DOI:
10.1039/D3SC05357A
(Edge Article)
Chem. Sci., 2024,
15, 5980-5992
Electrosynthesis of iminophosphoranes and applications in nickel catalysis†
Received
10th October 2023
, Accepted 6th March 2024
First published on 8th March 2024
Abstract
P(V) iminophosphorane compounds are accessed via electrochemical oxidation of commercially available P(III) phosphines, including mono-, di- and tri-dentate phosphines, as well as chiral phosphines. The reaction uses inexpensive bis(trimethylsilyl)carbodiimide as an efficient and safe aminating reagent. DFT calculations, cyclic voltammetry, and NMR studies provide insight into the reaction mechanism. The proposed mechanism reveals a special case of sequential paired electrolysis. DFT calculations of the frontier orbitals of an iminophosphorane are compared with those of the analogous phosphines and phosphine oxides. X-ray crystallographic studies of the ligands as well as a Ni-coordination complex provide structural insight for these ligands. The utility of these iminophosphoranes as ligands is demonstrated in nickel-catalyzed cross-electrophile couplings including C(sp2)–C(sp3) and C(sp2)–C(sp2) couplings, an electrochemically driven C–N cross-coupling, and a photochemical arylative C(sp3)–H functionalization. In some cases, these new ligands provide improved performance over commonly used sp2-N-based ligands (e.g. 4,4′-di-tert-butyl-2,2′-bipyridine).
Introduction
Phosphorus-containing compounds are ubiquitous in catalysis,1 particularly as phosphorus(III)-based ligands in transition metal catalysis.2,3 However phosphorus(V) compounds are relatively underexplored as ligands or catalysts. Phosphine oxide (P
O) ligands have been featured in Pd-couplings4–7 and asymmetric Cu-catalysed alkylation8 of imines and nitroalkenes (see ESI Fig. S1† for additional details). P(V)-containing structures have also been used as organocatalysts, including the use of phosphine oxide-based catalysts for enantioselective aldol reactions.9 P
S and P
Se based phosphorimide catalysts have been used to mediate per-silylation of 2′-deoxynucleosides to access the corresponding glycals.10 P
N based structures such as iminophosphoranes are known but represent an underutilized class of ligands in earth-abundant metal catalysis.11 There are exciting results of utilizing iminophosphoranes as ligands in catalytic cross-coupling reactions (e.g., Sonogashira,12 Suzuki-Miyaura,13 Kumada,14 and Negishi couplings14b) and hydrogenations of alkene,15 and ketones,16 cyclopropanation,17 alkene oligo- and polymerizations,18 ring opening polymerizations (e.g. lactide, ε-caprolactone),19 as well as in organocatalysis,20 amongst others.11Scheme 1 illustrates a selection of these examples. Iminophosphoranes have also been used as organic neutral super-electron donors in organic synthesis.21
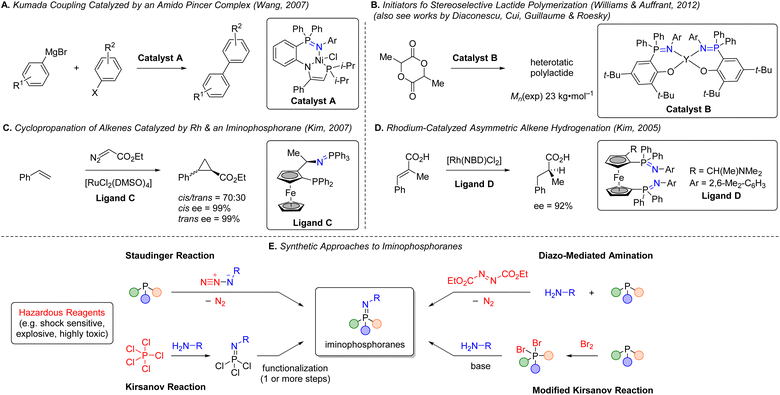 |
| Scheme 1 (A–D) Applications of iminophosphoranes as ligands in catalysis. (E) Synthetic approaches to iminophosphoranes. | |
Despite their appeal in catalysis, access to iminophos-phoranes remains limited, in stark contrast to the hundreds of commercially available P(III) ligands. The relative scarcity of applications of iminophosphoranes can be attributed to difficulty in their synthesis (e.g. hazardous reagents), limited access to commercially available precursors, and limited reaction scope. Scheme 1E illustrates a variety of commonly used synthetic approaches to iminophosphoranes. For example, synthesis through Staudinger reaction22 involves the use of azides which are potentially explosive, and requires one or more steps to access the requisite azide. Other approaches to iminophosphoranes include the Kirsanov reaction23 starting from hazardous PCl5, and the modified Kirsanov reaction,11,24 which relies on a two-step bromination–amination process of a phosphine. Other approaches exist with limited scope, such aminations facilitated by diethylazodicarboxylate,25 which is also a hazardous and shock-sensitive reagent.26 More recent approaches to iminophosphoranes have included the photolytic generation of nitrenes from bespoke dibenzothiophene N-substituted sulfilimine or dioxazolones reagents.27 More recently, the use of N-pivaloyloxybenzamide and iron catalysis was reported access N-acyl iminophosphoranes.28 Morandi reported a related approach using hydroxylamine-derived triflic acid salt to access iminophosphorane triflic acid salts.29 Ultimately, safe and scalable methods with broad scope are in high demand. To address these limitations, we wondered whether electrochemistry30 could be used to convert commercially available P(III) ligands to P(V) iminophosphorane sp2-N-ligands (Scheme 2A) via an environmentally sustainable and operationally simple and safe process. The potential to access novel iminophosphorane-containing P
N ligands from readily available phosphines would quickly expand the diversity of bi- and tridentate ligands in analogy to the classic sp2-nitrogen-based bipyridine and terpyridine ligands (Scheme 2B), which are gaining popularity in base-metal catalysis. Herein, we report an electrochemical method to access iminophosphoranes on gram scale (in batch and flow), mechanistic studies of this new transformation, and applications of these new iminophosphoranes as ligands in nickel catalysis under thermal, electrochemical, and photochemical conditions.
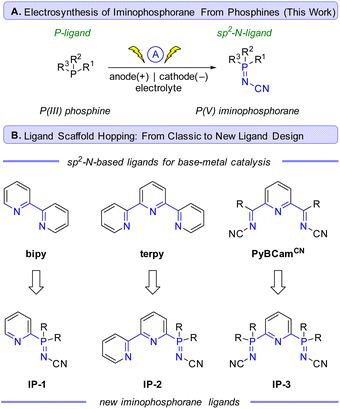 |
| Scheme 2 (A) Electrosynthesis of iminophosphoranes from phosphines. (B) Ligand scaffold hopping. | |
Results and discussion
Reaction optimization
Inspired by the success of the N-cyano imine ligands (i.e. pyridine-2,6-bis(N-cyanocarboxamidine), PyBCamCN) reported by Weix and Hansen for nickel-catalyzed cross-electrophile couplings (Scheme 2B),31 we chose to focus on N-cyano iminophosphoranes (Scheme 2A) for our initial explorations. Specifically, we targeted iminophosphorane 1a (Scheme 3) for the reaction development as it is air-stable and has a UV-vis chromophore, thus enabling analysis of reaction mixture via UPLC.
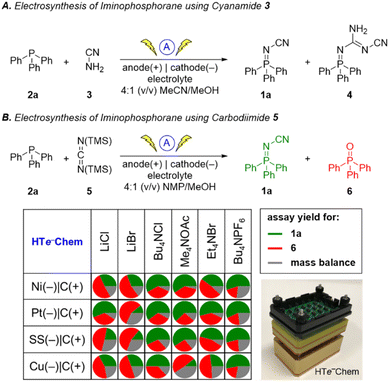 |
| Scheme 3 (A) Initial attempts at synthesizing iminophosphorane 1a using cyanamide. (B) Heat map of assay yields obtained using HTe−Chem to screen electrolytes and electrode for the synthesis of iminophosphorane 1a using bis(trimethylsilyl)carbodiimide. Conditions: 30 μmol scale (0.1 M) using constant current (1 mA) using 2 F mol−1 charge at room temperature (Note: C = graphite, SS = stainless steel). | |
Given that the modified Kirsanov reaction uses Br2 to oxidize PPh3 to Ph3PBr2 followed by amination to access iminophosphoranes,11 we explored using bromide-containing electrolytes to effect an anodic generation of Br2 to mediate the oxidation of Ph3P (2a) in the presence of cyanamide (3) (Scheme 3A). The use of Et4NBr as an electrolyte enabled oxidation of PPh3 in 4
:
1 (v/v) MeCN/MeOH provided 15% yield of 1a when using a graphite anode, a stainless-steel cathode along with guanidine 4 in 12% yield. The structure of 4 was confirmed by X-ray crystallography (Fig. 1).
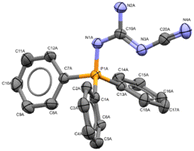 |
| Fig. 1 X-ray crystallographic structure of side-product 4 (CCDC 2259467). ORTEP ellipsoid plotted at 50% probability. | |
We explored an alternative aminating reagent towards improving the selectivity for iminophosphorane 1a over guanidine 4. We wondered whether bis(trimethylsilyl)carbodiimide (5) could provide a more soluble surrogate of cyanamide towards improving the outcome of the reaction. Indeed, the use of 5 under otherwise identical conditions provided iminophosphorane 1a in 70% yield and no detectable guanidine 4. We explored these conditions with a handful of other phosphines but soon realized that the solubility of the phosphine starting material was poor in a number of examples (e.g. xanthphos).
Thus, we replaced MeCN with NMP as a cosolvent to achieve improved solubility, however the yield for 1a dropped to 43%. Given the multivariate nature of this optimization problem, we turned to high-throughput experimentation to address this challenge. Using the recently reported32 and commercialized33 HTe−Chem reactor, we explored both electrolyte and electrode variables using 4
:
1 (v/v) NMP/MeOH as the solvent system (Scheme 3B). The use of Me4NOAc as the electrolyte in combination with platinum cathode and graphite anode provided the highest yield of iminophosphorane 1a. The Ph3P
O (6) side product was postulated to arise from adventitious water or oxygen in the reaction mixture reacting competitively against the aminating reagent.34
Based on reaction optimization using the HTe−Chem reactor, the optimal conditions were translated from 30 μmol scale to the Electrasyn 2.0 setup on 2 mmol scale using a current of 10 mA (j = 4.2 cm2 mA−1) and a charge of 2.5 F mol−1. The choice of NMP and Me4NOAc also facilitated reaction workup, as the reaction could be poured into water to precipitate out the desired product in many cases while leaving the electrolyte in solution. Collection of the crude product via filtration followed by recrystallization or column chromatography afforded the desired products in high purity with a variety of substrates (see ESI† for details).
Substrate scope
With optimal reaction conditions in hand, we turned our attention to exploring the substrate scope of our electrochemical synthesis of iminophosphoranes (Table 1). We targeted a diverse substrate scope, drawing inspiration from commercially available phosphine ligands commonly used in transition-metal catalysis (e.g., cross-couplings). Amination of triphenylphosphine on 2 mmol scale provide 1a in 83% yield. Electronically varied triarylphosphines are well tolerated as demonstrated with para-substituted analogues of triphenylphosphine: p-OMe (7) 74% yield, p-F (8) 92% yield, p-Cl (9) 73% yield, and p-CF3 (10) 83% yield. Styrenyl containing product 11 is obtained in 79% yield, which could have application as a precursor to iminophosphorane-functionalized polystyrene materials (e.g. polystyrene resin-bound ligand/catalyst for heterogenous catalysis).35Ortho-substitution is well tolerated as demonstrated by being able to access product 12 in 86% yield. Even tertiary amines functionalized phosphine can be carried through the reaction, albeit in diminished yield, as exemplified with an analogue of Ph2-APhos to access 13 in 39% yield.
Table 1 Reaction scope for the electrosynthesis of iminophosphorane using an IKA ElectraSyn 2.0a
Standard condition: 2.0 mmol of phosphine (0.13 M) in 4 : 1 v/v NMP/MeOH containing 5 (6.0 mmol of per phosphorus), and 1.5 mmol of Me4NOAc; constant current electrolysis (10 mA, j = 4.2 mA cm−2) and a charge of 2.5 F mol−1 per phosphorus using a graphite anode and a platinum foil cathode.
1 mmol of phosphine (0.07 M) using 14 : 1 v/v NMP/MeOH containing 1.5 mmol of Me4NOAc.
Same as standard conditions, except 6.0 mmol of 5 was used and the charge was 2.5 F mol−1.
Same as standard conditions, except 6.0 mmol of N-cyanoguanidine was used instead of 5.
|
|
Arylalkylphosphines are also suitable substrates, as iminophosphorane 14 is accessed in 56% yield from diphenylcyclohexylphosphine. Conversion of dicyclohexylphenylphosphine to 15 is achieved in high yield (82%). The conversion of CyJohnPhos to 16 in 75% yield demonstrates that an ortho-aryl substituent on the phenyl ring is tolerated with these dialkylmonoarylphoshine substrates. Acetals can be carried through this transformation as showcased with the functionalization of the meCgPPh ligand to product 17 in 89% yield. Trialkylphosphines are also suitable as demonstrated by the use of tribenzylphosphine to access 18, although a diminished yield was observed.
Heterocycles can also be brought through this transformation to access iminophosphorane products, potentially providing bidentate functionality, as demonstrated with products (19–23). The incorporation of pyridyl fragments is exemplified with both triaryl and diarylphosphines with products 19 (86% yield), 20 (87% yield), 21 (83% yield), 22 (65%), and 23 (85% yield), while access to a pyrazole-containing ligand is demonstrated with product 24 (81% yield). Chiral phosphine ligands, such as those based on phosphinooxazolines (PHOX) substructures, can be transformed to their corresponding iminophosphoranes. This was exemplified with products 25–27 that were obtained in yields ranging from 55–70%, without evidence of ee erosion.
The bidendate phosphines DPPE, DPEPhos, and Xantphos provide 28–30 in 87%, 63%, and 79% yield, respectively. 2,6-Bis(diphenylphosphino)pyridine is used to make 31 in 68% yield, providing a tridentate ligand36 based on three sp2-nitrogens. The privileged chiral ligand37 BINAP is converted to its iminophosphorane analog 32 in 62% yield. Similarly, popular BIPHEP and SEGPHOS ligand scaffolds are used to access 33 and 34 in 68% and 43% yields, respectively. The bis-amide containing Trost ligand38 (S,S)-DACH could be converted to the corresponding bis-iminophosphorane 35 in 76% yield.
Tripodal ligands are useful in coordination chemistry and catalysis. We explored the use of commercially available tridentate “triphos”39 ligands which enabled access to tris(iminophosphorane) ligands 36 and 37 in 44% and 74% yield, respectively. These ligands are envisioned to be useful for tripodal complex formation, a strategy that is often used with first-row transition metals.40
Access to hetero-bis-phosphine derivatives was explored as illustrated with products 38–40. Two strategies were utilized depending on the target: (1) starting from bis-phosphine mono-oxides, or (2) passing slightly more charge (i.e. 2.5 F mol−1) than the theoretical minimum charge of 2 F mol−1 to oxidize one of the two phosphines. Mixed P
O,P
N ligand 38 was accessed starting from the commercially available DPPE-mono-oxide (i.e. P(III)–P(V) monoxide). In contrast, mixed P(III)–P(V) DPPE structures 39 and 40 were obtained by passing only 2.5 F mol−1 of charge under otherwise identical conditions to those employed in Table 1. Selectivity between mono- and bis-oxidation products is challenging and is the cause of the lower yields for substrates 39 and 40. For example, bis oxidation to 28 is observed in ca. 40% yield after 2.5 F mol−1 when synthesizing 39. The ESI† contains additional substrates that were attempted but provide low yield or no product. Limitations include the use of electron-rich phosphines like trialkylphosphites, triphenylphosphites, although trialkylphosphines are suitable (see the ESI† for details).
Finally, returning to the side-product 4 that was obtained during the reaction optimization work, we wondered whether inexpensive N-cyanoguanidine, commonly used as a fertilizer,41 could be utilized in our reaction as the aminating reagent. Gratifyingly, the formation of iminophosphorane 4 containing cyanoguanidine was achieved in 49% yield.
The iminophosphoranes in Table 1 were isolated by either precipitation from the crude reaction mixture using water as an anti-solvent or via an aqueous workup, and in numerous cases a subsequent purification step via silica gel column chromatography, all in the presence of air. This highlights their air stability and resistance to hydrolysis. This is in contrast to N-alkyl substituted iminophosphoranes, which can be susceptible to decomposition to the corresponding phosphine oxide (e.g. Staudinger ligation).22
Reaction mechanism
In order to gain mechanistic insight into the reaction, we conducted DFT calculations and followed up with a series of experiments based on NMR spectroscopy (1H, 13C, 31P) and cyclic voltammetry. Five potential mechanisms were initially considered (See ESI† Scheme S2 along with associated discussion). Based on the data from these studies, a proposed mechanism is illustrated in Scheme 4. Reduction of methanol at the cathode generates hydrogen and methoxide. The methoxide attacks diimide 5, which upon loss of TMS–OMe (41) generates anion 42. Oxidization of 42 leads to N-centered radical 43. Subsequent attack of phosphine (2) generates 44 (ref. 42) which is immediately oxidized to 45. Generation of a second equivalent of methoxide, analogous to above, enables breakdown of 45 to generate the desired iminophosphorane product 46 and a second equivalent of TMS–OMe (41). To the best of our knowledge this represents a rare example of a domino electrolysis reaction,43,44 which could be categorized as a sequential paired electrolysis followed by a convergent paired electrolysis.30a,45
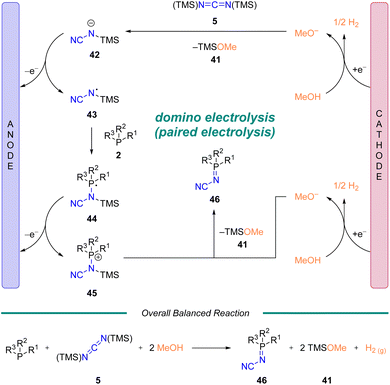 |
| Scheme 4 Proposed mechanism of domino-electrolysis. | |
Ligand properties
To gain insight into electronic effects of modifying P(III) ligands into their analogous P(V) structures (various iminophosphoranes and phosphine oxide), we computed their frontier molecular orbitals using M06-2X/6-31+G(d) SMD = MeCN. Fig. 2 illustrates the HOMO and LUMO orbitals for Ph3P (2a), Ph3P
N–CN (1a), Ph3P
N–Ph (47), Ph3P
N–Me (48), Ph3P
N–H (49), and Ph3P
O (6). The HOMO energy decreases monotonically across the following series: −7.05 eV (Ph3P
N-Ph), −7.31 eV (Ph3P), −7.34 eV (Ph3P
N–Me), −7.73 eV (Ph3P
N–H), −8.13 eV (Ph3P
N–CN), −8.48 eV (Ph3P
O); all values are versus vacuum. Across the series of iminophosphoranes in Fig. 2, N-cyano iminophosphorane 1a has the lowest lying HOMO and thus should be the most oxidatively resistant from the series and is in consistent the observation that it is air-stable. The DFT calculations show the HOMO is destabilized in the N-cyano iminophosphoranes compared to the analogous phosphine oxide, suggesting stronger σ-donor ability, yet still weaker than the analogous P(III) ligand. The LUMO energies do not decrease monotonically when considering the ligands in the same order as above, specifically the LUMO energy varies as follows: −0.01 eV (Ph3P), −0.34 eV (Ph3P
N–Ph), −0.01 eV (Ph3P), −0.31 eV (Ph3P
N–Me), −0.31 eV (Ph3P
N–H), −0.58 eV (Ph3P
N–CN), and −0.15 eV (Ph3P
O). Overall, these changes highlight that formal oxidation of the P(III) ligands to P(V) results not only in significant electronic changes, but also that these changes may be different depending on which group is attached to the P(V) centre (O for a phosphine oxide or various N–R groups for the various iminophosphoranes). Experimental study of the reduction potential across a subset of the iminophosphoranes using cyclic voltammetry reveals that these ligands are redox active (Fig. 3). Reversible reduction is observed for N-cyano iminophosphoranes, such as pyridyl containing ligand 19 (Ered = −2.45 V vs. Fc/Fc+) and bipyridyl based ligands 21 (Ered = −2.34 V vs. Fc/Fc+), but not triphenylphosphine based N-phenyl iminophosphorane 47 (Ep1/2,red = −2.97 V vs. Fc/Fc+), nor N-cyano iminophosphorane 1a (Ep1/2,red = −2.79 V vs. Fc/Fc+). Bis-iminophosphorane functionalized pyridine ligands 31 exhibits two sequential reduction events: (Ep1/2,red = −2.06 V and −2.22 V vs. Fc/Fc+), each is quasi-reversible. The redox active nature of these ligands may be beneficial for catalytic applications where reduction events are taking place (e.g. metal catalysed reductive cross-couplings).
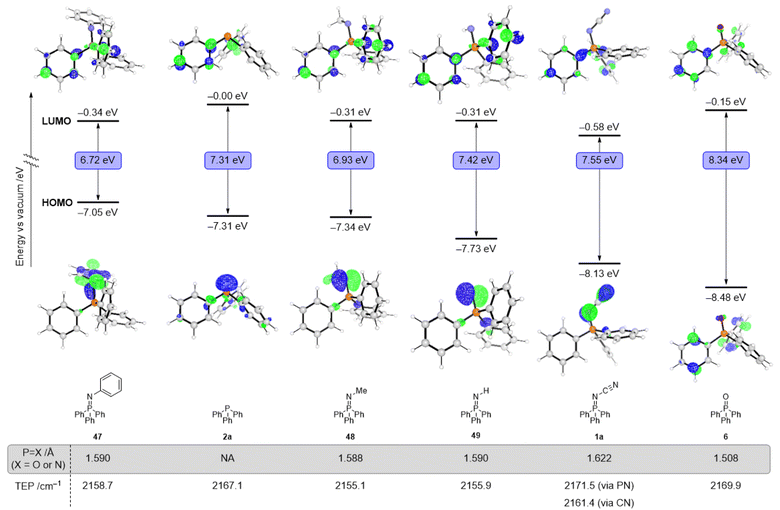 |
| Fig. 2 DFT computed lowest unoccupied molecular orbital (LUMO), (top) and highest occupied molecular orbital (HOMO) (bottom) for various P(III) and P(V) ligands using M06-2X/6-31+G(d) SMD = MeCN (note: orbitals are plotted using isolvalue = 0.075 a.u.) along with P = X (X = O or N) bond lengths. Tolman electronic parameters obtained from DFT calculations using MPW1PW91 functional and the following basis sets: 6-311+G(2d) for Ni and 6-311+G(d,p) for all other atoms. | |
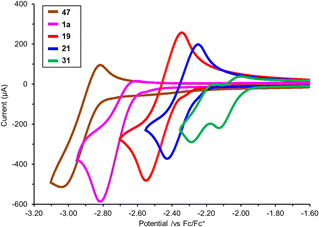 |
| Fig. 3 Cyclic voltammetry of selected iminophosphoranes illustrating their redox active properties. Data measured from 10 mM solutions of analyte in 0.1 M Bu4NPF6 in MeCN using a glassy carbon working electrode, a Pt counter electrode, a reference electrode comprised of a Ag wire in 10 mM AgNO3 in 0.1 M Bu4NPF6 in MeCN (ν = 500 mV s−1). | |
We also calculated the Tolman electronic parameter (TEP) to characterize the electronic properties of these ligands (Fig. 2).46–48 The TEP for a ligand L, which is defined as the IR frequency associated with the A1 stretch of the CO groups of the Ni(CO)3L complex, correlates with the electron-donating ability of the ligand. The computed TEP value of Ph3P
N–CN (1a) for binding via the nitrogen of the P
N moiety is higher (2171.5 cm−1) than the TEP values of Ph3P
N–Ph (2158.7 cm−1) and Ph3P
N–Me (2155.1 cm−1), supporting our proposal that N-cyano iminophosphoranes are more electron-deficient than N-aryl or N-alkyl iminophosphoranes.
To probe structural aspects of these new iminophosphoranes, a number of ligands (13, 24, (R)-25, 28, 29, (S)-33, 38, and 39), were crystallized and characterized by X-ray crystallography (Fig. 4).49 Key structural parameters are summarized in the ESI† (see Table S11). The bent geometry of the “P
N–CN” fragment was confirmed with a typical bond angle associated with the P
N–C fragment being ca. 124°. The iminophosphorane 4, (R)-25, 28, 29, 38 and 39 have comparable P
N bond lengths to Ph3P
N–Ph (1.603 Å), see ESI.†
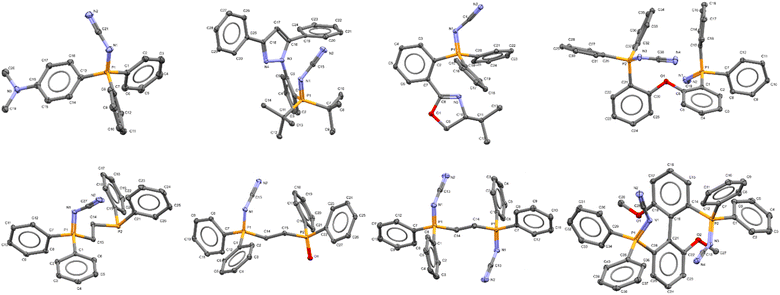 |
| Fig. 4 X-ray crystallographic structure of iminophosphorane, (top row, left to right): 13 (CCDC 2258974), 24 (CCDC 2258975), (R)-25 (CCDC 2259466), 29 (CCDC 2258980); (bottom row, left to right): 39 (CCDC 2258979), 38 (one of two inequivalent geometries, CCDC 2258978), 28 (CCDC 2258976), and (S)-33 (CCDC 2258977) (Note ORTEP ellipsoid plotted at 50% probability). | |
A more detailed comparison of bond metrics is provided in the ESI,† including comparison with X-ray crystallographic structures in the Cambridge Structural Database. The crystal structure of iminophosphorane (R)-25 and (S)-33 confirmed that the absolute configuration of the chiral starting materials was retained in our electrochemical synthesis.
To learn about the coordination geometry N-cyano iminophosphoranes, we grew single crystals from ca. 3
:
1 (v/v) MeCN/MeOH solutions of ligand 21 in the presence of NiBr2·3H2O using Et2O vapour diffusion. The X-ray crystallographic structure obtained was nickel complex Ni-1 (Fig. 5) which is a tetranuclear complex, where each Ni has octahedral coordination geometry (Fig. 5B). Both sp2-N of the 2,2′-bipyridine moiety and the nitrogen of the P
N fragment of the iminophosphorane coordinate to Ni. The nitrile nitrogen of the iminophosphorane coordinates to a neighboring Ni atom in a cyclic tetrameric arrangement. Additional coordination sites on the Ni are occupied by bromide and methanol (where the occupancy is split 75%/25% between these two ligands as shown in Fig. 5).
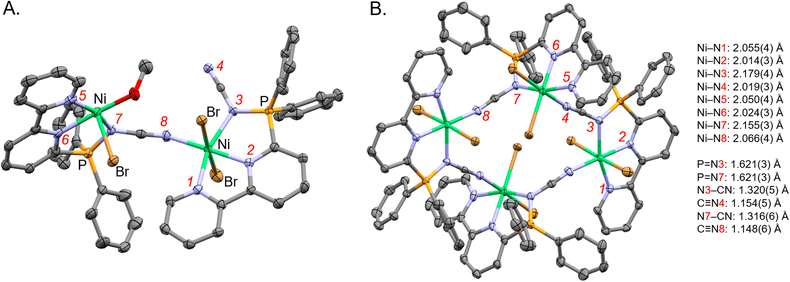 |
| Fig. 5 X-ray crystallographic structure of nickel complex Ni-1 (CCDC-2329725). (A) A subunit of Ni-1 illustrating that both Ni bound to both sp2-N of bipyridine and the N of the P N unit of ligand 21 (minor part with methanol coordinating to the nickel center shown). (B) The tetranuclear arrangement of Ni-1 (minor methanol motif omitted and major bromine part shown). Hydrogen atoms, non-coordinative counter ions, solvent, and minor parts (by occupancy) omitted for clarity. Thermal ellipsoids given at the 50% probability level. Color code: grey (carbon), red (oxygen), blue (nitrogen), green (nickel), orange (phosphorus), brown (bromine). | |
The fact that either nitrogen of the N-cyano iminophsphorane can participate in coordination to metals is consistent with previous coordination complexes of Ph3P
N–CN with Pt and Pd.50 Coordination of Ni to N-aryl iminophosphorane has also been documented.51 The ambidentate nature of N-cyano iminophsphoranes is characterized by the computed TEP values shown in Fig. 2 which highlight the nitrogen of the C
N moiety is more electron donating (TEP = 2161.4 cm−1) than the nitrogen of the P
N moiety (2171.5 cm−1).
Application to catalysis
To demonstrate the utility of the iminophosphorane ligands, we chose to explore five types of Ni-catalysed cross-coupling reactions which are reshaping how chemists synthesize molecules in industry (Scheme 5 and 6), specifically, both a thermally and an electrochemically driven C(sp2)–C(sp3) cross-electrophile coupling (XEC), a thermally activated C(sp2)–C(sp2) XEC, a photochemical arylative C–H functionalization, and a site-selective C–N coupling using electrochemistry.
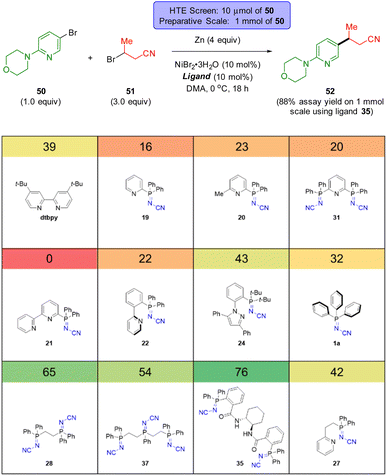 |
| Scheme 5 HTE screen (10 μmol scale based on 50) of sp2-N-based ligands in a thermal C(sp2)–C(sp3) XEC coupling. The number in the coloured box above each ligand is the assay yield for 52. | |
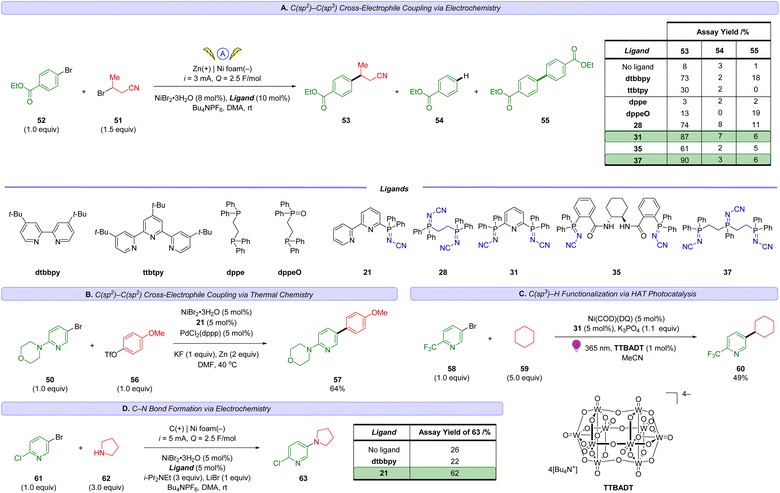 |
| Scheme 6 Application of iminophosphoranes in a nickel-catalyzed reactions: (A) C(sp2)–C(sp3) cross-electrophile coupling via electrochemistry, (B) C(sp2)–C(sp2) cross-electrophile coupling via thermal chemistry, (C) C–N coupling reaction using electrochemistry, and (D) C–H functionalization using HAT photocatalysis. | |
The groups of Weix,52 Hansen,52b,53 and Sevov,54 Reisman,55 amongst others,56 have each contributed notable advances in Ni-catalysed XEC beyond the early work by Périchon.57 These recent reports typically feature the use of two ligand systems (e.g. a bipyridine and a terpyridine ligand) and their relative ratio can be critical to the success of the reaction outcome.52b Recent works by Weix,52d Martin,58 Sigman and Doyle59 as well as others,60 have highlighted the importance of ligands that can stabilize Ni(I) catalytic intermediates. We sought to evaluate the usefulness of these N-cyano iminophosphoranes in XECs.52b
Scheme 5 reports a high throughput experimentation screen of 12 ligands for a nickel-catalyzed XEC between 4-(4-bromophenyl)morpholine (50) and 3-bromobutanenitrile (51) at 0 °C using Zn dust at the terminal reductant. The 12 ligands screened consisted of 4′-di-tert-butyl-2,2′-dipyridyl (dtbbpy) and 11 different N-cyano iminophosphoranes. The top three performing ligands were N-cyano iminophosphorane ligands 35 (derived from (S,S)-DACH), ligand 28 (based on DPPE), and ligands 37 (based on unsymmetrical triphos), providing assay yields of 76%, 65%, and 54%, respectively. A 1 mmol scale up of this XEC reaction using ligands 33 provided an assay yield of 88% yield.
Scheme 6A reports our results in exploring an electrochemically-driven C(sp2)–C(sp3) cross-electrophile coupling of ethyl 4-bromobenzoate (52) and 3-bromobutanenitrile (51) to access 53. The yield of desired XEC product 53 is tabulated in Scheme 6A along with key hydrodehalogenation impurity 54 and homocoupling side-product 55. We chose to focus our initial explorations using polydentate iminophosphorane ligands as described by our inspiration illustrated in Scheme 2 and the success of polydentate sp2-N-based ligands like 4,4′-di-tert-butyl-2,2′-dipyridyl (dtbbpy) or 4′′-tri-tert-butyl-2,2′:6′,2′′-terpyridine (ttbtpy) in Ni-catalysis. After testing a selection of polydentate iminophosphorane ligands (28, 31, 35, 37), we were delighted to obtain a high yield of product 53 with iminophosphorane ligands 31 and 37. The best-performing ligand was 37 providing 90% yield of XEC product 53 with, while minimizing impurities 54 and 55 to 3% and 6%, respectively. In comparison, the use of dtbbpy or ttbtpy on their own provided lower yields of 73% and 30% respectively.
Noteworthy, the use of the P(III) ligand DPPE provided only 3% yield of the XEC product 53 and the reaction mixture contained no evidence of DPPE at the end of the reaction, but instead the bis-oxide analog of DPPE was observed. Control experiments using the mono-oxide of DPPE (DPPEO) (or other bis-oxide ligands, see ESI† for details) as the ligand in the cross-electrophile coupling of 51 and 52 did not provide meaningful amounts of product 53. Specifically, 53 was obtained in 13% using DPPEO. This highlights why the P(V) iminophosphorane ligands are more suitable as they are less prone to oxidative decomposition pathways, unlike air/moisture-sensitive P(III) ligands, while also providing good catalytic activity unlike phosphine oxides.
sp2–sp2 XECs are also of interest as an alternative to Suzuki cross-couplings, bypassing the need for boronic acids which can be prone to protodeboronation.60 Weix has reported a dual catalytic system using nickel and palladium-catalysis to cross-couple aryl bromides with aryl triflates to access biaryls using Zn dust as the reductant.61 Employing this strategy, we successfully demonstrate that ligand 21 can enable the sp2–sp2 cross coupling of aryl bromide 50 with aryl triflate 56 to access product 57 in 64% yield (Scheme 6B).
To gain insight into the viability of using iminophosphorane ligands in high energy photochemical conditions (365 nm) with open shell species generated via hydrogen atom transfer (HAT) catalysis, we explored the use of MacMillan's metallaphotoredox method62 for direct arylation of C(sp3)–H bonds (Scheme 6C). Gratifyingly, irradiation of mixtures containing 2-trifluoromethyl-5-bromopyridine (58) and cyclohexane (59) in the presence of tetrakis(tetrabutylammonium)decatungstate ((n-Bu4N)4[W10O32], TTBADT), Ni(COD)(DQ)63 and iminophosphorane 31 with 365 nm provided the desired product 60 in useful yields.
Electrochemical Ni-catalyzed C–N cross couplings can offer milder conditions over their thermal counterpart (e.g. 20 °C instead of >110 °C).64 We explored the application of several iminophosphorane ligands (see ESI† for details) in the context of coupling aryl halide 61 with pyrrolidine (62) to access 63 (Scheme 6D) using electrochemical Ni-catalyzed conditions similar to those developed by Baran and coworkers.65 Gratifyingly, we obtained site selective coupling (Br over the activated Cl) to product 63 in 62% yield (and less than 5% yield of the product associated with C–N coupling at the 2-chloro position was observed). Interestingly, dtbbpy performed poorly for this substrate (22% yield of 58), further highlighting the importance of expanding the diversity of sp2-N-ligands towards improving selective cross-coupling reactions.
To demonstrate the scalability of the electrochemical synthesis of iminophosphoranes, we translated our batch electrosynthetic conditions to flow conditions (Scheme 7). Utilizing a parallel plate reactor equipped with carbon felt on graphite as the anode and a Pt cathode, we operated this flow electrolysis reactor in recirculating mode. By switching the electrolyte from Me4NOAc to the more soluble Bu4NOAc, and using carbon felt on graphite as the anode instead of graphite (increasing the surface area), we were able to increase the current density from 4.2 to 8.0 mA cm−2, and ultimately increase productivity. The use of 5.20 F mol−1 of charge provided complete consumption of both the starting material (DPPE) and the P(III)–P(V)-intermediate 39. The crude reaction mixture was simply diluted with water to induce a direct crystallization from the reaction mixture to isolate 28 (77% isolated yield, corrected for purity).
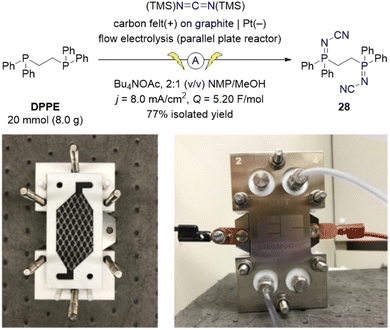 |
| Scheme 7 Multi-gram-scale flow-electrosynthesis of ligand 28 using an ElectroCell Micro Flow Cell® parallel plate reactor. Photographs illustrate the partially and fully assembled reactor. | |
Conclusions
We have developed an operationally simple and safe electrochemical synthesis of new iminophosphorane ligands from commercially available phosphines.66 A paired-electrolysis mechanism is operational where an electrogenerated base67 triggers the formation of a key intermediate to enable a rare example of domino electrolysis. This synthetic method is safer than conventional Staudinger chemistry (herein we would have required the use of cyanogen-azide, NC–N3, which is extremely hazardous due to its energetic nature, in fact it is a primary explosive).68 The utility of these compounds as ligands was demonstrated in five Ni-catalyzed cross-couplings, including C(sp2)–C(sp3) and C(sp2)–C(sp2) cross-electrophile couplings. Improved yields for the XEC of ethyl 4-bromobenzoate with 3-bromobutanenitrile were obtained when using iminophosphorane 31 or 37 compared to the commonly used dtbbpy and ttbtpy. Iminophosphorane ligand 21 also enabled site selectivity in a C–N coupling of a polyhalogenated pyridine derivative. This highlights the need of a diverse library of ligands to screen whenever optimizing for yields and selectivity in catalytic reactions. We anticipate broad applicability of these ligands for transition metal catalysis where sp2-nitrogen based ligands have traditionally been used (e.g. C–N, C–O, C–C cross-couplings). Applications of this electrosynthetic method to access iminophosphorane organocatalysts for anion-binding is also envisioned.20
Data availability
The ESI† contains the data associated with this article.
Author contributions
D. L. conceived the project. V. M., D. L., and Y.-h. L. directed the project. All the authors performed experiments. The manuscript was written through contributions of all authors.
Conflicts of interest
There are no conflicts to declare.
Acknowledgements
V. M. and M. T. C. acknowledge support from the Postdoctoral Fellowship program at Merck & Co., Inc., Rahway, NJ, USA. We acknowledge Ryan Cohen and Mikhail Reibarkh for assistance with the 13C{1H,31P} NMR experiment for ligand 28. We thank François Lévesque, Charles Yeung, and Eric Phillips (all at Merck & Co., Inc., Rahway, NJ, USA) for insightful discussions and feedback.
Notes and references
-
(a) A. L. Clevenger, R. M. Stolley, J. Aderibigbe and J. Louie, Chem. Rev., 2020, 120, 6124–6196 CrossRef CAS PubMed;
(b) M. B. Smith, Phosphorus Ligands, Reference Module in Chemistry, Molecular Sciences and Chemical Engineering, 2013 DOI:10.1016/B978-0-12-409547-2.01037-4;
(c)
E. I. Musina, A. S. Balueva and A. A. Karasik, Tertiary Phosphines: Preparation, in Organophosphorus Chemistry, 2022, vol. 51, pp. 1–61, 10.1039/9781839166198-00001.
- H. Guo, Y. C. Fan, Z. Sun, Y. Wu and O. Kwon, Chem. Rev., 2018, 118, 10049–10293 CrossRef CAS PubMed.
- D. Parmar, S. Raja and M. Rueping, Chem. Rev., 2014, 114, 9047–9153 CrossRef CAS PubMed.
-
(a) H. Li, K. M. Belyk, J. Yin, Q. Chen, A. Hyde, Y. Ji, S. Oliver, M. T. Tudge, L.-C. Campeau and K. R. Campos, J. Am. Chem. Soc., 2015, 137, 13728–13731 CrossRef CAS PubMed;
(b) Y. Ji, H. Li, A. M. Hyde, Q. Chen, K. M. Belyk, K. W. Lexa, J. Yin, E. C. Sherer, R. T. Williamson, A. Brunskill, S. Ren, L. C. Campeau, I. W. Davies and R. T. Ruck, Chem. Sci., 2017, 8, 2841–2851 RSC.
-
(a) Y. Ji, E. P. Plata, C. S. Regens, M. Hay, M. Schmidt, T. Razler, Y. Qiu, P. Geng, Y. Hsiao, T. Rosner, M. D. Eastgate and D. G. Blackmond, J. Am. Chem. Soc., 2015, 137, 13272–13281 CrossRef CAS PubMed;
(b) R. J. Fox, N. L. Cuniere, L. Bakrania, C. Wei, N. A. Strotman, M. Hay, D. Fanfair, C. Regens, G. L. Beutner, M. Lawler, P. Lobben, M. C. Soumeillant, B. Cohen, K. Zhu, D. Skliar, T. Rosner, C. E. Markwalter, Y. Hsiao, K. Tran and M. D. Eastgate, J. Org. Chem., 2019, 84, 4661–4669 CrossRef CAS PubMed.
- J. I. Murray, L. Zhang, A. Simon, M. V. S. Elipe, C. S. Wei, S. Caille and A. T. Parsons, Org. Process Res. Dev., 2023, 27, 198–205 CrossRef CAS.
-
(a) S. E. Denmark, R. C. Smith and S. A. Tymonko, Tetrahedron, 2007, 63, 5730–5738 CrossRef CAS PubMed;
(b) A. K. King, A. Brar and M. Findlater, Catal. Sci. Technol., 2023, 13, 301–304 RSC.
- A. Côté, (R,R)-Me-DuPHOS Monoxide. e-EROS Encycl. Reagents, Org. Synth., 2010 DOI:10.1002/047084289X.rn01242.
- S. Kotani, Y. Yoshiwara, M. Ogasawara, M. Sugiura and M. Nakajima, Angew. Chem., Int. Ed., 2018, 57, 15877–15881 CrossRef CAS PubMed.
- E. Mao, C. K. Chung, Y. Ji, Y.-h. Lam and P. E. Maligres, J. Org. Chem., 2021, 86, 7529–7536 CrossRef CAS PubMed.
-
(a) S. E. García-Garrido, A. P. Soto and J. García-Álvarez, Adv. Organomet. Chem., 2022, 77, 105–168 CrossRef;
(b) J. García-Álvarez, S. E. García-Garrido and V. Cadierno, J. Organomet. Chem., 2014, 751, 792–808 CrossRef;
(c) A. Jain, H. Karmakar, P. W. Roesky and T. K. Panda, Chem. Rev., 2023, 123, 13323–13373 CrossRef CAS PubMed.
- A. Arques, D. Auñon and P. Molina, Tetrahedron Lett., 2004, 45, 4337–4340 CrossRef CAS.
- R. Venkateswaran, M. S. Balakrishna and S. M. Mobin, Eur. J. Inorg. Chem., 2007, 1930–1938 CrossRef CAS.
-
(a) Z.-X. Wang and L. Wang, Chem. Commun., 2007, 2423–2425 RSC;
(b) C. Zhang and Z.-X. Wang, Organometallics, 2009, 28, 6507–6514 CrossRef CAS.
-
(a) D. J. Law and R. G. Cavell, J. Mol. Catal., 1994, 91, 175–186 CrossRef CAS;
(b) T. T. Co, S. C. Shim, C. S. Cho, T. J. Kim, S. O. Kang, W.-S. Han, J. Ko and C.-K. Kim, Organometallics, 2005, 24, 4824–4831 CrossRef CAS;
(c) S. Al-Benna, M. J. Sarsield, M. Thornton-Pett, D. L. Ormsby, P. J. Maddox, P. Bres and M. Bochmann, J. Chem. Soc., Dalton Trans., 2000, 4247–4257 RSC.
-
(a) A. Buchard, H. Heuclin, A. Auffrant, X. F. Le Goff and P. Le Floch, Dalton Trans., 2009, 1659–1667 RSC;
(b) A. Buchard, E. Payet, A. Auffrant, X. Le Goff and P. Le Floch, New J. Chem., 2010, 34, 2943–2949 RSC.
- V. D. M. Hoang, P. A. N. Reddy and T.-J. Kim, Tetrahedron Lett., 2007, 48, 8014–8017 CrossRef CAS.
-
(a) L. Beaufort, F. Benvenuti, L. Delaude and A. F. Noels, J. Mol. Catal. A: Chem., 2008, 283, 77–82 CrossRef CAS;
(b) O. Alhomaidan, G. Bai and D. W. Stephan, Organometallics, 2008, 27, 6343–6352 CrossRef CAS;
(c) D. Bézier, O. Daugulis and M. Brookhart, Organometallics, 2017, 36, 2947–2951 CrossRef;
(d) E. Martinez-Arripe, F. Jean-Baptiste-dit-Dominique, A. Auffrant, X.-F. Le Goff, J. Thuilliez and F. Nief, Organometallics, 2012, 31, 4854–4861 CrossRef CAS;
(e) Z. Jian, A. R. Petrov, N. K. Hangaly, S. Li, W. Rong, Z. Mou, K. A. Rufanov, K. Harms, J. Sundermeyer and D. Cui, Organometallics, 2012, 31, 4267–4282 CrossRef CAS;
(f) T. Cheisson, T.-P.-A. Cao, X. F. Le Goff and A. Auffrant, Organometallics, 2014, 33, 6193–6399 CrossRef CAS.
-
(a) E. M. Broderick, N. Guo, C. S. Vogel, C. Xu, J. Sutter, J. T. Miller, K. Meyer, P. Mehrhodavandi and P. Diaconescu, J. Am. Chem. Soc., 2011, 133, 9278–9281 CrossRef CAS PubMed;
(b) T.-P. Cao, A. Buchard, X. F. Le Goff, A. Auffrant and C. K. Williams, Inorg. Chem., 2012, 51, 2157–2169 CrossRef CAS PubMed;
(c) N. Yuntawattana, T. M. McGuire, C. B. Durr, A. Buchard and C. K. Williams, Catal. Sci. Technol., 2020, 10, 7226–7239 RSC;
(d) M. T. Gamer, P. W. Roesky, I. Palard, M. Le Hellaye and S. M. Guillaume, Organometallics, 2007, 26, 651–657 CrossRef CAS;
(e) Z. Mou, B. Liu, X. Liu, H. Xie, W. Rong, L. Li, S. Li and D. Cui, Macromolecules, 2014, 47, 2233–2241 CrossRef CAS;
(f) H. Xie, Z. Mou, B. Liu, P. Li, W. Rong, S. Li and D. Cui, Organometallics, 2014, 33, 722–730 CrossRef CAS.
-
(a) M. G. Núñez, A. J. M. Farley and D. J. Dixon, J. Am. Chem. Soc., 2013, 135, 16348–16351 CrossRef PubMed;
(b) M. Formica, D. Rozsar, G. Su, A. J. M. Farley and D. J. Dixon, Acc. Chem. Res., 2020, 53, 2235–2247 CrossRef CAS PubMed;
(c) X. Gao, J. Han and L. Wang, Org. Lett., 2015, 17, 4596–4599 CrossRef CAS PubMed;
(d) N. A. Richard, G. D. Charlton and C. A. Dyker, Org. Biomol. Chem., 2021, 19, 9167–9171 RSC;
(e) M. Formica, T. Rogova, H. Shi, N. Sahara, B. Ferko, A. J. M. Farley, K. E. Christensen, F. Duarte, K. Yamazaki and D. J. Dixon, Nat. Chem., 2023, 15, 714–721 CrossRef CAS PubMed;
(f) S. Kotani, K. Yoshiwara, M. Ogasawara, M. Sugiura and M. Nakajima, Angew. Chem., Int. Ed., 2018, 26, 15877–15881 CrossRef PubMed.
- S. S. Hanson, E. Doni, K. T. Traboulsee, G. Coulthard, J. A. Murphy and A. Dyker, Angew. Chem., Int. Ed., 2015, 54, 11236–11239 CrossRef CAS PubMed.
-
(a) C. Bdenarek, I. Wehl, N. Jung, U. Schepers and S. Bräse, Chem. Rev., 2020, 120, 4301–4354 CrossRef PubMed;
(b) Y. G. Gololobov and L. F. Kasukhin, Tetrahedron, 1992, 48, 1353–1406 CrossRef CAS.
- A. V. Kirsanov, Izv. Akad. Nauk SSSR, Ser. Khim., 1954, 646–655 CAS.
-
(a) L. Horner and H. Oediger, Liebigs Ann., 1959, 627, 142–162 CrossRef;
(b) A. V. Kirsanov, Izv. Akad. Nauk SSSR, 1950, 426–437 CAS.
- S. Bittner, M. Pomerantz, Y. Assaf, P. Krief, S. Xi and M. K. Witczak, J. Org. Chem., 1988, 53, 1–5 CrossRef CAS.
-
Bretherick's Handbook of Reactive Chemical Hazards, ed. P. G. Urban, Butterworth-Heinemann, Ltd, Oxford, 6th edn, 1999. DOI:10.1016/C2009-0-24106-7.
-
(a) H. Morita, A. Tatami, T. Maeda, B. J. Kim, W. Kawashima, T. Yoshimura and H. A. T. Akasaka, J. Org. Chem., 2008, 73, 7159–7163 CrossRef CAS PubMed;
(b) J.-J. Tang, X. Yu, Y. Wang, Y. Yamamoto and M. Bao, Angew. Chem., Int. Ed., 2021, 60, 16426–16435 CrossRef CAS PubMed.
- S. Lin, B. Lin, Z. Zhang, J. Chen, Y. Luo and Y. Xia, Org. Lett., 2022, 24, 3302–3306 CrossRef CAS PubMed.
- E. Falk, A. Franchino, T. Horak, L. Gürtler and B. Morandi, Org. Lett., 2023, 25, 1695–1700 CrossRef CAS PubMed.
-
(a) N. E. S. Tay, D. Lehnherr and T. Rovis, Chem. Rev., 2022, 122, 2487–2649 CrossRef CAS PubMed;
(b) M. Yan, Y. Kawamata and P. S. Baran, Chem. Rev., 2017, 117, 13230–13319 CrossRef CAS PubMed;
(c) A. Wiebe, T. Gieshoff, S. Mohle, E. Rodrigo, M. Zirbes and S. R. Waldvogel, Angew. Chem., Int. Ed., 2018, 57, 5594–5619 CrossRef CAS PubMed;
(d) B. Cohen, D. Lehnherr, M. Sezen-Edmonds, J. H. Forstater, M. O. Fredrick, L. Deng, A. C. Ferretti, K. Harper and M. Diwan, Chem. Eng. Res. Des., 2023, 192, 622–637 CrossRef CAS;
(e) D. Lehnherr and L. Chen, Org. Process Res. Dev., 2024, 28, 338–366 CrossRef CAS.
-
(a) E. C. Hansen, C. Li, S. Yang, D. Pedro and D. J. Weix, J. Org. Chem., 2017, 82, 7085–7092 CrossRef CAS PubMed;
(b) S. Kim, M. J. Goldfogel, M. M. Gilbert and D. J. Weix, J. Am. Chem. Soc., 2020, 142, 9902–9907 CrossRef CAS PubMed;
(c) A. L. Aguirre, N. L. Loud, K. A. Johnson, D. J. Weix and Y. Wang, Chem.–Eur. J., 2021, 27, 12981–129886 CrossRef CAS PubMed.
-
(a) J. Rein, J. R. Annand, M. K. Wismer, J. Fu, J. C. Siu, A. Klapars, N. A. Strotman, D. Kalyani, D. Lehnherr and S. Lin, ACS Cent. Sci., 2021, 7, 1347–1355 CrossRef CAS PubMed;
(b)
J. Rein, S. Lin, D. Kalyani and D. Lehnherr, High-Throughput Experimentation for Electrochemistry, in The Power of High-Throughput Experimentation, ed. D. C. Leitch, M. Jouffroy and M. Emmert, ACS Symposium Series, American Chemical Society, 2023, vol. 1419, pp. 167–187, DOI:10.1021/bk-2022-1419.ch010.
-
HTe−Chem Electrochemistry, Analytical Sales and Services, Inc., https://www.analytical-sales.com/product-category/photoredox-parallel-synthesis/hte-chem-electrochemistry/, accessed April 15, 2023.
- Both NMP and the tetraalkylammonium acetate supporting electrolyte are hygroscopic and were used as is without further drying prior to their use.
-
(a) F. Cozzi, Adv. Synth. Catal., 2006, 348, 1367–1390 CrossRef CAS;
(b)
S. Itsuno and N. Haraguchi, Chapter 2: Catalysts Immobilized onto Polymers, in Catalyst Immobilization: Methods and Applications, ed. M. Benaglia and A. Puglis, Wiley, 2019, DOI:10.1002/9783527817290.ch2.
- T. Tannoux and A. Auffrant, Coord. Chem. Rev., 2023, 474, 214845 CrossRef CAS.
-
(a)
Privileged Chiral Ligands and Catalysts, ed. Q.-L. Zhou, Wiley, 2011, DOI:10.1002/9783527635207;
(b) T. P. Yoon and E. N. Jacobsen, Science, 2003, 299, 1691–1693 CrossRef CAS PubMed.
- F. Richard, P. Clark, A. Hannam, T. Keenan, A. Jean and S. Arseniyadis, Chem. Soc. Rev., 2024, 53, 1936–1983 RSC.
- We note that “triphos” has been used to described both “1,1,1-tris(diphenylphosphinomethyl)ethane” and “bis(diphenylphosphinoethyl)phenylphosphine” in the literature.
-
(a)
G. Huttner, J. Strittmatter and S. Sandhoefner, Phosphorus Tripodal Ligands, in Compr. Coord. Chem. II, 2003, vol. 1, pp. 297–322, DOI:10.1016/B0-08-043748-6/01082-3;
(b) T. A. Betley and J. C. Peters, J. Am. Chem. Soc., 2003, 125, 10782–10783 CrossRef CAS PubMed.
-
T. Güthner and B. Mertschenk, Cyanamides, in Ullmann's Encyclopedia of Industrial Chemistry, Wiley-VCH, Weinheim, 2006, DOI:10.1002/14356007.a08_139.pub2.
- NBO analysis predicted the spin density is localized at the phosphorus preferentially over being delocalized into the arene (see ESI† Table S14).
- For an example of a domino electrolysis reaction, see previous reports on converting aldoximes to nitriles via oxidation to a nitrile oxide intermediate and subsequent reduction:
(a) T. Shono, Y. Matsumura, K. Tsubata, T. Kamada and K. Kishi, J. Org. Chem., 1989, 54, 2249–2251 CrossRef CAS;
(b) M. F. Hartmer and S. R. Waldvogel, Chem. Commun., 2015, 51, 16346–16348 RSC.
- For a 2nd example of a domino electrochemical reaction, see: X. Dong, J. L. Roeckl, S. R. Waldvogel and B. Morandi, Science, 2021, 371, 507–514 CrossRef CAS PubMed.
-
T. Wu and K. D. Moeller, Paired Electrolysis, in Science of Synthesis: Electrochemistry in Organic Synthesis, 2021, vol. 1, p. 481, DOI:10.1055/sos-SD-236-00331.
- C. A. Tolman, Chem. Rev., 1977, 77, 313–348 CrossRef CAS.
- D. G. Gusev, Organometallics, 2009, 28, 763–770 CrossRef CAS.
- We note that limitations are known regarding the interpretation of TEP values, see: D. Cremer and E. Kraka, Dalton Trans., 2017, 46, 8323–8338 RSC.
- Deposition numbers CCDC 2259467 (for 4), 2259466 (for (R)-25), 2258974 (for 13), 2258975 (for 24), 2258976 (for 28), 2258977 (for (S)-33), 2258978 (for 38), 2258979 (for 39), 2258980 (for 29), and 2329725 (for Ni-1), contain the supplementary crystallographic data for this paper.
- L. R. Favello, S. Fernández, M. M. García, R. Navarro and E. P. Urriolabeitia, J. Chem. Soc., Dalton Trans., 1998, 3745–3750 RSC.
-
(a) S. Al-Benna, M. J. Sarsfield, M. Thornton-Pett, D. L. Ormsby, P. J. Maddox, P. Brès and M. Bochmann, J. Chem. Soc., Dalton Trans., 2000, 4247–4257 RSC;
(b) T. Cheisson, T.-P.-A. Cao, X. F. Le Goff and A. Auffrant, Organometallics, 2014, 33, 6193–6199 CrossRef CAS.
-
(a) D. J. Weix, Acc. Chem. Res., 2015, 48, 1767–1775 CrossRef CAS PubMed;
(b) M. C. Franke, V. R. Longley, M. Rafiee, S. S. Stahl, E. C. Hansen and D. J. Weix, ACS Catal., 2022, 12, 12617–12626 CrossRef CAS PubMed;
(c) S. Kim, M. . J. Goldfogel, M. M. Gilbert and D. J. Weix, J. Am. Chem. Soc., 2020, 142, 9902–9907 CrossRef CAS PubMed;
(d) P. E. Piszel, B. Orzolek, A. K. Olszewski, M. Rotella, A. M. Spiewak, M. Kozlowski and D. J. Weix, J. Am. Chem. Soc., 2023, 145, 8517–8528 CAS.
-
(a) R. J. Perkins, D. J. Pedro and E. C. Hansen, Org. Lett., 2017, 19, 3755–3758 CrossRef CAS PubMed;
(b) R. J. Perkins, A. J. Hughes, D. J. Weix and E. C. Hansen, Org. Process Res. Dev., 2019, 23, 1746–1751 CrossRef CAS.
-
(a) T. B. Hamby, M. J. LaLama and C. S. Sevov, Science, 2022, 376, 410–416 CrossRef CAS PubMed;
(b) J. L. S. Zackasee, S. Al Zubaydi, B. L. Truesdell and C. S. Sevov, ACS Catal., 2022, 12, 1161–1166 CrossRef CAS PubMed;
(c) B. L. Truesdell, T. B. Hamby and C. S. Sevov, J. Am. Chem. Soc., 2020, 142, 5884–5893 CrossRef CAS PubMed.
- T. J. DeLano and S. E. Reisman, ACS Catal., 2019, 9, 6751–6754 CrossRef CAS PubMed.
-
(a) C. E. I. Knappke, S. Grupe, D. Gärtner, M. Corpet, C. Gosmini and A.
J. von Wangelin, Chem.–Eur. J., 2014, 6828–6842 CrossRef CAS PubMed;
(b) D. J. Charboneau, H. Huang, E. L. Barth, C. C. Germe, N. Hazari, B. Q. Mercado, M. R. Uehling and S. L. Zultanski, J. Am. Chem. Soc., 2021, 143(49), 21024–21036 CrossRef CAS PubMed;
(c) H. A. Sakai, W. Liu, C. C. Le and D. W. C. MacMillan, J. Am. Chem. Soc., 2020, 142, 11691–11697 CrossRef CAS PubMed;
(d) F. Chen, K. Chen, Y. Zhang, Y. He, Y.-M. Wang and S. Zhu, J. Am. Chem. Soc., 2017, 139, 13929–13935 CrossRef CAS PubMed;
(e) J. Zhou, D. Wang, W. Xu, Z. Hu and T. Xu, J. Am. Chem. Soc., 2023, 145, 2081–2087 CrossRef CAS PubMed;
(f) W. Zhang, L. Lu, W. Zhang, Y. Wang, S. D. Ware, J. Mondragon, J. Rein, N. Strotman, D. Lehnherr, K. A. See and S. Lin, Nature, 2022, 604, 292–297 CrossRef CAS PubMed;
(g) J. Liao, C. H. Basch, M. E. Hoerrner, M. R. Talley, B. P. Boscoe, J. W. Tucker, M. R. Garnse and M. P. Watson, Org. Lett., 2019, 21, 2941–2946 CrossRef CAS PubMed;
(h) J. Twilton, M. R. Johnson, V. Sidana, M. C. Franke, C. Bottecchia, D. Lehnherr, F. Lévesque, S. M. M. Knapp, L. Wang, J. B. Gerken, C. M. Hong, T. P. Vickery, M. D. Weisel, N. A. Strotman, D. J. Weix, T. W. Root and S. S. Stahl, Nature, 2023, 623, 71–76 CrossRef CAS PubMed.
-
(a) A. Conan, S. Sibille, E. d'Incan and J. Périchon, J. Chem. Soc. Chem. Commun., 1990, 48–49 RSC;
(b) M. Durandetti, J.-Y. Nédélec and J. Périchon, J. Org. Chem., 1996, 61, 1748–1755 CrossRef CAS PubMed;
(c) M. Durandetti, J. Périchon and J.-Y. Nédélec, J. Org. Chem., 1997, 62, 7914–7915 CrossRef CAS PubMed.
- C. S. Day, A. Rentería-Gómez, S. J. Ton, A. R. Gogoi, O. Gutierrez and R. Martin, Nat. Catal., 2023, 6, 244–253 CrossRef CAS.
- T. Tang, A. Hazra, D. S. Min, W. L. Williams, E. Jones, A. G. Doyle and M. S. Sigman, J. Am. Chem. Soc., 2023, 145, 8689–8699 CAS.
-
(a) P. A. Cox, A. G. Leach, A. D. Campbell and G. C. Lloyd-Jones, J. Am. Chem. Soc., 2016, 138, 9145–9157 CrossRef CAS PubMed;
(b) P. A. Cox, M. Reid, A. G. Leach, A. D. Campbell, E. J. King and G. C. Lloyd-Jones, J. Am. Chem. Soc., 2017, 139, 13156–13165 CrossRef CAS PubMed.
- L. . K. . G. Ackerman, M. M. Lovell and D. J. Weix, Nature, 2015, 524, 454–457 CrossRef CAS PubMed.
- I. B. Perry, T. F. Brewer, P. J. Sarver, D. M. Schultz, D. A. DiRocco and D. W. C. MacMillan, Nature, 2018, 560, 70–75 CrossRef CAS PubMed.
-
(a) V. T. Tran, N. Kim, C. Z. Rubel, X. Wu, T. Kang, T. C. Jankins, Z.-Q. Li, M. V. Joannou, S. Ayers, M. Gembicky, J. Bailey, E. J. Sturgell, B. B. Sanchez, J. S. Chen, S. Lin, M. D. Eastgate, S. R. Wisniewski and K. M. Engle, Angew. Chem., Int. Ed., 2023, 62, e202211794 CrossRef CAS PubMed;
(b) C. Z. Rubel, W.-J. He, S. R. Wisniewski and K. M. Engle, Acc. Chem. Res., 2024, 57, 312–326 CrossRef CAS PubMed.
- H. Hu, A. N. Singh, D. Lehnherr, V. Mdluli, S. W. Chun, A. M. Makarewicz, J. R. Gouker, O. Ukaegbu, S. Li, X. Wen, D. G. McLaren, J. E. Velasquez, J. C. Moore, S. Galanie, E. Appiah-Amponsah and E. L. Regalado, Anal. Chem., 2024, 96, 1138–1146 CrossRef CAS PubMed.
-
(a) C. Li, Y. Kawamata, H. Nakamura, J. C. Vantourout, Z. Liu, Q. Hou, D. Bao, J. T. Starr, J. hen, M. Yan and P. S. Baran, Angew. Chem., Int. Ed., 2017, 56, 13088–13093 CrossRef CAS PubMed;
(b) Y. Kawamata, J. C. Vantourout, D. P. Hickey, P. Bai, L. Chen, Q. Hou, W. Qiao, K. Barman, M. A. Edwards, A. F. Garrido-Castro, J. N. deGruyter, H. Nakamura, K. Knouse, C. Qin, K. J. Clay, D. Bao, C. Li, J. T. Starr, C. Garcia-Irizarry, N. Sach, H. S. White, M. Neurock, S. D. Minteer and P. S. Baran, J. Am. Chem. Soc., 2019, 141, 6392–6402 CrossRef CAS PubMed;
(c) S. Sengmany, F. Daili, I. Kribii and E. Léonel, J. Org. Chem., 2023, 88, 675–683 CrossRef CAS PubMed.
- After a pre-print of this work was posted online, a different electrochemical approach to N-aryl iminophosphoranes was reported, see:
(a)
V. Mdluli, D. Lehnherr, Y.-h. Lam, M. T. Chaudhry, J. A. Newman, J. O. DaSilva and E. L. Regalado, ChemRxiv, 2023, preprint, DOI:10.26434/chemrxiv-2023-f7d2q;
(b) R. C. e. Silva, C. Vega, M. Regnier, L. Capaldo, L. Wesenberg, G. Lowe, K. T. de Oliveira and T. Noël, Adv. Synth. Catal., 2024, 366, 955–960 CrossRef.
-
S. Kashimura and K. Matsumoto, Electrogenerated Base, in Encyclopedia of Applied Electrochemistry, ed. G. Kreysa, K. Ota and R. F. Savinell, Springer, 2014, New York, NY, DOI:10.1007/978-1-4419-6996-5_354.
-
(a) F. D. Marsh and E. Hermes, J. Am. Chem. Soc., 1964, 86, 4506–4507 CrossRef CAS;
(b)
D. Goldsmith, Cyanogen azide, in Encyclopedia of Reagents for Organic Synthesis, E-EROS Encyclopedia of Reagents for Organic Synthesis, 2001, DOI:10.1002/047084289X.rc268, 978-0471936237;
(c) F. D. Marsh, J. Org. Chem., 1972, 37, 2966–2969 CrossRef CAS;
(d)
R. Matyáš, J. Pachman, Primary Explosives, Springer Science & Business Media, 12 March 2013, p. 111 CrossRef;
(e)
M. L. Madigan, First Responders Handbook: An Introduction, CRC Press, Second Edition, 13 September 2017, p. 170 CrossRef.
|
This journal is © The Royal Society of Chemistry 2024 |
Click here to see how this site uses Cookies. View our privacy policy here.