DOI:
10.1039/D3SC06599E
(Edge Article)
Chem. Sci., 2024,
15, 3495-3501
Rare-earth metal ethylene and ethyne complexes†
Received
8th December 2023
, Accepted 26th January 2024
First published on 31st January 2024
Abstract
Guanidinate homometallic rare-earth ethyl complexes [LLn(μ2-η1:η2-Et)(Et)]2 (Ln = Y(1-Y), Lu(1-Lu)) and heterobimetallic rare-earth ethyl complexes LLn(Et)(μ2-η1:η2-Et)(μ2-η1-Et)(AlEt2) (Ln = Y(2-Y), Lu(2-Lu)) have been synthesized by the treatment of LLn(CH2C6H4NMe2-o)2 (L = (PhCH2)2NC(NC6H3iPr2-2,6)2) with different equivalents of AlEt3 in toluene at ambient temperature. Interestingly, the unprecedented rare-earth ethyne complex [LY(μ2-η1-Et)2(AlEt)]2(μ4-η1:η1:η2:η2-C2H2) (3-Y) containing a [C2H2]4− unit was afforded from 2-Y. The formation mechanism study on 3-Y was carried out by DFT calculations. Furthermore, the nature of the bonding of 3-Y was also revealed by NBO analysis. The reactions of LLn(CH2 C6H4NMe2-o)2 (Ln = Y, Lu) with AlEt3 (4 equiv.) in toluene at 50 °C produced firstly the non-Cp rare-earth ethylene complex LY(μ3-η1:η1:η2-C2H4)[(μ2-η1-Et)(AlEt2)(μ2-η1-Et)2(AlEt)] (4-Y), and the Y/Al ethyl complex LY[(μ2-η1-Et)2(AlEt2)]2 (5-Y) as an intermediate of 4-Y was isolated from the reaction of LY(CH2C6H4NMe2-o)2 with AlEt3 (4 equiv.) in toluene at −10 °C.
Introduction
Impressive progress has been witnessed in the development of the design and synthesis of new rare-earth alkyl complexes in the last few decades owing to their high activity in a wide range of stoichiometric and catalytic reactions.1–3 However, studies on the alkylidene and alkylidyne complexes are limited due to the HOMO/LUMO orbital energy mismatch between the rare-earth metal ions and carbon-centered orbitals,4–12 among which the deprotonation of AlMe3 and thermally induced methyl ligand degradation have been proven to be effective ways for the generation of CH22− and CH3− moieties. However, to our knowledge, compared to methyl congeners, only a few examples of rare-earth ethyl complexes and their derivatives have been isolated and structurally authenticated.13–16 Particularly, the rare-earth ethyl complexes engage in further degradation reactions, as shown for β-hydrogen abstraction as well as β-alkyl transfer, which are extremely limited,17 while the first ethylene complex prepared by Kaminsky's group was identified as the Zr–CH2CH2–Zr and Zr–CH2CH2–Al moieties generated by the reaction of Cp2ZrCl2 and AlEt3 in 1974.18 Bercaw et al. isolated the first ethylene bridged binuclear scandium complex {(C5Me4SiMe2 NtBu)Sc(PMe3)}2(μ-C2H4)17a (Scheme 1A). Anwander and co-workers reported the lanthanum ethyl complex La[(Et3Al)(μ-CH2CH2)(AlEt2)(μ-CH2CH2)(AlEt3)] containing two ethylene moieties17b (Scheme 1B). So the study on the chemistry of rare-earth ethylene complexes is still in its infancy; we became intensely interested in the synthetic strategies and the possible reactivity of the rare-earth ethylene complexes. In addition, we are also curious about whether the rare-earth ethyne complexes can be prepared from the rare-earth ethylene complexes via the C–H bond activation or other pathways. To our knowledge, in contrast to the late-transition metal ethylene and ethyne complexes,19,20 the early-transition metal ethylene analogues are less explored,18,21 and no rare earth metal ethyne complexes have been reported. With this in mind, we carried out the studies on the synthesis of rare-earth metal ethylene and ethyne complexes.
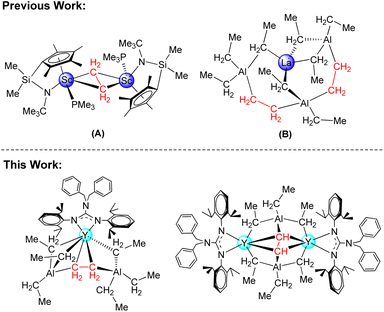 |
| Scheme 1 Rare-earth ethylene and ethyne complexes. | |
In this paper, distinctive guanidinato-based rare-earth ethylene and ethyne complexes were synthesized by controlling the molar ratio of the guanidinate dialkyl complexes22 with AlEt3. Moreover, the synthesis and bonding analysis (DFT) of the first rare-earth metal ethyne complex were studied.
Results and discussion
Synthesis and structural characterization
Firstly, the homoleptic rare-earth ethyl dimers [LLn(μ2-η1:η2-Et)(Et)]2 (Ln = Y(1-Y, 71%), Lu(1-Lu, 78%)) were afforded when two equivalents of AlEt3 were added to a toluene solution of dialkyl complexes at ambient temperature (Scheme 2). In the 1H VT NMR spectra of 1, only one set of signals assigned to ethyl groups was observed, evidencing the rapid exchange of the ethyl-bridged and the terminal ethyl units in the solvent (Fig. S3 and S6†). The peak at δ = 0.59 ppm for 1-Y (0.86 ppm for 1-Lu) is assignable to Ln−CH2CH3, and the resonance of CH2CH3 exhibits a peak at δ = 1.49 ppm for 1-Y (1.51 ppm for 1-Lu). The carbon atom signals of ethyl at δ = 40.9, 13.1 ppm for 1-Y (46.8, 13.4 ppm for 1-Lu) in the 13C{1H} NMR spectra can be considered. It is noteworthy that no hydrogen abstraction products were isolated when 1 were heated in toluene up to 70 °C for 12 h. The molecular structure of 1-Lu is also characterized by the single-crystal X-ray diffraction (Fig. 1). The bridged ethyl units of 1-Lu display the μ-η1:η2-ethyl bonding to the lutetium center. This coordination mode has previously been observed in the divalent Yb complex (C5Me5)2Yb(μ-η1:η2-Et)AlEt2(THF)15 and the trivalent Sm derivatives (C5Me5)2Sm(THF)(μ-η1:η2-Et)AlEt3 (ref. 16a) and [(C5Me5)2Sm]2[(μ-η1:η2-Et)2(μ-η1-Et)2Al4Et6(μ3-O)2].16b
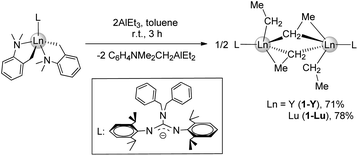 |
| Scheme 2 Synthesis of binuclear homometallic rare-earth ethyl complexes 1. | |
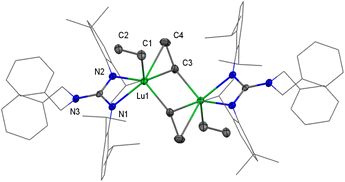 |
| Fig. 1 Molecular structure of 1-Lu with thermal ellipsoids at 30% probability. All hydrogen atoms are omitted for clarity. Selected bond distances (Å) and angles (°): Lu(1)–C(1) 2.332(4), Lu(1)–C(3) 2.435(4), Lu(1)–C(4) 2.848(5), C(1)–C(2) 1.531(6), C(3)–C(4) 1.528(6), Lu(1)–N(1) 2.275(3), Lu(1)–N(2) 2.296(3); Lu(1)–C(1)–C(2) 124.4(3), Lu(1)–C(3)–C(4) 88.8(3), C(1)–Lu(1)–C(3) 113.83(17), C(1)–Lu(1)–C(4) 90.88(17). | |
According to the relevant reports of heterobimetallic Y/Al methyl complexes,23 we might get a series of unique structural rare-earth ethyl complexes and their derivatives by controlling the amount of AlEt3. Thus, the treatment of LLn(CH2C6H4NMe2-o)2 (Ln = Y, Lu) with AlEt3 (3 equiv.) in toluene provided the heterobimetallic mixed ethyl/tetraethylaluminate complexes LLn(Et)(μ2-η1:η2-Et)(μ2-η1-Et)(AlEt2) (Ln = Y(2-Y for 83%), Lu(2-Lu for 90%)) (Scheme 3). In the NMR spectra of 2, the distinct differences of chemical shifts of [AlEt4]− moieties (1.30(CH3) and 0.33(CH2) for 2-Y; 1.28(CH3) and 0.53(CH2) for 2-Lu) and end-on-coordinated ethyl units (Ln-CH2CH3: 1.78(CH3) and 0.85(CH2) for 2-Y; 1.84(CH3) and 0.86(CH2) for 2-Lu) are investigated based on a comprehensive analysis of the 13C DEPT-135 NMR and HMQC-NMR spectra. Additionally, the carbon atom signals of tetraethylaluminate moieties (10.8(CH2), 10.5(CH3) for 2-Y; 12.8(CH2), 10.9(CH3) for 2-Lu) and end-on-coordinated ethyl ligands (39.1(d, JYC = 56 Hz, CH2), 14.1(CH3) for 2-Y; 44.2(CH2), 14.4(CH3) for 2-Lu) in the 13C{1H} NMR spectra can be detected. The 89Y NMR spectrum of 2-Y has a resonance at δ = 850.1 ppm that is within the wide range found for the reported organometallic yttrium complexes (ESI, Fig. S11†).24
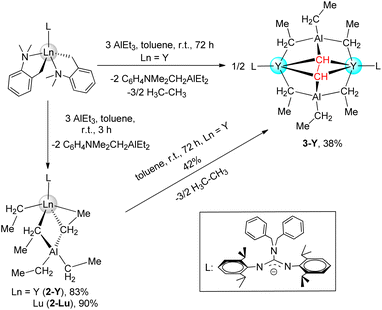 |
| Scheme 3 Synthesis of heterobimetallic rare-earth ethyl complexes 2 and ethyne complex 3-Y. | |
Complexes 2 are stable under an inert atmosphere at room temperature and sparingly soluble in n-hexane, however, they readily dissolve in aromatic solvents. The solid-state molecular structures of 2 were determined by X-ray diffraction analysis (Fig. 2). The Ln–CH2CH3(terminal) bond length (2.335(7) Å for 2-Y and 2.308(8) for 2-Lu) lies within the expected range.13c,14a,b Interestingly, the bridged ethyl units of [AlEt4]− display the μ-η1-Et and μ-η1:η2-Et bonding to the rare-earth center, and a Ln⋯H3C agostic interaction (Y–C–C: 85.8(3)°; Lu−C–C: 85.0(2)°) is likely to be present in the μ-η1:η2-Et bond mode.14d,e
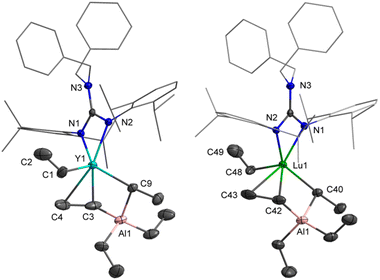 |
| Fig. 2 Molecular structures of complexes 2-Y (left) and 2-Lu (right) with thermal ellipsoids at 30% probability. All hydrogen atoms are omitted for clarity. Selected bond distances (Å) and angles (°): 2-Y: Y(1)–C(1) 2.335(7), Y(1)–C(3) 2.512(4), Y(1)–C(4) 2.838(5), Y(1)–C(9) 2.534(3), C(1)–C(2) 1.506(4), C(3)–C(4) 1.517(7), Y(1)–C(4) 2.838(5), Y(1)–Al(1) 3.043(10), Al(1)–C(3) 2.099(4), Al(1)–C(9) 2.087(3); Y(1)–C(1)–C(2) 134.4(5), Y(1)– C(3)–C(4) 85.8(3), C(1)–Y(1)–C(9) 95.9(2), C(1)–Y(1)–C(3) 110.0(2), C(3)–Y(1)–C(9) 84.88(12), C(1)–Y(1)–Al(1) 100.50 (16). 2-Lu: Lu(1)–C(40) 2.479(3), Lu(1)–C(48) 2.308(8), Lu(1)–C(42) 2.485(4), Lu(1)–C(43) 2.805(4), C(48)–C(49) 1.522(3), C(42)– C(43) 1.534(6), Lu(1)–C(43) 2.805(4), Lu(1)–Al(1) 2.988(10), Al(1)–C(40) 2.088(4), Al(1)–C(42) 2.102(4); Lu(1)–C(48)–C(49) 133.3(6), Lu(1)–C(42)–C(43) 85.0(2), C(40)–Lu(1)–C(48) 95.6(3), C(42)–Lu(1)–C(48) 109.9(3), C(40)–Lu(1)–C(42) 86.45(12), C(48)–Lu(1)–Al(1) 99.49(18). | |
Interestingly, the unexpected and unprecedented bridged-ethyne heterobimetallic Y/Al complex {LY(μ2-η1-Et)2(AlEt)}2(μ4-η1:η1:η2:η2-CH-CH) (3-Y) was isolated when the treatment of the yttrium dialkyl complex with AlEt3 (3 equiv) in toluene at room temperature for 72 h was carried out. However, attempts to prepare the lutetium analogue by a similar synthetic process were unsuccessful, even at reaction temperatures up to 60 °C. Certainly, 2-Y could be converted to 3-Y slowly through further hydrogen abstraction reactions along with the release of ethane. The 1H, 13C{1H} and 13C DEPT-135 NMR spectral analyses of 3-Y in C6D6 are particularly informative. The one singlet signal at δ = 2.66 ppm for 1H (89.8 ppm for 13C{1H}) is observed in the Y-bonded ethyne ligand, integrating two hydrogens, in accordance with the magnetic equivalency of the μ4-η1:η1:η2:η2-CH-CH unit. This implies high molecular symmetry and unhindered rotation of the μ4-η1:η1:η2:η2-CHCH moiety in the solvent. Meanwhile, only one set of proton signals of all ethyl groups in the [Et3Al–C2H2–AlEt3]4− moiety is observed with a broad peak (0.33 ppm for CH2) and a triplet (1.40 ppm for CH3) with integral ratios of 12:18.
Red crystals of 3-Y were crystallized from a concentrated toluene solution layered with hexane, and the X-ray structure analysis reveals a dimeric structure (Fig. 3). The Y and Al atoms are capped by the μ4-η1:η1:η2:η2-CHCH moiety. The characteristic feature of 3-Y is an ethyne bridge that forms a perpendicular bisector of the Y–Y axis. This is slightly different from the osmium complex Os2(CO)8(μ2-η1:η1-C2H2) in which the ethyne ligand is tetra-σ bonded over a square face.20d The Y–C(μ4-CHCH) bond lengths, ranging from 2.371(3) to 2.432(3) Å, are available. Interesting, the C–C bond length of the ethyne-bridge (1.550(5) Å) is a longish single C–C bond. This may be attributed to the generated steric hindrance of two AlEt3 stabilization with a highly polarized Y–CHCH–Y unit, which ultimately leads to the long μ4-η1:η1:η2:η2-coordinated C–C bond. Until now, as far as we are aware, 3-Y represents the first example of a well-defined rare-earth ethyne complex.
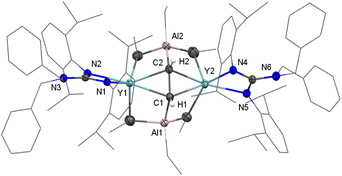 |
| Fig. 3 Molecular structure of 3-Y with thermal ellipsoids at 30% probability. All of the hydrogen atoms (except for H1 and H2) are omitted for clarity. Selected bond distances (Å): Y(1)–C(1) 2.432(3), Y(1)–C(2) 2.371(3), Y(2)–C(1) 2.377(3), Y(2)–C(2) 2.405(3), C(1)–C(2) 1.550(5), Al(1)–C(1) 1.976(3), Al(2)–C(2) 1.977(4); C(1)–Y(1)–C(2) 37.62(11), C(1)–C(2)–Y(1) 73.36(16), C(1)–C(2)–Al(2) 134.0(2), C(2)–C(1)–Al(1) 133.3(2). | |
Subsequently, when four equivalents of AlEt3 were used in the reaction with LY(CH2C6H4NMe2-o)2 in toluene at room temperature, unfortunately, the isolation of major products was unsuccessful. Unexpectedly, the mononuclear heterobimetallic ethylene complex LY(μ3-η1:η1:η2-C2H4)[(μ2-η1-Et)(AlEt2) (μ2-η1-Et)2(AlEt)] (4-Y, 81%) was obtained while the reaction mixture was treated at 50 °C for 24 h (Scheme 4). However, we just separated out 2-Lu as a major product when the reaction of the lutetium dialkyl complex with AlEt3 (4 equiv) was treated under the same conditions. To get more insights into the formation process of 4-Y, the same reaction was carried out at −10 °C for 3 h which gave a heterobimetallic Y/Al complex LY[(μ2-η1-Et)2(AlEt2)]2 (5-Y, 92%). In the NMR spectra of 5-Y, only one set of signals of the tetraethylaluminate moieties is observed in C6D6 at room temperature13d (Fig. S23–S24†), which is also similar to the [AlMe4]− ligands.7b,23 Crucially, 5-Y could be transformed into 4-Y in toluene at 50 °C for 24 h with the release of ethane through the β-hydrogen abstraction reaction25 (Fig. S25†). It is well established that 5-Y is a crucial intermediate in the formation of 4-Y. A broad singlet at δ = 0.81 ppm in the 1H NMR spectrum of 4-Y is assignable to the ethylene moiety of the type Y–CH2CH2–Y, integrating to four hydrogens, which is comparable to the ethylene resonance found for the lanthanum complex (AlEt4)La{(μ-Et)(AlEt2)}2(μ-C2H4)17b (1H NMR: 0.81 ppm in C6D6). However, these signals shifted markedly compared to the ethyne resonance found for 3-Y, verifying the differences in the core structural units. In the 13C{1H} NMR spectrum, the δ = 13.3 (JYC = 7 Hz) ppm is also observed as the carbon signal of the Y–CH2CH2–Y moiety. Compared to 2-Y, the chemical shift of the 89Y of 4-Y (326.4 ppm, Fig. S22†) toward the higher field might be attributed to the coordination of AlEt3 and increased electronegativity of the μ3-η1:η1:η2-C2H4 moiety.24
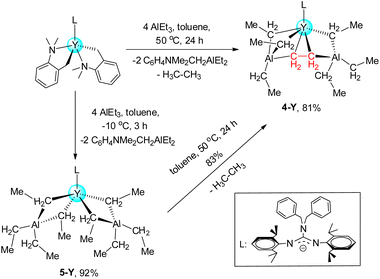 |
| Scheme 4 Synthesis of heterobimetallic rare-earth ethyl complex 4-Y and ethylene complex 5-Y. | |
4-Y was further characterized by X-ray diffraction analysis (Fig. 4). From structural parameters, the Y–C(μ2-C2H4) distances (2.475(9) and 2.689(9) Å) are slightly longer than in {(C5Me4SiMe2 NtBu)Sc(PMe3)}2(μ-C2H4) (2.320(9) and 2.357(9) Å) when the difference between metallic radii is considered. Besides, the C–C bond length of ethylene-bridged (1.533(13) Å) is longer that the bond length observed in {(C5Me4SiMe2NtBu)Sc(PMe3)}2(μ-η2:η2-C2H4) (1.433 (12) Å), and which is unlikely to the C
C distance in free ethylene (1.34 Å)26 while the length approaches a value typical of a carbon–carbon single bond, such as 1.522(2) Å in ethane.27 To our knowledge, 4-Y is the only well-defined non-Cp rare-earth ethylene complex. The molecular structure of 5-Y is also characterized by X-ray diffraction analysis (Fig. 5). The bridged ethyl units of [AlEt4]− only display the μ-η1-ethyl bonding to the yttrium center, similar to previous Ln/Al heterobimetallic ethyl complexes containing [AlEt4]− moieties.13a,c,e,g
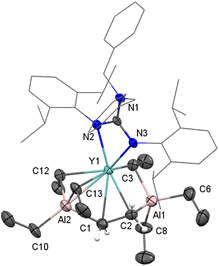 |
| Fig. 4 Molecular structure of 4-Y with thermal ellipsoids at 30% probability. All of the hydrogen atoms (except for H1 and H2) are omitted for clarity. Selected bond distances (Å) and angles (°): Y(1)–C(1) 2.689(9), Y(1)–C(2) 2.475(9), C(1)–C(2) 1.533(13), Al(1)– C(2) 2.047(10), Al(2)–C(1) 2.071(10); C(1)–Y(1)–C(2) 34.2(3), Y(1)– C(1)–C(2) 65.2(4), Y(1)–C(2)–C(1) 80.6(5), Y(1)–C(1)–Al(2) 70.3(3), Y(1)–C(2)–Al(1) 84.4(3). | |
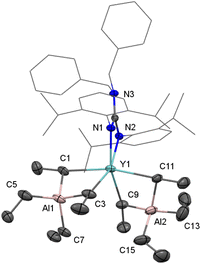 |
| Fig. 5 Molecular structure of complex 5-Y with thermal ellipsoids at 30% probability except for the 2,6-(iPr)2C6H3 groups and benzyl groups in the guanidinate ligand. All hydrogen atoms are omitted for clarity. Selected bond distances (Å) and angles (°): Y(1)–C(1) 2.619(4), Y(1)–C(3) 2.572(4), Y(1)–C(9) 2.564(3), Y(1)–C(11) 2.622(8), Y(1)–N(1) 2.336(2), Y(1)–N(2) 2.299(2), Al(1)–C(5) 2.008(5), Al(1)– C(7) 2.004(4), Al(2)–C(13) 1.956(6), Al(2)–C(15) 2.024(5); C(1)–Y(1)–C(3) 78.12(12), C(1)–Y(1)–C(9) 89.45(12), C(1)–Y(1)–C(11) 165.9(2), Y(1)–C(1)–Al(1) 85.17(13), Y(1)–C(9)–Al(2) 83.92(12). | |
DFT calculations
The formation of 1-Y, 2-Y, and 3-Y was investigated computationally at the DFT level (B3PW91 functional) including solvent and dispersion corrections (Fig. 6). The reaction begins by the electrophilic attack of the aluminum to the benzylic carbon, which occurs with almost no barrier (2.7 kcal mol−1) to form a stable intermediate (Int2). Int2 isomerizes in order to have an ethyl group in the bridging position between Y and Al. This isomerization allows the formation of Int3 with an energy cost of 14.3 kcal mol−1. This isomerization allows a kinetically facile Al–Et bond breaking (barrier of 0.6 kcal mol−1 from Int3, 14.9 kcal mol−1 from Int2). Following the intrinsic reaction coordinate, it yields an yttrium aminobenzyl/ethyl complex Int5, which further reacts in a similar fashion with a second triethylaluminium molecule. The same sequence of reaction as described previously allows the formation of a quite unstable monomeric guanidinato-yttrium diethyl complex (Int8), that can either dimerize to form 1-Y, which is thermodynamically favored by 69.1 kcal mol−1 or can further react with a third molecule of triethylaluminium to yield 2-Y, which is also thermodynamically favorable (−53.1 kcal mol−1).
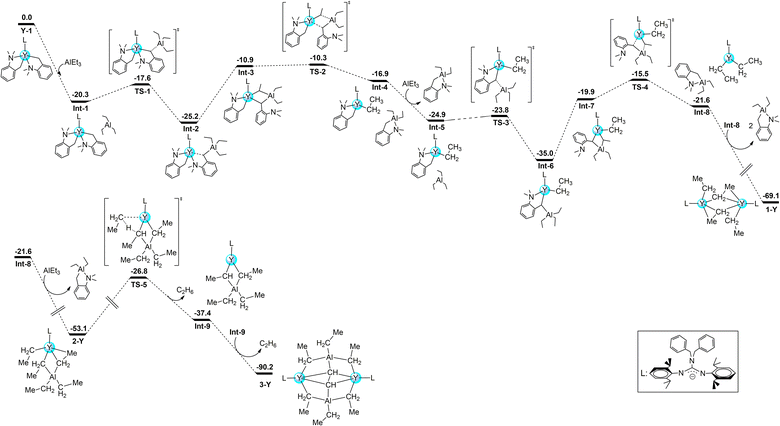 |
| Fig. 6 Computed enthalpy pathway at the DFT level for the formation of 1-Y, 2-Y, and 3-Y at room temperature. Energy is given in kcal mol−1. | |
Interestingly, 2-Y can undergo a C–H activation reaction with a barrier of 26.3 kcal mol−1 to yield Int9, which dimerizes to form the most stable complex 3-Y accompanying C–C bond cleavage (Me–CH) and new C–C bond formation (CH–CH) (−90.2 kcal mol−1). To gain more insights into the bonding properties of the yttrium ethyne complex, DFT calculations on 3-Y were carried out. Scrutinizing the molecular orbitals indicates that the HOMO-7 and HOMO-4 are Y–C σ bonding interactions (Fig. 7). The bonding situation is further confirmed by the Natural bonding orbital (NBO) analysis and the Wiberg bond indexes (WBIs). The geometry optimization (B3PW91) of 3-Y revealed structural parameters in agreement with their experimental counterparts: the C1–C2 (1.531 Å) distance resembled the observed X-ray distances of 1.550(5) Å. Indeed, the Wiberg bond index (WBI) is 1.02 for the C1–C2, in line with the lack of π interaction in the ethyne unit. The Y–C WBI values are 0.36, 0.39, 0.41, and 0.42 respectively, further corroborating the single bond character of the C1–C2 interaction. The polarized nature of the bonds is further demonstrated and the charges carried by the ethyne carbon are −1.40 and −1.41, whereas those of the ethyne hydrogen are +0.29 and +0.29.
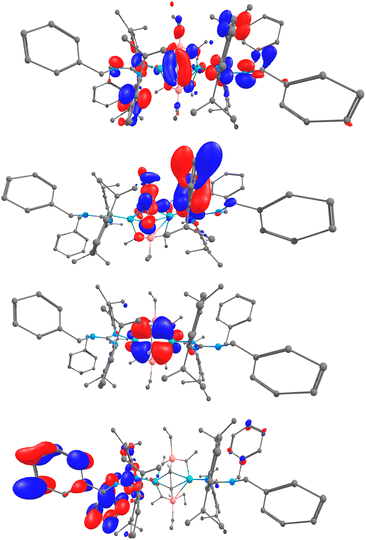 |
| Fig. 7 DFT computed MOs for the yttrium ethyne complex 3-Y: (a) HOMO-7, (b) HOMO-4, (c) HOMO, (d) LUMO. Atom color code: green, yttrium; blue, nitrogen; gray, carbon; and white, hydrogen. | |
Conclusions
In summary, we have successfully isolated and structurally characterized guanidinato-stabilized homo-metallic and heterobimetallic rare-earth ethyl complexes through transmetalation reactions. To our excitement, not only the first example of a non-Cp rare-earth ethylene complex through the β-H abstraction was isolated successfully, but also a unique well-defined rare-earth ethyne complex was obtained by the α-H abstraction and C–C σ bond metathesis process based on the mechanism studies by DFT calculations. Studies on the reaction chemistry of these hitherto unexplored μ2-CH2CH2 and μ4-CHCH moieties are underway.
Data availability
The data that support the findings of this study are available in the ESI† of this article.
Author contributions
Mr Wen Jiang carried out the experiments, crystal analyses and manuscript writing. Dr Thayalan Rajeshkumar and Prof. Laurent Maron are responsible for the DFT calculation. Dr Yuejian Lin helped Wen Jiang in crystal analyses. Miss. Guo Mengyue assisted Mr Jiangwen to synthesize the raw materials. Prof. Zhang Lixin designed the experiments and revised this paper.
Conflicts of interest
The authors declare no conflict of interest.
Acknowledgements
This work was supported by the National Natural Science Foundation of China (grant nos 21871052). We are grateful to Prof. Xigeng Zhou and Prof Huadong Wang at Fudan University for helpful discussion. The authors acknowledge the HPCs CALcul en Midi-Pyrénées (CALMIP-EOS grant 1415).
Notes and references
-
(a) M. Zimmermann and R. Anwander, Chem. Rev., 2010, 110, 6194–6259 CrossRef CAS PubMed;
(b) F. T. Edelmann, Chem. Soc. Rev., 2012, 41, 7657–7672 RSC;
(c) A. A. Trifonov and D. M. Lyubov, Coord. Chem. Rev., 2017, 340, 10–61 CrossRef CAS.
-
(a) Z. Hou and Y. Wakatsuki, Coord. Chem. Rev., 2002, 231, 1–22 CrossRef CAS;
(b) J. Gromada, J. F. Carpentier and A. Mortreux, Coord. Chem. Rev., 2004, 248, 397–410 CrossRef CAS;
(c) M. Nishiura and Z. Hou, Nat. Chem., 2010, 2, 257–268 CrossRef CAS PubMed;
(d) M. Nishiura, F. Guo and Z. Hou, Acc. Chem. Res., 2015, 48, 2209–2220 CrossRef CAS PubMed.
-
(a) S. Hong and T. J. Marks, Acc. Chem. Res., 2004, 37, 673–686 CrossRef CAS PubMed;
(b) T. E. Muller, K. C. Hultzsch, M. Yus, F. Foubelo and M. Tada, Chem. Rev., 2008, 108, 3795–3892 CrossRef PubMed;
(c) D. M. Lyubov and A. A. Trifonov, Inorg. Chem. Front., 2021, 8, 2965–2986 RSC;
(d) F. Ortu, Chem. Rev., 2022, 122, 6040–6116 CrossRef CAS PubMed.
-
(a) K. Aparna, M. Ferguson and R. G. Cavell, J. Am. Chem. Soc., 2000, 122, 726–727 CrossRef CAS;
(b) D. P. Mills, L. Soutar, W. Lewis, A. J. Blake and S. T. Liddle, J. Am. Chem. Soc., 2010, 132, 14379–14381 CrossRef CAS PubMed;
(c) M. Fustier, X. F. Le Goff, P. Le Floch and N. Mézailles, J. Am. Chem. Soc., 2010, 132, 13108–13110 CrossRef CAS PubMed;
(d) S. T. Liddle, D. P. Mills and A. J. Wooles, Chem. Soc. Rev., 2011, 40, 2164–2176 RSC;
(e) M. Fustier, X. F. Le Goff, M. Lutz, J. C. Slootweg and N. Mézailles, Organometallics, 2015, 34, 63–72 CrossRef CAS.
- H. M. Dietrich, K. W. Törnroos and R. Anwander, J. Am. Chem. Soc., 2006, 128, 9298–9299 CrossRef CAS PubMed.
-
(a) M. Zimmermann, D. Rauschmaier, K. Eichele, K. W. Törnroos and R. Anwander, Chem. Commun., 2010, 46, 5346–5348 RSC;
(b) J. Hong, L. Zhang, X. Yu, M. Li, Z. Zhang, P. Zheng, M. Nishiura, Z. Hou and X. Zhou, Chem.–Eur. J., 2011, 17, 2130–2137 CrossRef CAS PubMed;
(c) J. Hong, L. Zhang, K. Wang, Y. Zhang, L. Weng and X. Zhou, Chem.–Eur. J., 2013, 19, 7865–7873 CrossRef CAS PubMed;
(d) K. Wang, G. Luo, J. Hong, X. Zhou, H. Weng, Y. Luo and L. Zhang, Angew. Chem., Int. Ed., 2014, 53, 1053–1056 CrossRef CAS PubMed;
(e) J. Hong, Z. Li, Z. Chen, L. Weng, X. Zhou and L. Zhang, Dalton Trans., 2016, 45, 6641–6649 RSC;
(f) H. Tian, J. Hong, K. Wang, I. Rosal, L. Maron, X. Zhou and L. Zhang, J. Am. Chem. Soc., 2018, 140, 102–105 CrossRef CAS PubMed;
(g) J. Hong, H. Tian, L. Zhang, X. Zhou, I. del Rosal, L. Weng and L. Maron, Angew. Chem., Int. Ed., 2018, 57, 1062–1067 CrossRef CAS PubMed;
(h) C. O. Hollfelder, L. N. Jende, H. M. Dietrich, K. Eichele, C. Maichle-Mössmer and R. Anwander, Chem.–Eur. J., 2019, 25, 7298–7302 CrossRef CAS PubMed;
(i) C. O. Hollfelder, M. Zimmermann, C. Spiridopoulos, D. Werner, K. W. Törnroos, C. Maichle-Mössmer and R. Anwander, Molecules, 2019, 24, 3703–3730 CrossRef CAS PubMed;
(j) D. A. Buschmann, L. Schumacher and R. Anwander, Chem. Commun., 2022, 58, 9132–9135 RSC.
-
(a) M. Zimmermann, J. Takats, G. Kiel, K. W. Törnroos and R. Anwander, Chem. Commun., 2008, 612–614 RSC;
(b) J. Scott, H. J. Fan, B. F. Wicker, A. R. Fout, M. H. Baik and D. J. Mindiola, J. Am. Chem. Soc., 2008, 130, 14438–14439 CrossRef CAS PubMed;
(c) R. Litlabø, M. Zimmermann, K. Saliu, J. Takats, K. W. Törnroos and R. Anwander, Angew. Chem., Int. Ed., 2008, 47, 9560–9564 CrossRef PubMed;
(d) A. Venugopal, I. Kamps, D. Bojer, R. J. F. Berger, A. Mix, A. Willner, B. Neumann, H.-G. Stammler and N. W. Mitzel, Dalton Trans., 2009, 5755–5765 RSC;
(e) I. Korobkov and S. Gambarotta, Organometallics, 2009, 28, 5560–5567 CrossRef CAS;
(f) W. Huang, C. T. Carver and P. L. Diaconescu, Inorg. Chem., 2011, 50, 978–984 CrossRef CAS PubMed;
(g) D. Barisic, D. Diether, C. Maichle-Mössmer and R. Anwander, J. Am. Chem. Soc., 2019, 141, 13931–13940 CrossRef CAS PubMed;
(h) P. Zatsepin, E. Lee, J. Gu, M. R. Gau, P. J. Carroll, M. Baik and D. J. Mindiola, J. Am. Chem. Soc., 2020, 142, 10143–10152 CrossRef CAS PubMed;
(i) T. E. Rieser, R. Thim-Spöring, D. Schädle, P. Sirsch, R. Litlabø, K. W. Törnroos, C. Maichle-Mössmer and R. Anwander, J. Am. Chem. Soc., 2022, 144, 4102–4113 CrossRef CAS PubMed.
-
(a) W. Zhang, Z. Wang, M. Nishiura, Z. Xi and Z. Hou, J. Am. Chem. Soc., 2011, 133, 5712–5715 CrossRef CAS PubMed;
(b) T. Li, M. Nishiura, J. Cheng, Y. Li and Z. Hou, Chem.–Eur. J., 2012, 18, 15079–15085 CrossRef CAS PubMed.
-
(a) S. Li, M. Wang, B. Liu, L. Li, J. Cheng, C. Wu, D. Liu, J. Liu and D. Cui, Chem.–Eur. J., 2014, 20, 15493–15498 CrossRef CAS PubMed;
(b) T. Shima, T. Yanagi and Z. Hou, New J. Chem., 2015, 39, 7608–7616 RSC;
(c) J. Zhou, T. Li, L. Maron, X. Leng and Y. Chen, Organometallics, 2015, 34, 470–476 CrossRef CAS;
(d) T. Li, G. Zhang, J. Guo, S. Wang, X. Leng and Y. Chen, Organometallics, 2016, 35, 1565–1572 CrossRef CAS;
(e) F. Yan, S. Li, L. Li, W. Zhang, D. Cui, M. Wang and Y. Dou, Eur. J. Inorg. Chem., 2019, 17, 2277–2283 CrossRef.
-
(a) D. J. Mindiola and J. Scott, Nat. Chem., 2011, 3, 15–17 CrossRef CAS PubMed;
(b) C. Wang, J. Zhou, X. Zhao, L. Maron, X. Leng and Y. Chen, Chem.–Eur. J., 2016, 22, 1258–1261 CrossRef CAS PubMed;
(c) W. Mao, L. Xiang, L. Maron, X. Leng and Y. Chen, J. Am. Chem. Soc., 2017, 139, 17759–17762 CrossRef CAS PubMed;
(d) W. Mao, L. Xiang, C. A. Lamsfus, L. Maron, X. Leng and Y. Chen, J. Am. Chem. Soc., 2017, 139, 1081–1084 CrossRef CAS PubMed;
(e) W. Mao, L. Xiang, C. A. Lamsfus, L. Maron, X. Leng and Y. Chen, Chin. J. Chem., 2018, 36, 904–908 CrossRef CAS;
(f) C. Wang, L. Xiang, Y. Yang, J. Fang, L. Maron, X. Leng and Y. Chen, Chem.–Eur. J., 2018, 24, 5637–5643 CrossRef CAS PubMed;
(g) C. Wang, W. Mao, L. Xiang, Y. Yang, J. Fang, L. Maron, X. Leng and Y. Chen, Chem.–Eur. J., 2018, 24, 13903–13917 CrossRef CAS PubMed;
(h) W. Mao, Y. Wang, L. Xiang, Q. Peng, X. Leng and Y. Chen, Chem.–Eur. J., 2019, 25, 10304–10308 CrossRef CAS PubMed.
-
(a) W. Ma, C. Yu, Y. Chi, T. Chen, L. Wang, J. Yin, B. Wei, L. Xu, W. Zhang and Z. Xi, Chem. Sci., 2017, 8, 6852–6856 RSC;
(b) Y. Zheng, C. Cao, W. Ma, T. Chen, B. Wu, C. Yu, Z. Huang, J. Yin, H. Hu, J. Li, W. Zhang and Z. Xi, J. Am. Chem. Soc., 2020, 142, 10705–10714 CrossRef CAS PubMed.
-
(a) H. M. Dietrich, H. Grove, K. W. Törnroos and R. Anwander, J. Am. Chem. Soc., 2006, 128, 1458–1459 CrossRef CAS PubMed;
(b) L. C. H. Gerber, E. Le Roux, K. W. Törnroos and R. Anwander, Chem.–Eur. J., 2008, 14, 9555–9564 CrossRef CAS PubMed;
(c) D. Bojer, A. Venugopal, B. Neumann, H.-G. Stammler and N. W. Mitzel, Angew. Chem., Int. Ed., 2010, 49, 2611–2614 CrossRef CAS PubMed;
(d) D. Bojer, B. Neumann, H.-G. Stammler and N. W. Mitzel, Chem.–Eur. J., 2011, 17, 6239–6247 CrossRef CAS PubMed;
(e) D. Bojer, B. Neumann, H.-G. Stammler and N. W. Mitzel, Eur. J. Inorg. Chem., 2011, 3791–3796 CrossRef CAS;
(f) P. Deng, X. Shi, X. Gong and J. Cheng, Chem. Commun., 2021, 57, 6436–6439 RSC;
(g) W. Jiang, F. Kong, I. Rosal, M. Li, K. Wang, L. Maron and L. Zhang, Chem. Sci., 2023, 14, 9154–9160 RSC.
-
(a) W. J. Evans, L. R. Chamberlain and J. W. Ziller, J. Am. Chem. Soc., 1987, 109, 7209–7211 CrossRef CAS;
(b) D. Stern, M. Sabat and T. J. Marks, J. Am. Chem. Soc., 1990, 112, 9558–9575 CrossRef CAS;
(c) M. G. Klimpel, J. Eppinger, P. Sirsch, W. Scherer and R. Anwander, Organometallics, 2002, 21, 4021–4023 CrossRef CAS;
(d) A. Fischbach, M. G. Klimpel, M. Widenmeyer, E. Herdtweck, W. Scherer and R. Anwander, Angew. Chem., Int. Ed., 2004, 43, 2234–2239 CrossRef CAS PubMed;
(e) M. G. Schrems, H. M. Dietrich, K. W. Törnroos and R. Anwander, Chem. Commun., 2005, 5922–5924 RSC;
(f) A. Fischbach, F. Perdih, E. Herdtweck and R. Anwander, Organometallics, 2006, 25, 1626–1642 CrossRef CAS;
(g) H. Sommerfeldt, C. Meermann, M. G. Schrems, K. W. Törnroos, N. Frøystein, R. J. Miller, E. Scheidt, W. Scherer and R. Anwander, Dalton Trans., 2008, 1899–1907 RSC.
-
(a) M. D. Fryzuk, G. Giesbrecht and S. J. Rettig, Organometallics, 1996, 15, 3329–3336 CrossRef CAS;
(b) T. I. Gountchev and T. D. Tilley, Organometallics, 1999, 18, 2896–2905 CrossRef CAS;
(c) P. G. Hayes, W. E. Piers, L. W. M. Lee, L. K. Knight, M. Parvez, M. R. J. Elsegood and W. Clegg, Organometallics, 2001, 20, 2533–2544 CrossRef CAS;
(d) M. R. MacDonald, R. R. Langeslay, J. W. Ziller and W. J. Evans, J. Am. Chem. Soc., 2015, 137, 14716–14725 CrossRef CAS PubMed;
(e) D. B. Culver, W. Huynh, H. Tafazolian, T. C. Ong and M. P. Conley, Angew. Chem., Int. Ed., 2018, 57, 9520–9523 CrossRef CAS PubMed.
- H. Yamamoto, H. Yasuda, K. Yokota, A. Nakamura, Y. Kai and N. Kasai, Chem. Lett., 1988, 1963–1966 CrossRef CAS.
-
(a) W. J. Evans, T. M. Champagne, D. G. Giarikos and J. W. Ziller, Organometallics, 2005, 24, 570–579 CrossRef CAS;
(b) W. J. Evans, T. M. Champagne and J. W. Ziller, Organometallics, 2005, 24, 4882–4885 CrossRef CAS.
-
(a) P. J. Shapiro, W. P. Schaefer, J. A. Labinger, J. E. Bercaw and W. D. Cotter, J. Am. Chem. Soc., 1994, 116, 4623–4640 CrossRef CAS;
(b) H. M. Dietrich, K. W. Törnroos and R. Anwander, Angew. Chem., Int. Ed., 2011, 50, 12089–12093 CrossRef CAS PubMed.
-
(a) W. Kaminsky, J. Kopf and G. Thirase, Adv. Cycloaddit., 1974, 1531–1533 CAS;
(b) W. Kaminsky and H. Sinn, Adv. Cycloaddit., 1975, 424–437 CAS;
(c) W. Kaminsky and H. Vollmer, Adv. Cycloaddit., 1975, 438–448 CAS;
(d) W. Kaminsky, J. Kopf, H. Sinn and H. J. Vollmer, Angew. Chem., Int. Ed. Engl., 1976, 15, 629–630 CrossRef.
-
(a) L. H. Shultz, D. J. Tempel and M. Brookhart, J. Am. Chem. Soc., 2001, 123, 11539–11555 CrossRef CAS PubMed;
(b) X. Dai and T. H. Warren, Chem. Commun., 2001, 1998–1999 RSC;
(c) H. V. Rasika Dias, M. Fianchini, T. R. Cundari and C. F. Campana, Angew. Chem., Int. Ed., 2008, 47, 556–559 CrossRef PubMed;
(d) J. Wolf, K. Thommes, O. Briel, R. Scopelliti and K. Severin, Organometallics, 2008, 27, 4464–4474 CrossRef CAS;
(e) H. V. Rasika Dias and J. Wu, Eur. J. Inorg. Chem., 2008, 509–522 CrossRef;
(f) Y. Segawa, M. Yamashita and K. Nozaki, Organometallics, 2009, 28, 6234–6242 CrossRef CAS;
(g) P. Ebrahimpour, M. F. Haddow and D. F. Wass, Inorg. Chem., 2013, 52, 3765–3771 CrossRef CAS PubMed;
(h) M. Navarro, M. G. Alférez, M. de Sousa, J. Miranda-Pizarro and J. Campos, ACS Catal., 2022, 12, 4227–4241 CrossRef CAS PubMed;
(i) M. Maekawa, T. Hayashi, K. Sugimoto, T. Okubo and T. Kuroda-Sowa, Dalton Trans., 2023, 52, 14941–14948 RSC.
-
(a) G. Gervasio, R. Rossetti and P. L. Stanghellini, Organometallics, 1985, 4, 1612–1619 CrossRef CAS;
(b) S. F. Parker, P. H. Dallin, B. T. Keiller, C. E. Ansonb and U. A. Jayasooriya, Phys. Chem. Chem. Phys., 1999, 1, 2589–2592 RSC;
(c) G. Kiel, Z. Zhang, J. Takats and R. B. Jordan, Organometallics, 2000, 19, 2766–2776 CrossRef CAS;
(d) C. E. Anson, N. Sheppard, R. Pearman, J. R. Moss, P. Stoßel, S. Koch and J. R. Norton, Phys. Chem. Chem. Phys., 2004, 6, 1070–1076 RSC;
(e) J. A. Platts, G. J. S. Evans, M. P. Coogan and J. Overgaard, Inorg. Chem., 2007, 46, 6291–6298 CrossRef CAS PubMed.
-
(a) E. Durgun, S. Ciraci, W. Zhou and T. Yildirim, Phys. Rev. Lett., 2006, 97, 226102 CrossRef CAS PubMed;
(b) N. Wadnerkar, V. Kalamse and A. Chaudhari, RSC Adv., 2012, 2, 8497–8501 RSC.
- F. Kong, M. Li, X. Zhou and L. Zhang, RSC Adv., 2017, 7, 29752–29761 RSC.
-
(a) N. Dettenrieder, H. M. Dietrich, C. Schädle, C. Maichle-Mössmer, K. W. Törnroos and R. Anwander, Angew. Chem., Int. Ed., 2012, 51, 4461–4465 CrossRef CAS PubMed;
(b) W. Rong, M. Wang, S. Li, J. Cheng, D. Liu and D. Cui, Organometallics, 2018, 37, 971–978 CrossRef CAS.
-
(a) C. J. Schaverien, Organometallics, 1994, 13, 69–82 CrossRef CAS;
(b) R. E. White and T. P. Hanusa, Organometallics, 2006, 25, 5621–5630 CrossRef CAS;
(c) C. Schädle, A. Fischbach, E. Herdtweck, K. W. Törnroos and R. Anwander, Chem.–Eur. J., 2013, 19, 16334–16341 CrossRef PubMed;
(d) L. Lätsch, E. Lam and C. Copéret, Chem. Sci., 2020, 11, 6724–6735 RSC.
- P. Bertus, Organometallics, 2019, 38, 4171–4182 CrossRef CAS.
- F. A. Cotton, E. V. Dikarev, M. A. Petrukhina and R. E. Taylor, J. Am. Chem. Soc., 2001, 123, 5831–5832 CrossRef CAS PubMed.
-
M. D. Harmony, Chapter 1 Molecular Structure Determination from Spectroscopic Data Using Scaled Moments of Inertia, in Equilibrium Structural Parameters, ed. J. R. Durig, Elsevier, Oxford, U.K., Vibra-tional Spectra and Structure, 1999, vol. 24, pp. 1–83 Search PubMed.
Footnote |
† Electronic supplementary information (ESI) available: Full experimental procedures, spectra, and analytical data. CCDC 2259604–2259609. For ESI and crystallographic data in CIF or other electronic format see DOI: https://doi.org/10.1039/d3sc06599e |
|
This journal is © The Royal Society of Chemistry 2024 |
Click here to see how this site uses Cookies. View our privacy policy here.