tissues can elicit various reactions, such as biomolecular corona, immune responses, inflammatory, and the subsequent repair processes, all of which are intricately intertwined with supramolecular interactions.12 Annually, hospitals in the United States experience more than 770
000 occurrences of injury or death due to adverse drug events.13 Drug delivery systems aim to minimize discomfort caused by the system or its insertion method.
Good biocompatibility of all drug delivery system is the premise of their successful application. The security aspect of drug delivery systems involves both the safety of the delivered drug and the biocompatibility of the delivery system. Drug carriers are often characterized by challenges in their degradation, metabolism, and elimination, as well as potential issues with immunogenicity, toxicity, and other undesirable effects. Materials perceived as inert, such as those utilized as protective barriers or in drug delivery systems, can still have an effect on their surroundings.
Liposomes, created through self-assembly of natural, non-toxic phospholipids and cholesterol, are the most widely employed and effective drug delivery vehicles for various chemotherapeutic agents.14 For example, Doxil, the first nanomedicine of the cytotoxic drug doxorubicin, employs liposome formulation and is marketed as a chemotherapy agent for several cancers.15 Moreover, other drugs, including mifamurtide, cytarabine, and daunorubicin–vincristine, have also been loaded into liposomes and marketed as MEPACT, DepoCyt, and Onco-TCS, respectively.16 Despite limited understanding, the successful application of liposomes is ascribed to their favourable supramolecular interactions with biological molecules in vivo, thereby showing remarkable biocompatibility (Fig. 1A). For instance, hydrophilic drugs encapsulated in liposomes can enter cells via the distinctive fusion of liposomes with the cell membrane of eukaryotic cell or bacteria17 facilitated by electrostatic interaction and hydrophobic interactions provided by fusogenic lipids. Furthermore, liposome could be recognized by relevant enzymes for complete biodegradation.18
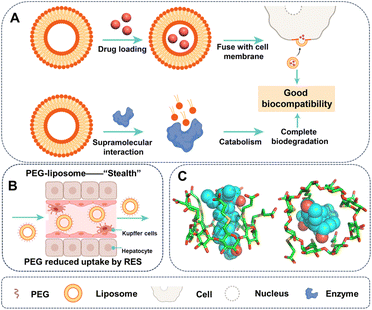 |
| Fig. 1 Schematic illustration of (A) good biocompatibility of liposome due to proper supramolecular interaction with cell membrane and enzymes. (B) PEG modification reduced the uptake by RES through minimize the supramolecular interactions. (C) Crystal structure of the host–guest complex between the gamma-cyclodextrin derivative and rocuronium (CCDC: 172 247). | |
Surface chemical modification of drug delivery systems,19 such as PEGylation, can considerably enhance their biocompatibility by altering supramolecular interactions with biomolecules.20,21 PEGylation could increase carrier solubility, shield the payload from plasma enzymes, prevent immune reactions, and reduce interaction with the reticuloendothelial system (RES) (Fig. 1B).22–24 Since 1990, over 20 FDA-approved drugs have utilized PEGylation, including treatments for diseases such as severe combined immunodeficiency, hepatitis C, multiple sclerosis, and various cancers.25 Although the mechanisms of PEGylation are not fully understood, it is conceivable that it provides physical blocking of the binding sites, and therefore decreased the supramolecular interactions, including electrostatic interactions and hydrogen bonding, which are dependent on the distance between the carrier and organism molecules. For instance, PEGylation of anti-p185HER2 antibody mitigates immune response, not by affecting its immune activity, but by diminishing the on rate in the binding kinetics.26
Supramolecular strategies provided various methods for improving the biocompatibility of drug delivery systems. One approach is to mitigate the toxicity of drugs to healthy tissues by utilizing host–guest inclusion interactions.27,28 Macrocycles, such as cyclodextrin, are prominent subjects of study in the realm of supramolecular chemistry, and they have been used in pharmaceutical industry to enhance biocompatibility by mitigating side effect of drugs. The most effective and sophisticated example is Sugammadex, a gamma-cyclodextrin derivative marketed by Merck under the brand name Bridion.29 Sugammadex complexed with rocuronium based on (1) augmented van der Waal and hydrophobic interactions resulting from extending the cavity depth of gamma-cyclodextrin and (2) improved electrostatic interaction through introducing carboxyl groups. The strong host–guest complexation (Ka ∼ 107 M−1) could reverse the effects of neuromuscular blocking agents such as rocuronium and vecuronium (Fig. 1C).30 Another approach is the construction of drug delivery systems based on supramolecular assemblies, e.g. peptide hydrogels driven by hydrophobic interaction, electrostatic interactions, hydrogen bonding and π⋯π stacking, improving the compatibility of drugs.31,32 However, synthetic or engineered drug delivery systems often face challenges of biocompatibility and immunogenicity, despite their ability to reduce drug toxicity to the carrier's level.33 Insufficient knowledge about material–tissue interactions impedes the advancement of biocompatible materials. Therefore, developing liposome-like compatible carriers with a thorough comprehension of supramolecular interactions between carriers and organisms is essential. A comprehensive understanding of supramolecular interactions between carriers and organisms is required to create carriers that are comparable to liposomes in terms of compatibility.34
3. Efficient and precise drug loading based on supramolecular interactions
Efficient drug loading is a critical aspect of developing drug delivery systems. High drug loading efficiency in drug delivery system minimizes the need for carrier materials, reducing potential side effects and manufacturing costs in drug delivery systems.35
Amphiphilic supramolecular assemblies formed by conjugates of hydrophilic and hydrophobic drugs enable self-delivery of drugs, achieving notably high drug loading, up to approximately 100% (Fig. 2A).36 A groundbreaking study produced an amphiphilic prodrug through the conjugation of the hydrophilic drug irinotecan, wherein the nitrogen of piperidine could be protonated and engage in interaction with water based on electrostatic interactions and hydrogen bonding, with the hydrophobic drug chlorambucil via a cleavable ester bond.37 The carrier-free nanomedicine approach has been applied to the development of drug conjugates such as cisplatin–vorinostat38 and erlotinib–curcumin.39 Some drugs can be assembled into nanoparticles through noncovalent interactions, such as the combination of hydrophilic clofarabine and hydrophobic raltitrexed, forming a supra-amphiphilic system that achieves ultra-high drug loading and self-delivery. Assembly of superamphiphiles also facilitates self-delivery of drugs with high loading,40 exemplified by hydrogen bond-mediated combination of hydrophilic clofarabine and hydrophobic raltitrexed.41
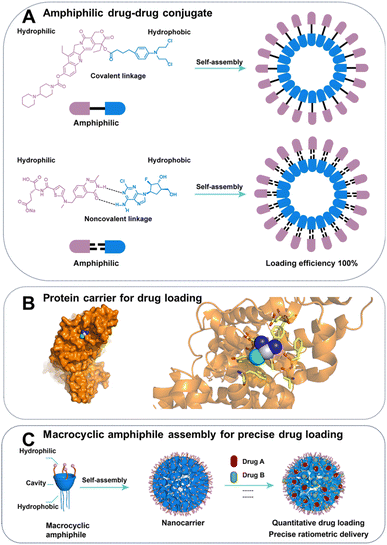 |
| Fig. 2 Schematic illustration of (A) amphiphilic drug–drug conjugate strategy. (B) Crystal structure of albumin complexing with cisplatin (PDB ID:4S1Y). (C) Schematic illustration of the advantages of assembly of macrocyclic amphiphile for precise drug loading in combination therapy. Blue area: hydrophobic cavity and lower rim of macrocycles; pink area: hydrophilic upper rim of macrocycles. | |
Drug delivery systems commonly employ porous carriers (e.g., inorganic nanoparticles,42,43 MOF,44–46 hydrogel47,48) to mix with drug solutions, with drug loading primarily dependent on supramolecular interactions such as electrostatic and hydrophobic forces.49 The low drug loading of drug delivery systems, usually only a few weight percent, were caused by not only the large molecular weight or size of the carrier, but also the absence of strong, specific interactions between the carrier and the drug. For example, although possessing high molecular weight, albumin, a protein host with specific binding sites still achieved high-efficiency loading of paclitaxel.50 Abraxane, an albumin-bound nanoparticle formulation of paclitaxel, is a highly successful example of cancer nanomedicine, approved by the FDA for treating metastatic breast cancer with drug loading of approximately 10%.51 The crystal structure of Abraxane has not been reported, but we can infer the supramolecular nature of its binding from the interaction of albumin with cisplatin (Fig. 2B).
Drug combinations that show synergy in vitro may not effectively accumulate in target tissues or cells in vivo, potentially limiting their clinical efficacy.52 Clinical combination therapies often rely on empirical derivation, optimizing individual drug doses to their maximum tolerated levels, rather than on rational identification of synergistic drug doses.53 Supramolecular assemblies have the potential to codelivery of drug combinations, in precise proportions, to the same tissue or cell, which can enhance the therapeutic effects.54–56 Following injection, Vyxeos, a liposomal formulation containing a 5
:
1 ratio of cytarabine to daunorubicin, maintains the same drug ratio in bloodstream for a duration of 24 hours, contrasting with a rapid and independent elimination from plasma observed for a saline-based cocktail of the two drugs.57,58 Currently, nanocarriers lack a means to accurately control drug ratios during loading and delivery, leading to inconsistencies between batches.59 Host–guest complexation may provide quantitative drug loading at a given concentration due to a defined number of binding sites and measurable association constants.60,61 Macrocyclic-amphiphile assemblies can load and deliver multiple drugs to tumours with precise ratios (Fig. 2C). For example, Liu et al. developed a drug carrier of assembles of an amphiphilic azocalixarene for complex with drug guests (paclitaxel and NLG919) based on electrostatic interactions provided by quaternary ammonium groups and hydrophobic interaction provided the deep hydrophobic cavity. Importantly, the ratio of the drugs loaded could be predicted by their binding constants and initial concentrations.62
Alternative drug delivery systems have the potential to offer increased capacity for drug transport. The drug delivery system NK911, composed of copolymer of PEG and polyaspartic acid, in which doxorubicin molecules were partially attached to the side chain of aspartic acid as the hydrophobic core of the micelles. Notably, the micelles can also load free doxorubicin drug component, and formed the formulation named NK911 for treatment of metastatic pancreatic cancer.63,64 NK105, a micelle-based carrier containing paclitaxel, has been evaluated for treatment of pancreatic, colonic, and gastric tumours.65–67
At ambient temperature, the majority of processes dominated by supramolecular interactions, such as hydrogen bonding, π stacking, and van der Waal interactions, are dynamic and reversible, owing to the significantly lower Gibbs energy barrier compared to chemical reactions. The phenomena would simplify material construction by leveraging spontaneity, modularity, and self-correcting abilities, thereby reducing batch-to-batch inconsistencies.68 Although numerous assemblies, including liposomes and lipid nanoparticles are in kinetic traps, their sizes can be conventionally standardized through post-drug loading extrusion processes, leveraging the dynamic and reversible characteristics of noncovalent interactions. For water-soluble siRNA, lipid nanoparticle formulations can be prepared by rapidly mixing lipid components in ethanol with an aqueous drug solution at specific pH and flow rates, triggering in situ self-assembly driven by hydrophobic interactions and drug loading through forming inverted micellar structures surrounding siRNA caused by the electrostatic interactions between cationic lipids and siRNA.69 The approach has enabled the reproducible and scalable production of lipid nanoparticle-mRNA formulations, demonstrating exceptional encapsulation efficiency and uniform size distribution.
4. Stable drug delivery systems need minimizing improper supramolecular interactions before drug release at lesion site
There are at least two factors that control the stability of drug delivery formulation. The primary consideration is to minimize the undesirable drug leakage, a crucial requirement for all drug delivery systems. The second consideration is that certain drugs are unstable in vivo and require protection by their carrier. This section provides a summary of the advancements made in enhancing the stability of drug delivery formulations through the utilization of supramolecular approaches.
Drug carriers may become unstable under the harsh conditions encountered during circulation, such as biomolecular coronas, blood flow, phagocytic cells, and excretion. The disintegration of drug carriers primarily stems from the competitive supramolecular interactions of various biological entities in bodily fluids, potentially impacting the state of molecular assemblies.70 In biological fluids, almost all nanomaterials are enveloped by a biomolecular corona caused by inevitable noncovalent associations with proteins, lipids, nucleic acids, and metabolites.71,72 Biomolecular corona, particularly protein corona, would modify physiochemical characteristics of nanoparticle surface, and therefore alter their interactions with biosystems and determine their ultimate fate (Fig. 3A).73,74 Protein corona patterns can unpredictably alter nanoparticle outcomes, such as uptake,75 biodistribution,76 immunological responses77 and toxicity,78 posing challenges for nanomedicine. Due to the dynamic nature of the supramolecular interactions, ambient factors like pH and ionic strength influence the corona profile and conformational changes of proteins binding onto the surface of nanocarriers. Additionally, weak noncovalent interactions enable the transient binding of numerous functional epitopes on the corona to multiple membrane receptors, thereby leading to subsequent biological effects, including the activation of cytosolic signal transduction.79 Notably, nanoparticles can be designed to recruit plasma proteins that are beneficial to drug delivery. For example, surface modification of liposomes with a short amyloid β-derived peptide, resulting in forming plasma apolipoprotein corona-coated liposomes that can effectively target brain tissue.80 The multifaceted roles of recognition of corona proteins in pharmacokinetics, immunoregulatory signalling, and gene expression are still not fully understood. Deciphering impact of corona protein pattern on cell internalization, tissue and organ distribution, and inflammatory cytokine secretion presents a challenge. Insufficient understanding of protein-carrier binding modes, especially for clinically used nanoparticles, hampers identification of general trends in protein corona dynamics and their relationship to paradoxical biological impacts, impeding reproducible nanomedicine development.81,82
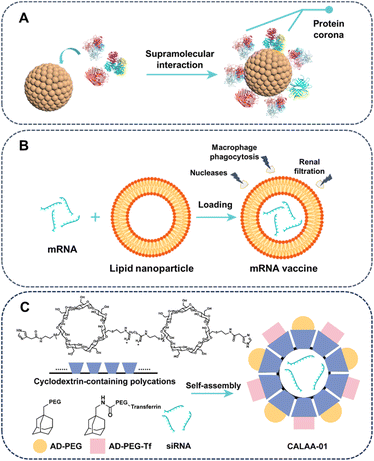 |
| Fig. 3 Schematic illustration of (A) protein corona formation through supramolecular interactions between nanoparticles and proteins, (B) protection of mRNA by nanocarriers of lipid nanoparticles in mRNA vaccine, and (C) the self-assembling nanoparticle system CALAA-01. | |
Numerous short-lived drugs exhibit inherent in vivo instability caused by various factors, with RNA, such as messenger RNA (mRNA) and small interfering RNA (siRNA), being a quintessential example. To elicit therapeutic efficacy, mRNA molecules must target specific cells precisely and produce sufficient requisite proteins. However, extracellular nucleases, macrophage phagocytosis, and renal filtration promptly degrade and remove mRNA, with a scant 0.01% of extravasated mRNAs entering target cells.83 Leveraging drug delivery advancements, various materials are devised to deliver mRNA in vivo, safeguarding it from rapid degradation by ubiquitous RNases.84,85 In the triumphant Pfizer/BioNTech BNT162b2 and Moderna mRNA-1273 COVID-19 vaccines, lipid nanoparticles protecting mRNA through encapsulating the hydrophilic mRNA by non-covalent supramolecular interactions, such as hydrogen bonding and electrostatic interactions (Fig. 3B).86,87 With the validation of vaccines against SARS-CoV-2 by Moderna and Pfizer-BioNTech, mRNA has shown immense potential for gene-editing and protein-based therapies.88,89 Moreover, siRNA also faced the stability problem similar with mRNA, and exhibited biological half-life of less than an hour in human plasma. Remarkably, CALAA01, a self-assembling nanoparticle system involving the host–guest complexation between cyclodextrin-based polycations (CDP) and two guests (adamantane-PEG (AD-PEG) and adamantane-PEG-transferrin (AD-PEG-Tf)) based on hydrophobic interactions, demonstrated remarkable efficiency in protecting siRNA in vivo (Fig. 3C).90 CALAA01 was the initial polymer-based siRNA nanomedicine to enter phase 1a/1b clinical trials in humans, although the trial was withdrawn due to dose-limiting toxicity caused by the instability of the transferrin targeting agent.91
The stability of drug carriers is profoundly affected by the interplay between their constituent materials and the surrounding environment. Carriers are cleared from the circulation mainly through interactions with reticuloendothelial system or mononuclear phagocyte system consisting phagocytes, monocytes and dendritic cells.92 Cationic nanocarriers undergo rapid clearance, whereas neutral and slightly negative nanocarriers exhibit extended half-lives in the circulation.93 In addition, adjuvants could co-assemble with nanoparticles to augment their stability.94
5. Supramolecular interactions for crossing biological barriers and regulating spatiotemporal biological distribution
Effective biodistribution and drug delivery is challenging as carriers encounter biological barriers of tissues that impede matter exchange, limiting their delivery to the target site (Fig. 4).95 A meta-analysis of 232 data sets revealed that a median of only 0.7% of the injected dose of nanoparticles successfully entered a solid tumour in a mouse model.95 Barriers can manifest as discernible physical structures, like skin, cornea, and gut, or less well-defined entities, such as the nerve's surrounding connective tissue. The extent and characteristics of barriers encountered by carriers, and strategies for surmounting them, are contingent upon tissue type, drug properties, administration route and disease type and progression. The choice of administration routes can significantly impact organ distribution, expression kinetics, and therapeutic outcomes, a determination often guided by the properties of nanoparticles and therapeutic indications. Systemic administrations are commonly utilized due to the limitations of local delivery, which is typically restricted to diseases in easily accessible sites and frequently requires invasive procedures and intricate techniques. Drug delivery systems aim to facilitate the passage of drugs across barriers that would otherwise be insurmountable at safe and reasonable doses.
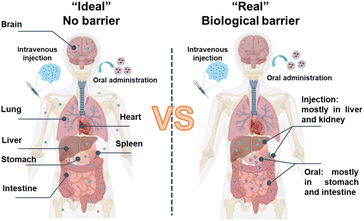 |
| Fig. 4 Schematic illustration of drug distribution of in the absence ((left), a fictitious state) and presence ((right), real situation) of biological barriers. | |
Transdermal drug administration aims to deliver drugs locally or systemically in a non-invasive manner.96 The main barrier to drug permeation is the stratum corneum, comprising dead corneocytes (primarily cross-linked keratin) and lipids (mainly cholesterol and fatty acids) forming lamellar bilayers that occupy intercellular spaces (Fig. 5A).97 The first type of chemical permeation enhancers (CPEs) is fluidizers, e.g. fatty acids, that enhance partition and diffusion coefficients by distributing into the lipid bilayers through hydrophobic interactions, facilitating the crossing of small molecules through the stratum corneum.98 Extractors, another type of CPEs, predominantly remove lipids from the stratum corneum using solvent and surfactants, boosting the diffusion coefficient by creating pores. Moreover, transdermal drug delivery has provided valuable insights for drug delivery across other similar barriers, including CPEs for amplifying nerve penetration99 and tympanic membrane permeability of drugs.100 Transportation could be facilitated through receptor binding across the blood–brain barrier.101 By altering keratin structure and disrupting lipid bilayers through supramolecular interactions with the stratum corneum,102 Hyaluronic acid enhances the permeation of various small molecular drugs.103 Low-molecular-weight chitosans improve the transdermal delivery of baicalin through dipole–dipole and van der Waals interactions with lipid bilayers.104
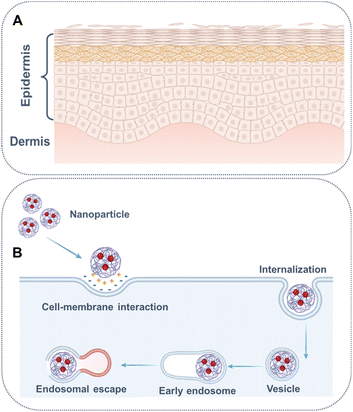 |
| Fig. 5 Schematic illustration of (A) structure of skin and (B) internalization of nanoparticle and endosomal escape. | |
Human mucus consists of thick disulfide bond cross-linked fibers of hydrophilic mucin proteins, ranging from tens to hundreds of micrometers, entangled with DNA and glycoproteins.105 Carriers employed for mucus-penetrating delivery usually possess hydrophilic properties and a neutral charge, unlike the properties needed for crossing the skin. Hydrophilic and neutrally charged PEG surface modification considerably enhanced polystyrene nanoparticle diffusion in mucus through reduced mucus interactions.106 Additionally, coating nanoparticles containing acyclovir monophosphate with PEG-rich block copolymer poloxamer 407 significantly improved mucus penetration.107 Inhaled vehicles have the potential to enhance pulmonary delivery, yet encounter barriers of mucus and pulmonary surfactant.108
Despite its popularity and acceptance, oral delivery encounters barriers in the gastrointestinal tract.109 The gastrointestinal tract exhibits significant variations in pH levels and acidity.109 Furthermore, a pronounced pH gradient across the mucosal barrier from neutral endothelial cell surfaces to acidic intestinal lumen exposes carriers to heightened vulnerability.109 Macromolecule absorption in the GI tract, like insulin and antibodies, is challenging due to tight junctions between intestinal epithelial cells. Moreover, drug influx is countered by efflux transporters on GI epithelial cell membranes, like p-glycoprotein, actively expelling drugs post-enterocyte uptake. The transferrin pathway enables transepithelial movement in the intestines using transferrin-coated nanoparticles, which is valuable for treating colon cancer and irritable bowel disease with overexpressed transferrin receptors in the intestinal mucosa.110
After contacting target cells, carriers still face multiple barriers that hinder their uptake and intracellular trafficking, impacting functional delivery.93 Anionic carriers may have difficulty contacting the cell surface due to repulsion. Cationic carriers, when excessively positively charged, have the potential to inflict damage on the cell membrane and induce cytotoxicity.111 Clathrin-mediated endocytosis, the main pathway for nanoparticle uptake, involves receptor–mediated interactions between nanoparticles and the clathrin-expressing regions of the cell membrane.112 Stiffer NPs are often better internalized as their low energy barrier caused by the less tendency to deform during membrane internalization.113
For proper functionality, every drug must reach its designated target site, such as the nucleus (doxorubicin, platinum drugs), the cytosol, or the mitochondria, at therapeutic concentrations.114 Upon endocytosis, nanoparticles are sequestered in endosomes that matured into lysosomes, greatly influencing the stability of both nanoparticles and drugs.112 To evade degradation in lysosomes, materials need escape from the endosome by responding to acidic conditions and utilizing the proton sponge effect (Fig. 5B).115 For example, within acidic endosomes, cationic polymers, such as poly(ethylene imine), acted as a proton sponge, which was partially protonation limited by the electrostatic repulsion, resulting in the bursting of the endosome and the escape of the cargos.116,117 Furthermore, Zhang et al. developed an amphiphilic self-assemble of PpIX-PEG-(KLAKLAK)2, wherein protoporphyrin IX (PpIX) was conjugated with the D-(KLAKLAK)2 peptide by a PEG linker. The D-(KLAKLAK)2 peptide moiety could target mitochondria through electrostatic interactions with negatively charged mitochondrial membrane, resulting in improved phtotodynamic therapy efficacy.118
6. Selective and controlled release of drugs through mechanism based on supramolecular interactions
Drug delivery systems often induce an immediate burst release, which not only results in the squandering of valuable drug cargo but also poses potential harm. For example, replacing polymeric microparticles with liposomes significantly reduces toxicity from the initial release of hydrophilic local anesthetics.119 Accordingly, stimuli-responsive smart drug carriers that exhibit responsiveness to various physiological stimuli such as pH, redox, enzymes, temperature, and hypoxia, have emerged as a prominent focus in drug delivery research.120 While sustained drug release patterns are commonly pursued in drug delivery systems, they may not meet the requirements of all drugs. For example, diabetics benefit from short bursts of insulin delivery.121 This section will present the concept of controlled drug release through the implementation of supramolecular design.
Diseased microenvironments often exhibit different pH with normal tissues, such as the acidic pH in tumours or the fluctuating pH during wound healing stages.122 Therefore, pH-sensitive platforms were developed to selectively release drugs under specific pH conditions. Hyperthermic wound sites can be targeted by temperature-responsive drug delivery systems.123 Nutropin Depot represents a novel controlled-release system for the systemic administration of protein, achieved by encapsulating a coordination complex of zinc ions and human growth hormone within biodegradable microspheres.124 This approach allows for sustained release of human growth hormone, facilitating the treatment of pituitary dwarfism through biweekly administration.
Corona formation can modulate drug release kinetics based on the physicochemical properties of a nanocarrier, the corona itself, and factors such as pH and temperature. However, the influence of corona on release kinetics of stimulus-responsive nanocarriers are still not well understood.125 Future research should investigate the protein corona's role on stimulus-responsive nanocarriers. Understanding the protein corona's methodological implications would enhance the design of safe and efficient therapeutic nanomedicine.126
Supramolecular approaches have been widely utilized for controlled drug release in drug delivery.127 By responding to microenvironments, such as low pH and hypoxia, the states of self-assembly and molecular recognition can be modulated, thus achieving controlled drug release.128 Remarkably, supramolecular design enables tailored release of drugs or photosensitizers at tumour sites, via mechanisms of competitive host–guest complexation rather than chemical reactions, in response to biomarker molecules such as ATP, spermine, and bile acids.129,130 For example, Zhang et al. reported a supramolecular chemotherapy system using the host–guest complex between oxaliplatin and cucurbit[7]uril driven by hydrogen bonding and hydrophobic interactions. Toxicity of the oxaliplatin was decreased by the host–guest complexation. Interestingly, cucurbit[7]uril could complex with spermine, which is overexpressed in tumor environments and essential to tumor growth, through the electrostatic interactions and hydrophobic interactions. The complexation between cucurbit[7]uril and spermine not only recovered the antitumor activity of oxaliplatin by competitive replacement, but also consumed the overexpressed spermine in tumor environments. Therefore, molecular recognitions based on supramolecular interactions will greatly enrich the types of stimuli and improve the spatiotemporal accuracy of response.
7. Conclusion and outlook
In conclusion, this perspective provides a summary of the supramolecular contributions in the action of drug delivery. It enables readers to see easily what has been accomplished to date, while giving a forward perspective into how and where the field is progressing and key challenges to be overcome in the future.
Based on our viewpoint from supramolecular chemists that continually advance the fundamental research and clinical translation of drug delivery systems, we highlighted the crucial and ubiquitous supramolecular interactions in the action of drug delivery. The importance of supramolecular principles is sometimes neglected, especially in drug delivery systems that not developed by supramolecular chemists. In this perspective, we depicted and emphasized the basic viewpoint by analysing the contribution of supramolecular interactions in the desired properties of drug delivery systems in detail. Therefore, the next personal viewpoint is that supramolecular principles should be systematically considered in developing all drug delivery systems.
The utilization of supramolecular materials, fabricated by supramolecular chemists using commonly employed building blocks,131 in the field of biomedicine is currently in its nascent stages. The majority of the research still focuses on employing macrocyclic host–guest systems for drug delivery,132 such as combination therapy based on amphiphilic azocalixarenes62 and CALAA01 based on cyclodextrins,90 which were discussed in the main text. This is likely due to the significant enhancement of weak interactions through the macrocyclic effect, making macrocycles the preferred subjects of investigation for supramolecular chemists. Interestingly, these promising preliminary studies indicate that macrocycles have demonstrated distinctive advantages in drug delivery, encompassing molecular-level protection, and quantitative drug loading, and so on. Therefore, researchers and companies in the traditional biomedical field should pay greater attention to supramolecular systems, as they offer the potential to introduce highly specific intermolecular interactions and exert precise control over molecular entities. This capability could be crucial for developing next-generation drug delivery systems. This aspect of the research is currently limited to fundamental studies and is not extensively elaborated upon in this perspective. Interested readers are encouraged to refer to our previously published reviews on the subject.133–135
The field of nanomedicine typically necessitates the integration of various functions, yet this inevitably engenders heightened intricacies in nanomedicine formulations, presenting challenges in their clinical translation. The integrated utilization of molecular recognition and assembly in supramolecular design holds promise for the simple integration of multiple functionalities through the use of basic building blocks and components. This approach enables the construction of highly reproducible and multifunctional biomaterials, thereby facilitating the treatment of complex diseases. Our work on the construction of multifunctional materials using amphiphilic calixarenes for the treatment of rheumatoid arthritis serves as a testament to this point.136
The challenge of identifying effective approaches to assess the optimal properties of nanocarriers persists. The dynamic and reversible nature of supramolecular methodologies, coupled with the modular characteristics and quantitative drug loading provided by host–guest chemistry, enables the convenient and rapid assembly of a diverse array of precisely tailored drug delivery systems. This approach may facilitate the construction of high-throughput screening systems to obtain optimal parameters such as drug loading ratios and the number of targeting moieties.137
Author contributions
All authors contributed to the writing of the manuscript.
Conflicts of interest
There are no conflicts to declare.
Acknowledgements
This work was supported by the NSFC (No. U20A20259, 22201299 and 22371147), the Fundamental Research Funds for the Central Universities (Nankai University, No. 63241407), and the NCC Fund (Grant No. NCC2020FH04). The authors would like to express their gratitude to Jie Chen and Fang-Yuan Chen from the College of Chemistry, Nankai University for their assistance in creating the figures and revising the writing style.
Notes and references
- I. de Lazaro and D. J. Mooney, Obstacles and opportunities in a forward vision for cancer nanomedicine, Nat. Mater., 2021, 20, 1469–1479 CrossRef CAS PubMed.
- Y. Jiang, Z. Jiang, M. Wang and L. Ma, Current understandings and clinical translation of nanomedicines for breast cancer therapy, Adv. Drug Delivery Rev., 2022, 180, 114034 CrossRef CAS PubMed.
- J. Hodgson, ADMET-turning chemicals into drugs, Nat. Biotechnol., 2001, 19, 722–726 CrossRef CAS PubMed.
- D. L. Stirland, J. W. Nichols, S. Miura and Y. H. Bae, Mind the gap: a survey of how cancer drug carriers are susceptible to the gap between research and practice, J. Controlled Release, 2013, 172, 1045–1064 CrossRef CAS PubMed.
- M. W. Tibbitt, J. E. Dahlman and R. Langer, Emerging Frontiers in Drug Delivery, J. Am. Chem. Soc., 2016, 138, 704–717 CrossRef CAS PubMed.
- C. Bradley, Benzedrine and dexedrine in the treatment of children's behavior disorders, Pediatrics, 1950, 5, 24–37 CrossRef CAS PubMed.
- J. Shi, Z. Xiao, N. Kamaly and O. C. Farokhzad, Self-assembled targeted nanoparticles: evolution of technologies and bench to bedside translation, Acc. Chem. Res., 2011, 44, 1123–1134 CrossRef CAS PubMed.
- J. Wang, Y. Li and G. Nie, Multifunctional biomolecule nanostructures for cancer therapy, Nat. Rev. Mater., 2021, 6, 766–783 CrossRef CAS PubMed.
- J. Shi, P. W. Kantoff, R. Wooster and O. C. Farokhzad, Cancer nanomedicine: progress, challenges and opportunities, Nat. Rev. Cancer, 2017, 17, 20–37 CrossRef CAS PubMed.
- K. Strebhardt and A. Ullrich, Paul ehrlich's magic bullet concept: 100 years of progress, Nat. Rev. Cancer, 2008, 8, 473–480 CrossRef CAS PubMed.
- A. Kakkar, G. Traverso, O. C. Farokhzad, R. Weissleder and R. Langer, Evolution of macromolecular complexity in drug delivery systems, Nat. Rev. Chem, 2017, 1, 0063 CrossRef CAS PubMed.
- D. F. Williams, On the mechanisms of biocompatibility, Biomaterials, 2008, 29, 2941–2953 CrossRef CAS PubMed.
- D. C. Classen, S. L. Pestotnik, R. S. Evans, J. F. Lloyd and J. P. Burke, Adverse Drug Events in Hospitalized Patients: Excess Length of Stay, Extra Costs, and Attributable Mortality, J. Am. Med. Assoc., 1997, 277, 301–306 CrossRef CAS PubMed.
- N. Grimaldi, F. Andrade, N. Segovia, L. Ferrer-Tasies, S. Sala, J. Veciana and N. Ventosa, Lipid-based nanovesicles for nanomedicine, Chem. Soc. Rev., 2016, 45, 6520–6545 RSC.
- Y. Barenholz, Doxil(R)-the first FDA-approved nano-drug: lessons learned, J. Controlled Release, 2012, 160, 117–134 CrossRef CAS PubMed.
- T. M. Allen and P. R. Cullis, Liposomal drug delivery systems: from concept to clinical applications, Adv. Drug Delivery Rev., 2013, 65, 36–48 CrossRef CAS PubMed.
- A. Scheeder, M. Brockhoff, E. N. Ward, G. S. K. Schierle and I. M. F. Kaminski, Molecular Mechanisms of Cationic Fusogenic Liposome Interactions with Bacterial Envelopes, J. Am. Chem. Soc., 2023, 145, 28240 CrossRef CAS PubMed.
- B. Sheikholeslami, N. W. Lam, K. Dua and M. Haghi, Exploring the impact of physicochemical properties of liposomal formulations on their in vivo fate, Life Sci., 2022, 300, 120574 CrossRef CAS PubMed.
- I. Ekladious, Y. L. Colson and M. W. Grinstaff, Polymer-drug conjugate therapeutics: advances, insights and prospects, Nat. Rev. Drug Discovery, 2019, 18, 273–294 CrossRef CAS PubMed.
- M. Goldberg, R. Langer and X. Jia, Nanostructured materials for applications in drug delivery and tissue engineering, J. Biomater. Sci., Polym. Ed., 2007, 18, 241–268 CrossRef CAS PubMed.
- J. M. Harris and R. B. Chess, Effect of pegylation on pharmaceuticals, Nat. Rev. Drug Discovery, 2003, 2, 214–221 CrossRef CAS PubMed.
- R. Gref, Y. Minamitake, M. T. Peracchia, V. Trubetskoy, V. Torchilin and R. Langer, Biodegradable long-circulating polymeric nanospheres, Science, 1994, 263, 1600–1603 CrossRef CAS PubMed.
- F. M. Veronese and G. Pasut, PEGylation, successful approach to drug delivery, Drug Discovery Today, 2005, 10, 1451–1458 CrossRef CAS PubMed.
- J. S. Suk, Q. Xu, N. Kim, J. Hanes and L. M. Ensign, PEGylation as a strategy for improving nanoparticle-based drug and gene delivery, Adv. Drug Delivery Rev., 2016, 99, 28–51 CrossRef CAS PubMed.
- D. Yadav and H. K. Dewangan, PEGYLATION: an important approach for novel drug delivery system, J. Biomater. Sci., Polym. Ed., 2021, 32, 266–280 CrossRef CAS PubMed.
- S. Kubetzko, C. A. Sarkar and A. Pluckthun, Protein PEGylation decreases observed target association rates via a dual blocking mechanism, Mol. Pharmacol., 2005, 68, 1439–1454 CrossRef CAS PubMed.
- C. L. Deng, S. L. Murkli and L. D. Isaacs, Supramolecular hosts as in vivo sequestration agents for pharmaceuticals and toxins, Chem. Soc. Rev., 2020, 49, 7516–7532 RSC.
- H. Yin, X. Zhang, J. Wei, S. Lu, D. Bardelang and R. Wang, Recent advances in supramolecular antidotes, Theranostics, 2021, 11, 1513–1526 CrossRef CAS PubMed.
- A. Bom, M. Bradley, K. Cameron, J. K. Clark, J. van Egmond, H. Feilden, E. J. MacLean, A. W. Muir, R. Palin, D. C. Rees and M.-Q. Zhang, A Novel Concept of Reversing Neuromuscular Block: Chemical Encapsulation of Rocuronium Bromide by a Cyclodextrin-Based Synthetic Host, Angew. Chem., Int. Ed., 2002, 41, 265–270 CrossRef CAS.
- J. M. Adam, D. J. Bennett, A. Bom, J. K. Clark, H. Feilden, E. J. Hutchinson, R. Palin, A. Prosser, D. C. Rees, G. M. Rosair, D. Stevenson, G. J. Tarver and M. Q. Zhang, Cyclodextrin-derived host molecules as reversal agents for the neuromuscular blocker rocuronium bromide: synthesis and structure-activity relationships, J. Med. Chem., 2002, 45, 1806–1816 CrossRef CAS PubMed.
- J. Li, R. Xing, S. Bai and X. Yan, Recent advances of self-assembling peptide-based hydrogels for biomedical applications, Soft Matter, 2019, 15, 1704–1715 RSC.
- Z. Lyu, L. Ding, A. Tintaru and L. Peng, Self-Assembling Supramolecular Dendrimers for Biomedical Applications: Lessons Learned from Poly(amidoamine) Dendrimers, Acc. Chem. Res., 2020, 53, 2936–2949 CrossRef CAS PubMed.
- D. S. Kohane and R. Langer, Biocompatibility and drug delivery systems, Chem. Sci., 2010, 1, 441–446 RSC.
- M. J. Webber, E. A. Appel, E. W. Meijer and R. Langer, Supramolecular biomaterials, Nat. Mater., 2016, 15, 13–26 CrossRef CAS PubMed.
- G. Yang, Y. Liu, H. Wang, R. Wilson, Y. Hui, L. Yu, D. Wibowo, C. Zhang, A. K. Whittaker, A. P. J. Middelberg and C. X. Zhao, Bioinspired Core-Shell Nanoparticles for Hydrophobic Drug Delivery, Angew. Chem., Int. Ed., 2019, 58, 14357–14364 CrossRef CAS PubMed.
- J. Zuo, X. Gao, J. Xiao and Y. Cheng, Carrier-free supramolecular nanomedicines assembled by small-molecule therapeutics for cancer treatment, Chin. Chem. Lett., 2023, 34, 107827 CrossRef CAS.
- P. Huang, D. Wang, Y. Su, W. Huang, Y. Zhou, D. Cui, X. Zhu and D. Yan, Combination of small molecule prodrug and nanodrug delivery: amphiphilic drug–drug conjugate for cancer therapy, J. Am. Chem. Soc., 2014, 136, 11748–11756 CrossRef CAS PubMed.
- S. Xu, X. Zhu, W. Huang, Y. Zhou and D. Yan, Supramolecular cisplatin-vorinostat nanodrug for overcoming drug resistance in cancer synergistic therapy, J. Controlled Release, 2017, 266, 36–46 CrossRef CAS PubMed.
- C. Cheng, B. Sui, M. Wang, X. Hu, S. Shi and P. Xu, Carrier-Free Nanoassembly of Curcumin-Erlotinib Conjugate for Cancer Targeted Therapy, Adv. Healthcare Mater., 2020, 9, e2001128 CrossRef PubMed.
- R. Zhang, R. Xing, T. Jiao, K. Ma, C. Chen, G. Ma and X. Yan, Carrier-Free, Chemophotodynamic Dual Nanodrugs via Self-Assembly for Synergistic Antitumor Therapy, ACS Appl. Mater. Interfaces, 2016, 8, 13262–13269 CrossRef CAS PubMed.
- D. Wang, C. Yu, L. Xu, L. Shi, G. Tong, J. Wu, H. Liu, D. Yan and X. Zhu, Nucleoside Analogue-Based Supramolecular Nanodrugs Driven by Molecular Recognition for Synergistic Cancer Therapy, J. Am. Chem. Soc., 2018, 140, 8797–8806 CrossRef CAS PubMed.
- A. C. Anselmo and S. Mitragotri, A Review of Clinical Translation of Inorganic Nanoparticles, AAPS J., 2015, 17, 1041–1054 CrossRef CAS PubMed.
- M. J. Mitchell, M. M. Billingsley, R. M. Haley, M. E. Wechsler, N. A. Peppas and R. Langer, Engineering precision nanoparticles for drug delivery, Nat. Rev. Drug Discovery, 2021, 20, 101–124 CrossRef CAS PubMed.
- R. C. Huxford, J. Della Rocca and W. Lin, Metal-organic frameworks as potential drug carriers, Curr. Opin. Chem. Biol., 2010, 14, 262–268 CrossRef CAS PubMed.
- C. Y. Sun, C. Qin, X. L. Wang and Z. M. Su, Metal-organic frameworks as potential drug delivery systems, Expert Opin. Drug Delivery, 2013, 10, 89–101 CrossRef CAS PubMed.
- Y. Wang, J. Yan, N. Wen, H. Xiong, S. Cai, Q. He, Y. Hu, D. Peng, Z. Liu and Y. Liu, Metal-organic frameworks for stimuli-responsive drug delivery, Biomaterials, 2020, 230, 119619 CrossRef CAS PubMed.
- M. Hamidi, A. Azadi and P. Rafiei, Hydrogel nanoparticles in drug delivery, Adv. Drug Delivery Rev., 2008, 60, 1638–1649 CrossRef CAS PubMed.
- N. A. Peppas, J. Z. Hilt, A. Khademhosseini and R. Langer, Hydrogels in Biology and Medicine: From Molecular Principles to Bionanotechnology, Adv. Mater., 2006, 18, 1345–1360 CrossRef CAS.
- Y. Li and L. Yang, Driving forces for drug loading in drug carriers, J. Microencapsulation, 2015, 32, 255–272 CrossRef CAS PubMed.
- E. M. Hersh, S. J. O'Day, A. Ribas, W. E. Samlowski, M. S. Gordon, D. E. Shechter, A. A. Clawson and R. Gonzalez, A phase 2 clinical trial of nab-paclitaxel in previously treated and chemotherapy-naive patients with metastatic melanoma, Cancer, 2010, 116, 155–163 CrossRef CAS PubMed.
- B. Damascelli, G. Cantù, F. Mattavelli, P. Tamplenizza, P. Bidoli, E. Leo, F. Dosio, A. M. Cerrotta, G. Di Tolla, L. F. Frigerio, F. Garbagnati, R. Lanocita, A. Marchianò, G. Patelli, C. Spreafico, V. Tichà, V. Vespro and F. Zunino, Intraarterial chemotherapy with polyoxyethylated castor oil free paclitaxel, incorporated in albumin nanoparticles (ABI-007), Cancer, 2001, 92, 2592–2602 CrossRef CAS PubMed.
- L. Ma, M. Kohli and A. Smith, Nanoparticles for combination drug therapy, ACS Nano, 2013, 7, 9518–9525 CrossRef CAS PubMed.
- Q. Hu, W. Sun, C. Wang and Z. Gu, Recent advances of cocktail chemotherapy by combination drug delivery systems, Adv. Drug Delivery Rev., 2016, 98, 19–34 CrossRef CAS PubMed.
- S. Mignani, M. Bryszewska, B. Klajnert-Maculewicz, M. Zablocka and J. P. Majoral, Advances in combination therapies based on nanoparticles for efficacious cancer treatment: an analytical report, Biomacromolecules, 2015, 16, 1–27 CrossRef CAS PubMed.
- R. X. Zhang, H. L. Wong, H. Y. Xue, J. Y. Eoh and X. Y. Wu, Nanomedicine of synergistic drug combinations for cancer therapy – Strategies and perspectives, J. Controlled Release, 2016, 240, 489–503 CrossRef CAS PubMed.
- J. Jia, F. Zhu, X. Ma, Z. Cao, Z. W. Cao, Y. Li, Y. X. Li and Y. Z. Chen, Mechanisms of drug combinations: interaction and network perspectives, Nat. Rev. Drug Discovery, 2009, 8, 111–128 CrossRef CAS PubMed.
- P. Tardi, S. Johnstone, N. Harasym, S. Xie, T. Harasym, N. Zisman, P. Harvie, D. Bermudes and L. Mayer,
In vivo maintenance of synergistic cytarabine:daunorubicin ratios greatly enhances therapeutic efficacy, Leuk. Res., 2009, 33, 129–139 CrossRef CAS PubMed.
- G. Batist, K. A. Gelmon, K. N. Chi, W. H. Miller Jr, S. K. Chia, L. D. Mayer, C. E. Swenson, A. S. Janoff and A. C. Louie, Safety, pharmacokinetics, and efficacy of CPX-1 liposome injection in patients with advanced solid tumors, Clin. Cancer Res., 2009, 15, 692–700 CrossRef CAS PubMed.
- S. Aryal, C. M. Hu and L. Zhang, Polymeric nanoparticles with precise ratiometric control over drug loading for combination therapy, Mol. Pharm., 2011, 8, 1401–1407 CrossRef CAS PubMed.
- M. J. Webber and R. Langer, Drug delivery by supramolecular design, Chem. Soc. Rev., 2017, 46, 6600–6620 RSC.
- W.-C. Geng, J. L. Sessler and D.-S. Guo, Supramolecular prodrugs based on host–guest interactions, Chem. Soc. Rev., 2020, 49, 2303–2315 RSC.
- Z. Zhang, Y. X. Yue, L. Xu, Y. Wang, W. C. Geng, J. J. Li, X. L. Kong, X. Zhao, Y. Zheng, Y. Zhao, L. Shi, D. S. Guo and Y. Liu, Macrocyclic-Amphiphile-Based Self-Assembled Nanoparticles for Ratiometric Delivery of Therapeutic Combinations to Tumors, Adv. Mater., 2021, 33, e2007719 CrossRef PubMed.
- T. Nakanishi, S. Fukushima, K. Okamoto, M. Suzuki, Y. Matsumura, M. Yokoyama, T. Okano, Y. Sakurai and K. Kataoka, Development of the polymer micelle carrier system for doxorubicin, J. Controlled Release, 2001, 74, 295–302 CrossRef CAS PubMed.
- Y. Matsumura, T. Hamaguchi, K. M. T Ura, Y. Yamada, Y. Shimada, K. Shirao, T. Okusaka, H. Ueno, M. Ikeda and N. Watanabe, Phase I clinical trial and pharmacokinetic evaluation of NK911, a micelle-encapsulated doxorubicin, Br. J. Cancer, 2004, 91, 1775–1781 CrossRef CAS PubMed.
- H. Mukai, K. Kato, T. Esaki, S. Ohsumi, Y. Hozomi, N. Matsubara, T. Hamaguchi, Y. Matsumura, R. Goda, T. Hirai and Y. Nambu, Phase I study of NK105, a nanomicellar paclitaxel formulation, administered on a weekly schedule in patients with solid tumors, Invest. New Drugs, 2016, 34, 750–759 CrossRef CAS PubMed.
- K. Kato, K. Chin, T. Yoshikawa, K. Yamaguchi, Y. Tsuji, T. Esaki, K. Sakai, M. Kimura, T. Hamaguchi, Y. Shimada, Y. Matsumura and R. Ikeda, Phase II study of NK105, a paclitaxel-incorporating micellar nanoparticle, for previously treated advanced or recurrent gastric cancer, Invest. New Drugs, 2012, 30, 1621–1627 CrossRef CAS PubMed.
- Y. Fujiwara, H. Mukai, T. Saeki, J. Ro, Y.-C. Lin, S. E. Nagai, K. S. Lee, J. Watanabe, S. Ohtani, S. B. Kim, K. Kuroi, K. Tsugawa, Y. Tokuda, H. Iwata, Y. H. Park, Y. Yang and Y. Nambu, A multi-national, randomised, open-label, parallel, phase III non-inferiority study comparing NK105 and paclitaxel in metastatic or recurrent breast cancer patients, Br. J. Cancer, 2019, 120, 475–480 CrossRef CAS PubMed.
- A. S. Braegelman and M. J. Webber, Integrating Stimuli-Responsive Properties in Host–Guest Supramolecular Drug Delivery Systems, Theranostics, 2019, 9, 3017–3040 CrossRef CAS PubMed.
- A. K. K. Leung, Y. Y. C. Tam, S. Chen, I. M. Hafez and P. R. Cullis, Microfluidic Mixing: A General Method for Encapsulating Macromolecules in Lipid Nanoparticle Systems, J. Phys. Chem. B, 2015, 119, 8698–8706 CrossRef CAS PubMed.
- J. S. Gebauer, M. Malissek, S. Simon, S. K. Knauer, M. Maskos, R. H. Stauber, W. Peukert and L. Treuel, Impact of the Nanoparticle–Protein Corona on Colloidal Stability and Protein Structure, Langmuir, 2012, 28, 9673–9679 CrossRef CAS PubMed.
- P. C. Ke, S. Lin, W. J. Parak, T. P. Davis and F. Caruso, A Decade of the Protein Corona, ACS Nano, 2017, 11, 11773–11776 CrossRef CAS PubMed.
- D. Walczyk, F. B. Bombelli, M. P. Monopoli, I. Lynch and K. A. Dawson, What the Cell “Sees” in Bionanoscience, J. Am. Chem. Soc., 2010, 132, 5761–5768 CrossRef CAS PubMed.
- M. P. Monopoli, C. Aberg, A. Salvati and K. A. Dawson, Biomolecular coronas provide the biological identity of nanosized materials, Nat. Nanotechnol., 2012, 7, 779–786 CrossRef CAS PubMed.
- M. De, C.-C. You, S. Srivastava and V. M. Rotello, Biomimetic Interactions of Proteins with Functionalized Nanoparticles: A Thermodynamic Study, J. Am. Chem. Soc., 2007, 129, 10747–10753 CrossRef CAS PubMed.
- V. Francia, K. Yang, S. Deville, C. Reker-Smit, I. Nelissen and A. Salvati, Corona Composition Can Affect the Mechanisms Cells Use to Internalize Nanoparticles, ACS Nano, 2019, 13, 11107–11121 CrossRef CAS PubMed.
- A. Salvati, A. S. Pitek, M. P. Monopoli, K. Prapainop, F. B. Bombelli, D. R. Hristov, P. M. Kelly, C. Aberg, E. Mahon and K. A. Dawson, Transferrin-functionalized nanoparticles lose their targeting capabilities when a biomolecule corona adsorbs on the surface, Nat. Nanotechnol., 2013, 8, 137–143 CrossRef CAS PubMed.
- F. Chen, G. Wang, J. I. Griffin, B. Brenneman, N. K. Banda, V. M. Holers, D. S. Backos, L. Wu, S. M. Moghimi and D. Simberg, Complement proteins bind to nanoparticle protein corona and undergo dynamic exchange in vivo, Nat. Nanotechnol., 2016, 12, 387–393 CrossRef PubMed.
- C. Ge, J. Du, L. Zhao, L. Wang, Y. Liu, D. Li, Y. Yang, R. Zhou, Y. Zhao, Z. Chai and C. Chen, Binding of blood proteins to carbon nanotubes reduces cytotoxicity, Proc. Natl. Acad. Sci. U. S. A., 2011, 108, 16968–16973 CrossRef CAS PubMed.
- K. A. Dawson and Y. Yan, Current understanding of biological identity at the nanoscale and future prospects, Nat. Nanotechnol., 2021, 16, 229–242 CrossRef CAS PubMed.
- Z. Zhang, J. Guan, Z. Jiang, Y. Yang, J. Liu, W. Hua, Y. Mao, C. Li, W. Lu, J. Qian and C. Zhan, Brain-targeted drug delivery by manipulating protein corona functions, Nat. Commun., 2019, 10, 3561 CrossRef PubMed.
- H. S. Leong, K. S. Butler, C. J. Brinker, M. Azzawi, S. Conlan, C. Dufes, A. Owen, S. Rannard, C. Scott, C. Chen, M. A. Dobrovolskaia, S. V. Kozlov, A. Prina-Mello, R. Schmid, P. Wick, F. Caputo, P. Boisseau, R. M. Crist, S. E. McNeil, B. Fadeel, L. Tran, S. F. Hansen, N. B. Hartmann, L. P. W. Clausen, L. M. Skjolding, A. Baun, M. Agerstrand, Z. Gu, D. A. Lamprou, C. Hoskins, L. Huang, W. Song, H. Cao, X. Liu, K. D. Jandt, W. Jiang, B. Y. S. Kim, K. E. Wheeler, A. J. Chetwynd, I. Lynch, S. M. Moghimi, A. Nel, T. Xia, P. S. Weiss, B. Sarmento, J. das Neves, H. A. Santos, L. Santos, S. Mitragotri, S. Little, D. Peer, M. M. Amiji, M. J. Alonso, A. Petri-Fink, S. Balog, A. Lee, B. Drasler, B. Rothen-Rutishauser, S. Wilhelm, H. Acar, R. G. Harrison, C. Mao, P. Mukherjee, R. Ramesh, L. R. McNally, S. Busatto, J. Wolfram, P. Bergese, M. Ferrari, R. H. Fang, L. Zhang, J. Zheng, C. Peng, B. Du, M. Yu, D. M. Charron, G. Zheng and C. Pastore, On the issue of transparency and reproducibility in nanomedicine, Nat. Nanotechnol., 2019, 14, 629–635 CrossRef CAS PubMed.
- E. Nance, S. H. Pun, R. Saigal and D. L. Sellers, Drug delivery to the central nervous system, Nat. Rev. Mater., 2021, 7, 314–331 CrossRef PubMed.
- Y. M. D. Lo, E. K. O. Ng and N. B. Y. Tsui, Stability of Endogenous and Added RNA in Blood Specimens, Serum, and Plasma, Clin. Chem., 2002, 48, 1647–1653 CrossRef.
- X. Hou, T. Zaks, R. Langer and Y. Dong, Lipid nanoparticles for mRNA delivery, Nat. Rev. Mater., 2021, 6, 1078–1094 CrossRef CAS PubMed.
- K. A. Hajj and K. A. Whitehead, Tools for translation: non-viral materials for therapeutic mRNA delivery, Nat. Rev. Mater., 2017, 2, 17056 CrossRef CAS.
- L. R. Baden, H. M. El Sahly, B. Essink, K. Kotloff, S. Frey, R. Novak, D. Diemert, S. A. Spector, N. Rouphael, C. B. Creech, J. McGettigan, S. Khetan, N. Segall, J. Solis, A. Brosz, C. Fierro, H. Schwartz, K. Neuzil, L. Corey, P. Gilbert, H. Janes, D. Follmann, M. Marovich, J. Mascola, L. Polakowski, J. Ledgerwood, B. S. Graham, H. Bennett, R. Pajon, C. Knightly, B. Leav, W. Deng, H. Zhou, S. Han, M. Ivarsson, J. Miller, T. Zaks and C. S. Group, Efficacy and Safety of the mRNA-1273 SARS-CoV-2 Vaccine, N. Engl. J. Med., 2021, 384, 403–416 CrossRef CAS PubMed.
- F. P. Polack, S. J. Thomas, N. Kitchin, J. Absalon, A. Gurtman, S. Lockhart, J. L. Perez, G. Perez Marc, E. D. Moreira, C. Zerbini, R. Bailey, K. A. Swanson, S. Roychoudhury, K. Koury, P. Li, W. V. Kalina, D. Cooper, R. W. Frenck Jr, L. L. Hammitt, O. Tureci, H. Nell, A. Schaefer, S. Unal, D. B. Tresnan, S. Mather, P. R. Dormitzer, U. Sahin, K. U. Jansen, W. C. Gruber and C. C. T. Group, Safety and Efficacy of the BNT162b2 mRNA Covid-19 Vaccine, N. Engl. J. Med., 2020, 383, 2603–2615 CrossRef CAS PubMed.
- U. Sahin, K. Kariko and O. Tureci, mRNA-based therapeutics-developing a new class of drugs, Nat. Rev. Drug Discovery, 2014, 13, 759–780 CrossRef CAS PubMed.
- X. Huang, N. Kong, X. Zhang, Y. Cao, R. Langer and W. Tao, The landscape of mRNA nanomedicine, Nat. Med., 2022, 28, 2273–2287 CrossRef CAS PubMed.
- K. Chaturvedi, K. Ganguly, A. R. Kulkarni, V. H. Kulkarni, M. N. Nadagouda, W. E. Rudzinski and T. M. Aminabhavi, Cyclodextrin-based siRNA delivery nanocarriers: a state-of-the-art review, Expert Opin. Drug Delivery, 2011, 8, 1455–1468 CrossRef CAS PubMed.
- C. Molinar, M. Tannous, D. Meloni, R. Cavalli and A. Scomparin, Current Status and Trends in Nucleic Acids for Cancer Therapy: A Focus on Polysaccharide-Based Nanomedicines, Macromol. Biosci., 2023, 23, e2300102 CrossRef PubMed.
- S. Schwartz, Unmet needs in developing nanoparticles for precision medicine, Nanomedicine, 2017, 12, 271–274 CrossRef CAS PubMed.
- E. Blanco, H. Shen and M. Ferrari, Principles of nanoparticle design for overcoming biological barriers to drug delivery, Nat. Biotechnol., 2015, 33, 941–951 CrossRef CAS PubMed.
- X. Cheng and R. J. Lee, The role of helper lipids in lipid nanoparticles (LNPs) designed for oligonucleotide delivery, Adv. Drug Delivery Rev., 2016, 99, 129–137 CrossRef CAS PubMed.
- S. Wilhelm, A. J. Tavares, Q. Dai, S. Ohta, J. Audet, H. F. Dvorak and W. C. W. Chan, Analysis of nanoparticle delivery to tumours, Nat. Rev. Mater., 2016, 1, 16014 CrossRef CAS.
- A. C. Watkinson, M.-C. Kearney, H. L. Quinn, A. J. Courtenay and R. F. Donnelly, Future of the transdermal drug delivery market – have we barely touched the surface?, Expert Opin. Drug Delivery, 2016, 13, 523–532 CrossRef CAS PubMed.
- M. Heisig, R. Lieckfeldt, G. Wittum, G. Mazurkevich and G. Lee, Non Steady-state Descriptions of Drug Permeation Through Stratum Corneum. I. The Biphasic Brick-and-Mortar Model, Pharm. Res., 1996, 13, 421–426 CrossRef CAS PubMed.
- M. R. Prausnitz and R. Langer, Transdermal drug delivery, Nat. Biotechnol., 2008, 26, 1261–1268 CrossRef CAS PubMed.
- E. J. Simons, E. Bellas, M. W. Lawlor and D. S. Kohane, Effect of Chemical Permeation Enhancers on Nerve Blockade, Mol. Pharmacol., 2009, 6, 265–273 CrossRef CAS PubMed.
- R. Yang, V. Sabharwal, O. S. Okonkwo, N. Shlykova, R. Tong, L. Y. Lin, W. Wang, S. Guo, J. J. Rosowski, S. I. Pelton and D. S. Kohane, Treatment of otitis media by transtympanic delivery of antibiotics, Sci. Transl. Med., 2016, 8, 356ra120 Search PubMed.
- W. M. Pardridge, The blood–brain barrier: Bottleneck in brain drug development, NeuroRx, 2005, 2, 3–14 CrossRef PubMed.
- M. Witting, A. Boreham, R. Brodwolf, K. Vávrová, U. Alexiev, W. Friess and S. Hedtrich, Interactions of Hyaluronic Acid with the Skin and Implications for the Dermal Delivery of Biomacromolecules, Mol. Pharmacol., 2015, 12, 1391–1401 CrossRef CAS PubMed.
- H. S. Jung, K. S. Kim, S. H. Yun and S. K. Hahn, Enhancing the transdermal penetration of nanoconstructs: could hyaluronic acid be the key?, Nanomedicine, 2014, 9, 743–745 CrossRef CAS PubMed.
- X. Zhou, D. Liu, H. Liu, Q. Yang, K. Yao, X. Wang, L. Wang and X. Yang, Effect of Low Molecular Weight Chitosans on Drug Permeation through Mouse Skin: 1. Transdermal Delivery of Baicalin, J. Pharm. Sci., 2010, 99, 2991–2998 CrossRef CAS PubMed.
- S. K. Lai, Y.-Y. Wang and J. Hanes, Mucus-penetrating nanoparticles for drug and gene delivery to mucosal tissues, Adv. Drug Delivery Rev., 2009, 61, 158–171 CrossRef CAS PubMed.
- S. K. Lai, D. E. O'Hanlon, S. Harrold, S. T. Man, Y.-Y. Wang, R. Cone and J. Hanes, Rapid transport of large polymeric nanoparticles in fresh undiluted human mucus, Proc. Natl. Acad. Sci. U. S. A., 2007, 104, 1482–1487 CrossRef CAS PubMed.
- L. M. Ensign, B. C. Tang, Y. Y. Wang, T. A. Tse, T. Hoen, R. Cone and J. Hanes, Mucus-penetrating nanoparticles for vaginal drug delivery protect against herpes simplex virus, Sci. Transl. Med., 2012, 4, 138ra179 Search PubMed.
- R. A. Cone, Barrier properties of mucus, Adv. Drug Delivery Rev., 2009, 61, 75–85 CrossRef CAS PubMed.
- L. M. Ensign, R. Cone and J. Hanes, Oral drug delivery with polymeric nanoparticles: the gastrointestinal mucus barriers, Adv. Drug Delivery Rev., 2012, 64, 557–570 CrossRef CAS PubMed.
- J. M. Yong, J. Mantaj, Y. A.-O. Cheng and D. A.-O. Vllasaliu, Delivery of Nanoparticles across the Intestinal Epithelium via the Transferrin Transport Pathway, Pharmaceutics, 2019, 11, 298 CrossRef CAS PubMed.
- N. Hoshyar, S. Gray, H. Han and G. Bao, The effect of nanoparticle size on in vivo pharmacokinetics and cellular interaction, Nanomedicine, 2016, 11, 673–692 CrossRef CAS PubMed.
- S. Behzadi, V. Serpooshan, W. Tao, M. A. Hamaly, M. Y. Alkawareek, E. C. Dreaden, D. Brown, A. M. Alkilany, O. C. Farokhzad and M. Mahmoudi, Cellular uptake of nanoparticles: journey inside the cell, Chem. Soc. Rev., 2017, 46, 4218–4244 RSC.
- L. Zhang, H. Chen, J. Xie, M. Becton and X. Wang, Interplay of Nanoparticle Rigidity and Its Translocation Ability through Cell Membrane, J. Phys. Chem. B, 2019, 123, 8923–8930 CrossRef CAS PubMed.
- W.-H. Chen, G.-F. Luo and X.-Z. Zhang, Recent Advances in Subcellular Targeted Cancer Therapy Based on Functional Materials, Adv. Mater., 2019, 31, 1802725 CrossRef PubMed.
- G. Chen, A. A. Abdeen, Y. Wang, P. K. Shahi, S. Robertson, R. Xie, M. Suzuki, B. R. Pattnaik, K. Saha and S. Gong, A biodegradable nanocapsule delivers a Cas9 ribonucleoprotein complex for in vivo genome editing, Nat. Nanotechnol., 2019, 14, 974–980 CrossRef CAS PubMed.
- O. Boussif, F. Lezoualc'h, M. A. Zanta, M. D. Mergny, D. Scherman, B. Demeneix and J. P. Behr, A versatile vector for gene and oligonucleotide transfer into cells in culture and in vivo: polyethylenimine, Proc. Natl. Acad. Sci. U. S. A., 1995, 92, 7297–7301 CrossRef CAS PubMed.
- T. Bus, A. Traeger and U. S. Schubert, The great escape: how cationic polyplexes overcome the endosomal barrier, J. Mater. Chem. B, 2018, 6, 6904–6918 RSC.
- K. Han, Q. Lei, S.-B. Wang, J.-J. Hu, W.-X. Qiu, J.-Y. Zhu, W.-N. Yin, X. Luo and X.-Z. Zhang, Dual-Stage-Light-Guided Tumor Inhibition by Mitochondria-Targeted Photodynamic Therapy, Adv. Funct. Mater., 2015, 25, 2961 CrossRef CAS.
- H. Epstein-Barash, I. Shichor, A. H. Kwon, S. Hall, M. W. Lawlor, R. Langer and D. S. Kohane, Prolonged duration local anesthesia with minimal toxicity, Proc. Natl. Acad. Sci. U. S. A., 2009, 106, 7125–7130 CrossRef CAS PubMed.
- C. Ding, C. Chen, X. Zeng, H. Chen and Y. Zhao, Emerging Strategies in Stimuli-Responsive Prodrug Nanosystems for Cancer Therapy, ACS Nano, 2022, 16, 13513–13553 CrossRef CAS PubMed.
- J. Kost, J. Wolfrum and R. Langer, Magnetically enhanced insulin release in diabetic rats, J. Biomed. Mater. Res., 1987, 21, 1367–1373 CrossRef CAS PubMed.
- C. R. Kruse, M. Singh, S. Targosinski, I. Sinha, J. A. Sørensen, E. Eriksson and K. Nuutila, The effect of pH on cell viability, cell migration, cell proliferation, wound closure, and wound reepithelialization: In vitro and in vivo study, Wound Repair Regen., 2017, 25, 260–269 CrossRef PubMed.
- H. Hathaway, J. Ajuebor, L. Stephens, A. Coffey, U. Potter, J. M. Sutton and A. T. Jenkins, Thermally triggered release of the bacteriophage endolysin CHAP(K) and the bacteriocin lysostaphin for the control of methicillin resistant Staphylococcus aureus (MRSA), J. Controlled Release, 2017, 245, 108–115 CrossRef CAS PubMed.
- O. L. Johnson, J. L. Cleland, H. J. Lee, M. Charnis, E. Duenas, W. Jaworowicz, D. Shepard, A. Shahzamani, A. J. S. Jones and S. D. Putney, A month–long effect from a single injection of microencapsulated human growth hormone, Nat. Med., 1996, 2, 795–799 CrossRef CAS PubMed.
- S. Sharifi, G. Caracciolo and M. Mahmoudi, Biomolecular Corona Affects Controlled Release of Drug Payloads from Nanocarriers, Trends Pharmacol. Sci., 2020, 41, 641–652 CrossRef CAS PubMed.
- M. Mahmoudi, The need for robust characterization of nanomaterials for nanomedicine applications, Nat. Commun., 2021, 12, 5246 CrossRef CAS PubMed.
- Z. Li, N. Song and Y. W. Yang, Stimuli-Responsive DrugDelivery Systems Based on Supramolecular Nanovalves, Matter, 2016, 1, 673–692 Search PubMed.
- X. Y. Hu, R. Fu and D. S. Guo, Hypoxia-Responsive Host–Guest Drug Delivery System, Acc. Chem. Res., 2023, 4, 925–938 CAS.
- Y. Chen, Z. Huang, H. Zhao, J. F. Xu, Z. Sun and X. Zhang, Supramolecular Chemotherapy: Cooperative Enhancement of Antitumor Activity by Combining Controlled Release of Oxaliplatin and Consuming of Spermine by Cucurbit[7]uril, ACS Appl. Mater. Interfaces, 2017, 9, 8602–8608 CrossRef CAS PubMed.
- J. Gao, J. Li, W.-C. Geng, F.-Y. Chen, X. Duan, Z. Zheng, D. Ding and D.-S. Guo, Biomarker Displacement Activation: A General Host–Guest Strategy for Targeted Phototheranostics in Vivo, J. Am. Chem. Soc., 2018, 140, 4945–4953 CrossRef CAS PubMed.
- Y. Wu, L. Sun, X. Chen, J. Liu, J. Ouyang, X. Zhang, Y. Guo, Y. Chen, W. Yuan, D. Wang, T. He, F. Zeng, H. Chen, S. Wu and Y. Zhao, Cucurbit[8]uril-based water-dispersible assemblies with enhanced optoacoustic performance for multispectral optoacoustic imaging, Nat. Commun., 2023, 14, 3918 CrossRef CAS PubMed.
- J. Zhou, L. Rao, G. Yu, T. R. Cook, X. Chen and F. Huang, Supramolecular cancer nanotheranostics, Chem. Soc. Rev., 2021, 50, 2839–2891 RSC.
- W.-C. Geng, J. L. Sessler and D.-S. Guo, Supramolecular prodrugs based on host–guest interactions, Chem. Soc. Rev., 2020, 49, 2303–2315 RSC.
- Z. Zheng, W.-C. Geng, Z. Xu and D.-S. Guo, Macrocyclic Amphiphiles for Drug Delivery, Isr. J. Chem., 2019, 59, 913–927 CrossRef CAS.
- X.-Y. Hu, R. Fu and D.-S. Guo, Hypoxia-Responsive Host–Guest Drug Delivery System, Acc. Mater. Res., 2023, 4, 925–938 CrossRef CAS.
- S. Li, J.-J. Li, Y.-Y. Zhao, M.-M. Chen, S.-S. Su, S.-Y. Yao, Z.-H. Wang, X.-Y. Hu, W.-C. Geng, W. Wang, K.-R. Wang and D.-S. Guo, Supramolecular Integration of Multifunctional Nanomaterial by Mannose-Decorated Azocalixarene with Ginsenoside Rb1 for Synergistic Therapy of Rheumatoid Arthritis, ACS Nano, 2023, 17, 25468–25482 CrossRef CAS PubMed.
- D. G. Anderson, S. Levenberg and R. Langer, Nanoliter-scale synthesis of arrayed biomaterials and application to human embryonic stem cells, Nat. Biotechnol., 2004, 22, 863–866 CrossRef CAS PubMed.
|
This journal is © The Royal Society of Chemistry 2024 |
Click here to see how this site uses Cookies. View our privacy policy here.