DOI:
10.1039/D4SC00649F
(Edge Article)
Chem. Sci., 2024,
15, 6012-6021
Cobalt- or rhodium-catalyzed synthesis of 1,2-dihydrophosphete oxides via C–H activation and formal phosphoryl migration†
Received
28th January 2024
, Accepted 11th March 2024
First published on 14th March 2024
Abstract
A highly stereo- and chemoselective intermolecular coupling of diverse heterocycles with dialkynylphosphine oxides has been realized via cobalt/rhodium-catalyzed C–H bond activation. This protocol provides an efficient synthetic entry to functionalized 1,2-dihydrophosphete oxides in excellent yields via the merger of C–H bond activation and formal 1,2-migration of the phosphoryl group. Compared with traditional methods of synthesis of 1,2-dihydrophosphetes that predominantly relied on stoichiometric metal reagents, this catalytic system features high efficiency, a relatively short reaction time, atom-economy, and operational simplicity. Photophysical properties of selected 1,2-dihydrophosphete oxides are also disclosed.
Introduction
Organophosphorus molecules are an important class of compounds which are not only widely utilized as ligands or organocatalysts for diverse transformations but also function as drugs and bioactive molecules.1 The development of efficient methods for the synthesis of functionalized organophosphorus compounds has attracted continuous attention.2 In particular, four-membered phosphorus compounds have found vast applications in the studies of catalysis, medicinal chemistry, and materials science (Fig. 1).3 For instance, compound I is known as a new organic catalyst that enables the reductive C–N cross-coupling of functionalized nitroalkanes with arylboronic acids, and compound II exhibits unique photophysical properties, while compound III is employed as a useful chiral bidentate ligand. However, efficient methods to access four-membered phosphacycles, especially 1,2-dihydrophosphete (oxides), only remain sporadic. In 1985, Mathey and co-workers reported synthesis of metal complexes of 2-keto-1,2-dihydrophosphetes via CO insertion into a P–C bond of phosphirene-chromium, -molybdenum, and -tungsten pentacarbonyl complexes as a result of ring expansion.4 After that, they further disclosed coupling of electron-poor phospha-alkene P–W(CO)5 complexes with electron-rich alkynes via [2 + 2] cycloadditions (Scheme 1a1).5 In 1989, Knobler reported the reaction of diphenyltitanacyclobutene with phenyldichlorophosphine, allowing the first isolation of free 1,2-dihydrophosphetes (Scheme 1a2).6 In 2021, Pietschnig and co-workers established a transition-metal free annulation reaction between 1,3-diynes and phosphanides (Scheme 1a3).7 Recently, Gates accomplished cyclization of 1-phosphabutadiene and isolated Au- and Pd-stabilized 1,2-dihydrophosphete complexes (Scheme 1a4).8
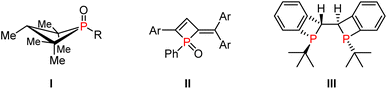 |
| Fig. 1 Selected examples of four-membered phosphacycles. | |
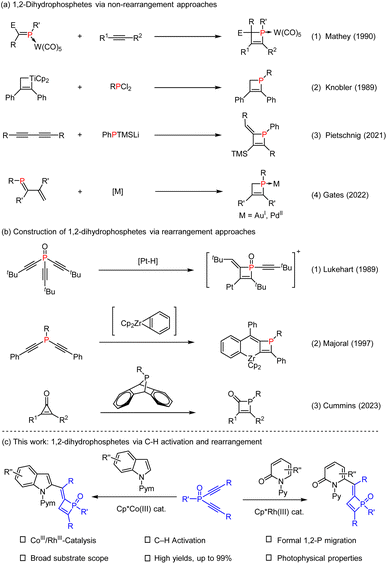 |
| Scheme 1 Synthesis of 1,2-dihydrophosphetes. | |
Rearrangement reactions provide straightforward and efficient access to complex organic frameworks via structural reorganizations.9 In 1989, Lukehart developed stoichiometric reactions of trialkynylphosphine oxide and platinum hydride that involve Pt–H addition and rearrangement reactions (Scheme 1b1).10 In 1997, Majoral reported synthesis of metallacycle-supported 1,2-dihydrophosphete via coupling of bis(alkynyl)phosphines with zirconocene-benzynes (Scheme 1b2).11 Very recently, Cummins realized phosphinidene transfer to cyclopropenones for synthesis of phosphet-2-ones(Scheme 1b3).12 Despite these strategies, the majority of systems require the employment of stoichiometric amounts of metal reagents such as zirconium, platinum, titanium, and tungsten complexes. In addition, the reaction scope is also limited due to compatibility issues. Therefore, efficient synthesis of 1,2-dihydrophosphetes from readily available reagents still awaits further development.
We reasoned that 1,2-dihydrophosphetes can be accessed via catalytic manipulation of the triple bond in dialkynylphosphine (oxides). Indeed, metal-catalyzed coupling of dialkynylphosphine oxides/sulfides delivered various powerful strategies to create complex phosphacycles.13 The C
C bond in the dialkynylphosphine is expected to readily participate as a special π-bond in C–H activation reactions, and the resulting alkenyl phosphine intermediate may also undergo rearrangement to give complex structures. While significant achievements have been made in C–H bond activation catalyzed by transition metals such as Pd, Rh, Ru, Ir, Mn, and Co,14 P-containing substrates are generally less explored either as the arene or the coupling reagent.15 Consequently, catalytic construction of four-membered phosphacycles remains a formidable challenge. By taking advantage of ready availability of arenes, our objective was to synthesize 1,2-dihydrophosphetes via integration of C–H bond activation and migration of the P atom in the reactive intermediate. Herein, we report the development of cobaltIII or rhodiumIII-catalyzed C–H activation of diverse classes of heteroarenes and coupling with dialkynylphosphine oxides, which affords a series of rare 1,2-dihydrophosphete oxides in high yields (Scheme 1c). The mechanism of this reaction has also been briefly explored.
Results and discussion
We commenced our studies with optimization of reaction parameters of the coupling of 1-(pyrimidin-2-yl)-1H-indole 1a and methylbis(phenylethynyl)phosphine oxide 2a (Table 1). The reaction occurred at 100 °C in the presence of a Cp*Co(CO)I2/AgSbF6 catalyst in DCE for 3 h, and the desired four membered PV
O product (3a) was isolated in 95% yield. It was found that Cp*Co(CO)I2 showed superiority to other catalysts such as [Cp*RhCl2]2 and [Cp*Co(MeCN)3](BF4)2 (Table 1, entries 1–3). The screening of solvents showed that TFE was the best choice (Table 1, entries 4–8), and a nearly quantitative yield was realized when the reaction was performed at 100 °C (entry 8). In addition, the reaction efficiency was slightly affected when the reaction temperature was lowered from 100 °C to 60 °C (entries 8–12).
Table 1 Optimization studiesa
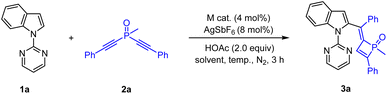
|
Entry |
Catalyst |
Solvent |
Temp. (°C) |
Yieldb (%) |
The reactions were carried out with 1a (0.13 mmol), 2a (0.10 mmol), M cat. (4 mol%), AgSbF6 (8 mol%) and HOAc (2.0 equiv.) in a solvent (1.0 mL) under N2 for 3 h.
Isolated yields.
AgSbF6 (16 mol%) was used.
No AgSbF6 was used.
|
1 |
[Cp*RhCl2]2c |
DCE |
100 |
78 |
2 |
[Cp*Co(MeCN)3](BF4)2 |
DCE |
100 |
93d |
3 |
Cp*Co(CO)I2 |
DCE |
100 |
95 |
4 |
Cp*Co(CO)I2 |
MeCN |
100 |
43 |
5 |
Cp*Co(CO)I2 |
THF |
100 |
Trace |
6 |
Cp*Co(CO)I2 |
EA |
100 |
35 |
7 |
Cp*Co(CO)I2 |
Dioxane |
100 |
40 |
8 |
Cp*Co(CO)I2 |
TFE |
100 |
98 |
9 |
Cp*Co(CO)I2 |
TFE |
90 |
96 |
10 |
Cp*Co(CO)I2 |
TFE |
80 |
91 |
11 |
Cp*Co(CO)I2 |
TFE |
70 |
90 |
12 |
Cp*Co(CO)I2 |
TFE |
60 |
93 |
With the optimized conditions established, we next evaluated the generality of this transformation (Scheme 2). Indoles with a variety of functionalities at the 4-position such as methyl, methoxy, halogens, ester group and formyl reacted smoothly to give the corresponding products 3a–3g in excellent yields. Besides, other functional groups such as electron-donating (Me, OBn) and -withdrawing (CO2Me, CHO and CN) and halogen groups (F, Cl and Br) at the C-5 position of the indoles were all generally tolerated, and the corresponding products (3h–3o) were isolated in high yields. Indoles bearing a 6-substituent underwent rapid coupling with 2a in good to excellent chemical yields (up to 99% yield, 3p–3v). The structure of 3r was confirmed by X-ray crystallographic analysis.16 7-Substituted indoles were also viable, affording the desired coupling products in good efficiency (3w–3y). Moreover, a pyrrole substrate was also amenable to this transformation, delivering the desired product 3z in 88% yield.
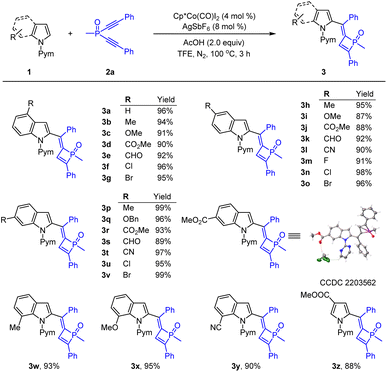 |
| Scheme 2 Substrate scope of indoles by cobalt-catalysis. Conditions: indoles 1 (0.26 mmol), dialkynylphosphine oxide 2a (0.20 mmol, 1.0 equiv.), Cp*Co(CO)I2 (4.0 mol%), AgSbF6 (8 mol%), HOAc (2.0 equiv.) in 2.0 mL of TFE under N2 at 100 °C for 3 h. Isolated yields. | |
The scope of the dialkynylphosphine oxides was next investigated (Scheme 3). Examination of the para- and meta-substituents in the benzene ring of the diyne terminus revealed that both electron-withdrawing and -donating groups were tolerated, providing products 4a–4f in 88–97% yields. The reaction worked well when a 1-naphthyl-substituted substrate was used, delivering the product 4g in 80% yield. Besides, dialkynylphosphine oxides containing heteroaryl groups in the alkyne unit were also applicable, and 2- and 3-thienyl groups produced the desired products 4h and 4i in 94% and 85% yield, respectively. Gratifyingly, alkenyl-substituted substrates afforded satisfactory results as in the isolation of product 4j in 88% yield. Cyclic and linear alkyl substituents were all tolerated under the reaction conditions with corresponding 4k–4m being isolated in 72–81% yields. Of note, changing the P-methyl group diyne to a P-phenyl one led to the desired product 4n in high isolated yield. To our disappointment, replacing the methyl group on the phosphorus center with a methoxyl or tert-butyl group failed to deliver any desired product.
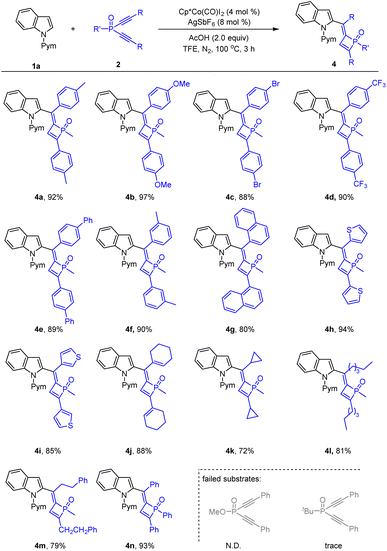 |
| Scheme 3 Substrate scope of dialkynylphosphine oxides by cobalt catalysis. Conditions: indoles 1a (0.26 mmol), dialkynylphosphine oxides 2 (0.20 mmol, 1.0 equiv.), Cp*Co(CO)I2 (4.0 mol%), AgSbF6 (8 mol%), and HOAc (2.0 equiv.) in TFE (2 mL) under N2 at 100 °C for 3 h. Isolated yields. | |
To further expand the scope of arenes, 2-pyridones were then examined. The coupling of 2-pyridone 5a and dialkynylphosphine oxide 2a only gave poor efficiency when using the same Cp*Co(Co)I2 catalyst. To our delight, when catalyzed by [Cp*RhCl2]2/AgSbF6 in DCE, the desired product was isolated in 92% yield (see the ESI† for details). The scope of the 2-pyridone substrate was then explored using the dialkynylphosphine oxide 2a as a coupling partner (Scheme 4). Thus, 2-pyridones bearing a series of substituents such as methyl, methoxyl, benzyloxyl, halogen, cyano and trifluoromethyl at the C3- or C4-positions were all compatible in this reaction, delivering the corresponding products in consistently high yields (6b–6l, 78–93% yield). 6-Bromo-isoquinolinone was tolerated under the reaction conditions and the coupling afforded the corresponding product 6m in 85% yield, which was confirmed by X-ray crystallography studies.17 2-Pyridone bearing a 5-methyl-N-pyridinyl group reacted smoothly to give product 6n in 90% yield. Regarding the dialkynylphosphine oxides, our results indicated that a series of phenyl, naphthyl, heteroaryl, and alkyl groups in the alkyne were all tolerated, furnishing the corresponding 1,2-dihydrophosphete oxides in good to high yields (6o–6z, 68–90%). The tolerance of bulky tBu groups in 6y may provide mechanistic insights (vide infra).
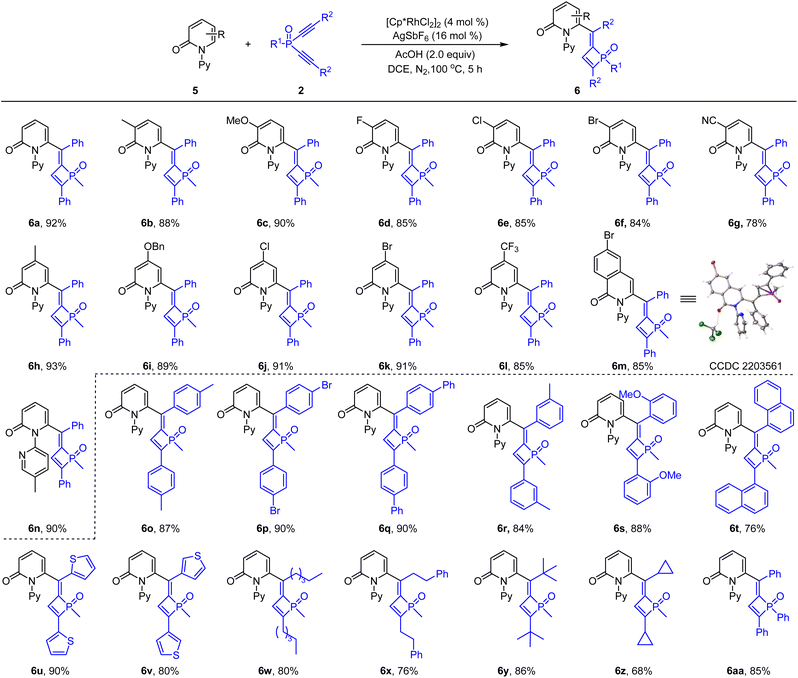 |
| Scheme 4 Scope of 2-pyridones and dialkynylphosphine oxides by rhodium catalysis. Conditions: 2-pyridones 5 (0.26 mmol), dialkynylphosphine oxides 2 (0.20 mmol, 1.0 equiv.), [Cp*RhCl2]2 (4.0 mol%), AgSbF6 (16 mol%), HOAc (2.0 equiv.) in 2.0 mL of DCE under N2 at 100 °C for 5 h. Isolated yields. | |
The photophysical properties of six products were briefly investigated (Fig. 2 and Table 2). Compounds 3a–4h displayed an intense absorption band in the UV/Vis region centered at 370 nm, attributed to the π–π* transitions of the extended π-conjugated system. Besides, these derivatives exhibited blue emissions. The fluorescent emission maxima appeared in the range of 470–504 nm (Table 2). These results may indicate their potential for applications in photoelectronics.
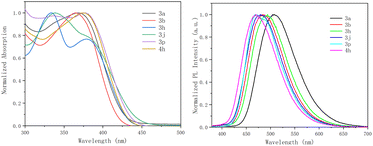 |
| Fig. 2 Normalized absorption (left) and emission (right) spectra of 3a, 3b, 3h, 3j, 3p, and 4h in DCM (1 × 10−5 M). | |
Table 2 Photophysical properties of selected products (1 × 10−5 M in DCM)
Compound |
λ
abs
(nm) |
λ
em
(nm) |
Φ
F
|
Absorption maxima.
Fluorescent emission maxima.
Absolute quantum yields (determined with an integrating sphere system).
|
3a
|
368 |
504 |
0.0383 |
3b
|
365 |
484 |
0.0478 |
3h
|
334, 379 |
494 |
0.0282 |
3j
|
339 |
479 |
0.0305 |
3p
|
346, 375 |
470 |
0.0430 |
4h
|
374 |
470 |
0.0288 |
Synthetic applications of a representative product have been demonstrated (Scheme 5). The reaction of 1a and dialkynylphosphine oxide 2a was scaled up to a mmol scale, from which product 3a was isolated in 95% yield. Treatment of 3a with Lawesson's reagent afforded the phosphine sulfide 7 in high yield. The phosphine oxide 3a was reduced by PhSiH3 to give phosphine 8 in excellent yield. Reduction of the phosphine oxide by PhSiH3 followed by protection by borane gave the adduct 9 in 70% yield. Tetrahydrophosphete oxide 10 was formed in 47% yield under a palladium/H2 reductive system. Meanwhile, the tetrahydrophosphete oxide 10 as an organic phosphine catalyst could catalyze deoxygenation of CF3SO2Cl and the reaction with indole to afford C3-trifluoromethylsulfenylation indole 12 in 83% yield.3j
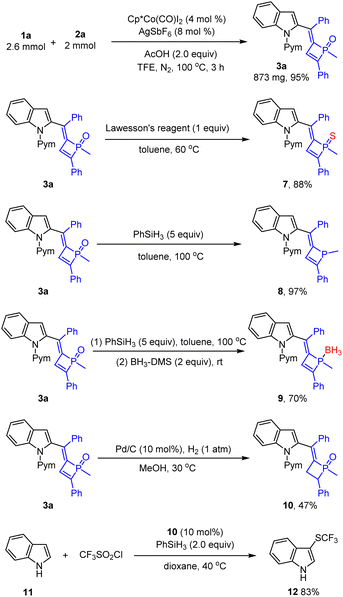 |
| Scheme 5 Synthetic applications of a product. | |
Preliminary mechanistic studies were conducted to gain insight into the reaction mechanism (Schemes 6 and 7). First, an H/D exchange experiment was conducted using CD3COOD as a deuterium source, and H/D scrambling was observed at the C2, C3, and C7 of indole 1a (Scheme 6a), supporting the reversibility of C–H activation at these positions under the reaction conditions. A deuterium labeling experiment was then performed using 1a and 2a in the presence of CD3COOD (Scheme 6b), and analysis of the product by 1H NMR spectroscopy revealed that the 3-position of the indole and olefinic C–H of dihydrophosphete ring was substantially deuterated. The significant deuteration at the olefinic C–H position likely suggests protonolysis of a Co–C bond in the catalytic cycle. Parallel competitive reactions of two electronically distinguishable indoles 1b (R = OMe) and 1d (R = CO2Me) have been conducted, and the more electron-rich indole tends to react with slightly higher reactivity (Scheme 6c). Next, a crossover experiment using a mixture of 2a (R = Me) and 2b (R = OMe) was then performed, and HRMS analysis of the product mixture indicated that no crossover product was present, revealing an intramolecular P
O migration process (Scheme 6d). Moreover, Hammett studies were also performed for a series of indoles with various substituents at the C5-position (see the ESI† for details) and for a series of dialkynylphosphine oxides bearing different para substituents (Scheme 7). A linear correlation was observed for each series through the Hammett plot. A negative ρ value (−0.40 for the dialkynylphosphine oxide series) and (−1.18 for the indole series (see the ESI† for details)) was observed for each plot, and this outcome suggests positive charge accumulation in the transition state, which is stabilized by an electron-donating substituent.
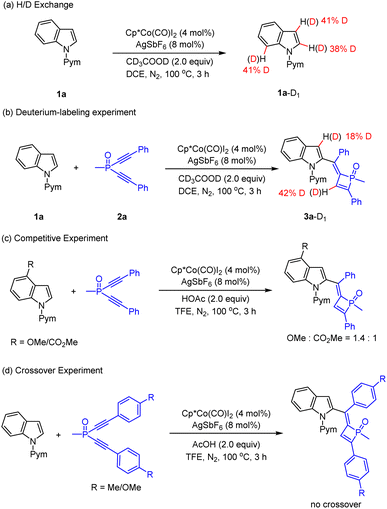 |
| Scheme 6 Mechanistic studies. | |
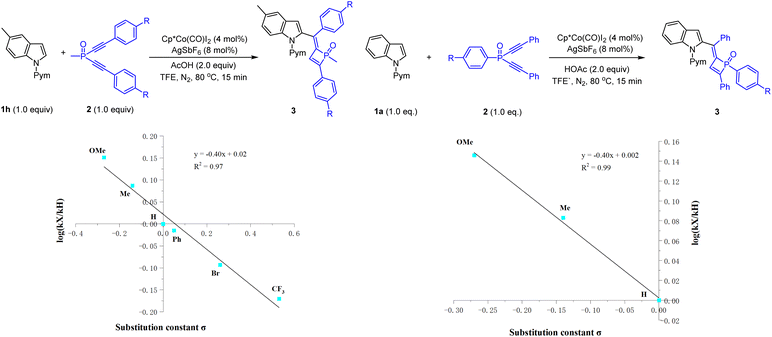 |
| Scheme 7 Hammett studies for the cobalt-catalyzed systems. | |
On the basis of these results and related literature reports of C–H activation-coupling with alkynes, a plausible mechanism for the Co(III)-catalyzed system is proposed in Scheme 8. Starting form a Co(III) caboxylate species, C–H activation occurs at the C(2) position to give the intermediate Bvia cyclometalation. Subsequently, the resulting Co–C(2) bond undergoes migratory insertion into an alkyne unit of the incoming diyne 2a, forming an alkenylcobalt intermediate C. At this stage, two pathways are possible. In route A (Scheme 8, left), β-C(alkynyl) elimination is proposed to give a cobalt alkynyl intermediate with a pendant phosphonium ylide (D). Coordination of the carbanion leads to an alkenyl intermediate E, and the subsequent α insertion of the Co–alkenyl bond into the vinylidene-like C(α) triggers a nucleophilic addition of the C(β) to the phosphonium center. The resulting intermediate F undergoes protonolysis to release the final product and completes the catalytic cycle. Alternatively in route B (Scheme 8, right), the intermediate C is proposed to undergo the 2nd migratory insertion into the alkyne to generate a three-membered phosphacycle (G). Anti-elimination18 of the phosphinate anion gives an enyne intermediate H, where the alkyne unit is activated toward 4-endo-dig cyclization to form the same intermediate F. While it is challenging to unequivocally distinguish between these two pathways by experimental methods, we tend to prefer the route A based on the kinetic studies. Hammett plots (Scheme 7) of both series of Co-catalyzed reactions supported the intermediacy of key cationic species that is stabilized by EDGs. Accordingly, the route A is more likely. On the other hand, the isolation of product 6y in good yields under the Rh-catalyzed conditions starting from the diyne with tert-butyl termini also argues against the route B. This is because the formation of the corresponding rhodium congener of intermediate G will cause strong steric repulsions between the metal center and proximal tBu group.
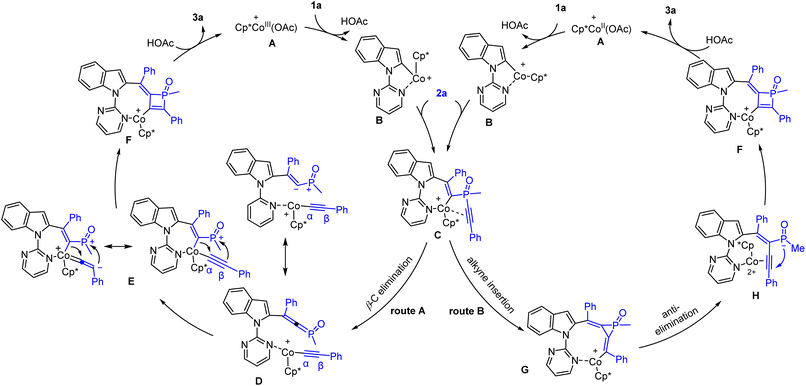 |
| Scheme 8 Proposed possible reaction pathways. | |
Conclusions
In summary, a series of 1,2-dihydrophosphete oxides were efficiently synthesized through a cobalt/rhodium-catalyzed C–H alkenylation/P
O migration sequence via the coupling of indoles/2-pyridone with dialkynylphosphine oxides. A large array of functional groups were tolerated in this catalytic system. The photophysical properties of selected products indicate the potentiality of 1,2-dihydrophosphetes as electroluminescent materials. Mechanistic studies have been performed and Hammett studies suggest build-up of positive charges in the catalytic cycle, and the P-alkynyl group likely undergoes beta-elimination and migratory insertion. Asymmetric reactions of the related chemistry of diynes are underway in our laboratory.
Data availability
Further details of the experimental procedure, 1H and 13C NMR, and X-ray crystallographic data for products 3r and 6m are available in the ESI.†
Author contributions
X. L. conceived the idea and initiated the project. G. Z. performed the initial studies and analyzed the data. S. X. and R. M. performed the experiments. X. L. and S. X. wrote the manuscript.
Conflicts of interest
The authors declare no competing financial interests.
Acknowledgements
Financial support from the NSFC (No. 22371175), research grant from the Central Universities of China (GK202306002), and research fund from the SNNU are gratefully acknowledged.
Notes and references
-
(a) J. Grembecka, A. Mucha, T. Cierpicki and P. Kafarski, The Most Potent Organophosphorus Inhibitors of Leucine Aminopeptidase. Structure-Based Design, Chemistry, and Activity, J. Med. Chem., 2003, 46, 2641–2655 CrossRef CAS PubMed;
(b) Y. Wei and M. Shi, Multifunctional Chiral Phosphine Organocatalysts in Catalytic Asymmetric Morita–Baylis–Hillman and Related Reactions, Acc. Chem. Res., 2010, 43, 1005–1018 CrossRef CAS PubMed;
(c) T. Wang, X. Han, F. Zhong, W. Yao and Y. Lu, Amino Acid-Derived Bifunctional Phosphines for Enantioselective Transformations, Acc. Chem. Res., 2016, 49, 1369–1378 CrossRef CAS PubMed;
(d) W. Huang, S. Liu, D. Zou, M. Thomas, Y. Wang, T. Zhou, J. Romero, A. Kohlmann, F. Li, J. Qi, L. Cai, T. Dwight, Y. Xu, R. Xu, R. Dodd, A. Toms, L. Parillon, X. Lu, R. Anjum, S. Zhang, F. Wang, J. Keats, S. Wardwell, Y. Ning, Q. Xu, L. Moran, Q. Mohemmad, H. Jang, T. Clackson, N. Narasimhan, V. Rivera, X. Zhu, D. Dalgarno and W. Shakespeare, Discovery of Brigatinib (AP26113), a Phosphine Oxide-Containing, Potent, Orally Active Inhibitor of Anaplastic Lymphoma Kinase, J. Med. Chem., 2016, 59, 4948–4964 CrossRef CAS PubMed;
(e) P. Thornton, H. Kadri, A. Miccoli and Y. Mehellou, Nucleoside Phosphate and Phosphonate Prodrug Clinical Candidates, J. Med. Chem., 2016, 59, 10400–10410 CrossRef CAS PubMed;
(f) T. Imamoto, Searching for Practically Useful P-Chirogenic Phosphine Ligands, Chem. Rec., 2016, 16, 2659–2673 CrossRef PubMed;
(g) E. Peris and R. Crabtree, Key factors in pincer ligand design, Chem. Soc. Rev., 2018, 47, 1959–1968 RSC;
(h) H. Guo, Y. Fan, Z. Sun, Y. Wu and O. Kwon, Phosphine Organocatalysis, Chem. Rev., 2018, 118, 10049–10293 CrossRef CAS PubMed;
(i) H. Ni, W. Chan and Y. Lu, Phosphine-Catalyzed Asymmetric Organic Reactions, Chem. Rev., 2018, 118, 9344–9411 CrossRef CAS PubMed;
(j) E. Regulska, P. Hindenberg and C. Romero-Nieto, From Phospha-phenalenes to Diphosphahexaarenes: An Overview of Linearly Fused Six-Membered Phosphorus Heterocycles, Eur. J. Inorg. Chem., 2019, 2019, 1519–1528 CrossRef CAS;
(k) P. van Leeuwen, P. Kamer, J. Reek and P. Dierkes, Ligand Bite Angle Effects in Metal-catalyzed C–C Bond Formation, Chem. Rev., 2000, 100, 2741–2770 CrossRef CAS PubMed;
(l) A. Mucha, P. Kafarski and Ł. Berlicki, Remarkable Potential of the α-Aminophosphonate/Phosphinate Structural Motif in Medicinal Chemistry, J. Med. Chem., 2011, 54, 5955–5980 CrossRef CAS PubMed.
-
(a) T. Lei, G. Liang, Y.-Y. Cheng, B. Chen, C.-H. Tung and L.-Z. Wu, Cobaloxime Catalysis for Enamine Phosphorylation with Hydrogen Evolution, Org. Lett., 2020, 22, 5385–5389 CrossRef CAS PubMed;
(b) H. Hou, B. Zhou, J. Wang, D. Zhao, D. Sun, X. Chen, Y. Han, C. Yan, Y. Shi and S. Zhu, Stereo- and Regioselective cis-Hydrophosphorylation of 1,3-Enynes Enabled by the Visible-Light Irradiation of NiCl2(PPh3)2, Org. Lett., 2021, 23, 2981–2987 CrossRef CAS PubMed;
(c) J. Gbubele and T. Olszewski, Asymmetric synthesis of organophosphorus compounds using H–P reagents derived from chiral alcohols, Org. Biomol. Chem., 2021, 19, 2823–2846 RSC;
(d) D.-L. Zhu, S. Jiang, Q. Wu, H. Wang, L.-L. Chai, H.-Y. Li and H.-X. Li, Visible-Light-Induced Nickel-Catalyzed P(O)–C(sp2) Coupling Using Thioxanthen-9-one as a Photoredox Catalysis, Org. Lett., 2021, 23, 160–165 CrossRef CAS PubMed;
(e) D. Ji, J. Jing, Y. Wang, Z. Qi, F. Wang, X. Zhang, Y. Wang and X. Li, Palladium-catalyzed asymmetric hydrophosphination of internal alkynes: atroposelective access to phosphine-functionalized olefins, Chem, 2022, 8, 3346–3362 CrossRef CAS;
(f) P.-C. Cui, Z.-C. Yin and G.-W. Wang, Visible-Light-Promoted C4-Selective Phosphorylation of Pyridine and Quinoline Derivatives, Org. Lett., 2023, 25, 2663–2668 CrossRef CAS PubMed;
(g) V. Roy and S. Raha Roy, Light-Induced Activation of C–X Bond via Carbonate-Assisted Anion–π Interactions: Applications to C–P and C–B Bond Formation, Org. Lett., 2023, 25(6), 923–927 CrossRef CAS PubMed;
(h) D. Ji, Z. Qi and X. Li, Palladium-Catalyzed Regio- and Enantioselective Hydrophosphination of gem-Difluoroallenes, Org. Lett., 2023, 25, 5957–5962 CrossRef CAS PubMed;
(i) R. Cui, Y. Wang, L. Yuwen, L. Gao, Z. Huang, W.-H. Wang and Q.-W. Zhang, Ni-Catalyzed Asymmetric C–P Cross-Coupling Reaction for the Synthesis of Chiral Heterocyclic Phosphine Oxides, Org. Lett., 2023, 25, 6139–6142 CrossRef CAS PubMed;
(j) S. Zhang, N. Jiang, J.-Z. Xiao, G.-Q. Lin and L. Yin, Copper(I)-Catalyzed Asymmetric Hydrophosphination of 3,3-Disubstituted Cyclopropenes, Angew. Chem., Int. Ed., 2023, 62, e20221879 Search PubMed;
(k) W. Luo, F. Xu, Z. Wang, J. Pang, Z. Wang, Z. Sun, A. Peng, X. Cao and L. Li, Chemodivergent Staudinger Reactions of Secondary Phosphine Oxides and Application to the Total Synthesis of LL-D05139β Potassium Salt, Angew. Chem., Int. Ed., 2023, 62, e20231011 Search PubMed;
(l) Y. Masuda, D. Ikeshita, K. Higashida, M. Yoshida, N. Ishida, M. Murakami and M. Sawamura, Photocatalytic 1,2-Phosphorus-Migrative [3 + 2] Cycloaddition of Tri(t-butyl)phosphine with Terminal Alkynes, J. Am. Chem. Soc., 2023, 145, 19060–19066 CrossRef CAS PubMed.
-
(a) G. Li, Y. Kanda, S. Hong and A. Radosevich, Enabling Reductive C–N Cross-Coupling of Nitroalkanes and Boronic Acids by Steric Design of P(III)/P(V)=O Catalysts, J. Am. Chem. Soc., 2022, 144, 8242–8248 CrossRef CAS PubMed;
(b) J. Tönjes, L. Longwitz and T. Werner, Poly(methylhydrosiloxane) as a reductant in the catalytic base-free Wittig reaction, Green Chem., 2021, 23, 4852–4857 RSC;
(c) L. Longwitz and P.-D. Thomas Werner, Reduction of Activated Alkenes by PIII/PV Redox Cycling Catalysis, Angew. Chem., Int. Ed., 2020, 59, 2760–2763 CrossRef CAS PubMed;
(d) J. Lin, Y. Zhu, W. Cai and Y. Huang, Phosphine-Mediated Sequential [2+4]/[2+3] Annulation to Construct Pyrroloquinolines, Org. Lett., 2022, 24, 1593–1597 CrossRef CAS PubMed;
(e) S. Hong and A. Radosevich, Chemoselective Primary Amination of Aryl Boronic Acids by PIII/PV=O-Catalysis: Synthetic Capture of the Transient Nef Intermediate HNO, J. Am. Chem. Soc., 2022, 144, 8902–8907 CrossRef CAS PubMed;
(f) J. Buonomo, M. Cole, C. Eiden and C. Aldrich, 1,3-Diphenyldisiloxane Enables Additive-Free Redox Recycling Reactions and Catalysis with Triphenylphosphine, Synthesis, 2020, 52, 3583–3594 CrossRef CAS;
(g) P. Finkbeiner, J. Hehn and C. Gnamm, Phosphine Oxides from a Medicinal Chemist's Perspective: Physicochemical and in Vitro Parameters Relevant for Drug Discovery, J. Med. Chem., 2020, 63, 7081–7107 CrossRef CAS PubMed;
(h) T. Nykaza, G. Li, J. Yang, M. Luzung and A. Radosevich, PIII/PV=O Catalyzed Cascade Synthesis of N-Functionalized Azaheterocycles, Angew. Chem., Int. Ed., 2020, 59, 4505–4510 CrossRef CAS PubMed;
(i) L. Longwitz, A. Spannenberg and T. Werner, Phosphetane Oxides as Redox
Cycling Catalysts in the Catalytic Wittig Reaction at Room Temperature, ACS Catal., 2019, 9, 9237–9244 CrossRef CAS;
(j) A. Ghosh, M. Lecomte, S.-H. Kim-Lee and A. Radosevich, Organophosphorus-Catalyzed Deoxygenation of Sulfonyl Chlorides: Electrophilic (Fluoroalkyl)sulfenylation by PIII/PV=O Redox Cycling, Angew. Chem., Int. Ed., 2019, 58, 2864–2869 CrossRef CAS PubMed;
(k) T. Nykaza, J. Cooper, G. Li, N. Mahieu, A. Ramirez, M. Luzung and A. Radosevich, Intermolecular Reductive C–N Cross Coupling of Nitroarenes and Boronic Acids by PIII/PV=O Catalysis, J. Am. Chem. Soc., 2018, 140, 15200–15205 CrossRef CAS PubMed;
(l) T. Nykaza, A. Ramirez, T. Harrison, M. Luzung and A. Radosevich, Biphilic Organophosphorus-Catalyzed Intramolecular Csp2–H Amination: Evidence for a Nitrenoid in Catalytic Cadogan Cyclizations, J. Am. Chem. Soc., 2018, 140, 3103–3113 CrossRef CAS PubMed;
(m) H. Chen, S. Pascal, Z. Wang, P.-A. Bouit, Z. Wang, Y. Zhang, D. Tondelier, B. Geffroy, R. Réau, F. Mathey, Z. Duan and M. Hissler, 1,2-Dihydrophosphete: A Platform for the Molecular Engineering of Electroluminescent Phosphorus Materials for Light-Emitting Devices, Chem.–Eur. J., 2014, 20, 9784–9793 CrossRef CAS PubMed;
(n) T. Imamoto, K. Crépy and K. Katagiri, Optically active 1,1′-di-tert-butyl-2,2′-dibenzophosphetenyl: a highly strained P-stereogenic diphosphine ligand, Tetrahedron: Asymmetry, 2004, 15, 2213–2218 CrossRef CAS.
- A. Marinetti, J. Fischer and F. Mathey, Carbonylation of a strained phosphorus-carbon bond, conversion of phosphirene into 2-keto-1,2-dihydrophosphete complexes: an entry into the chemistry of the phosphorus analogs of unsaturated. β-Lactams, J. Am. Chem. Soc., 1985, 107, 5001–5002 CrossRef CAS.
- A. Marinetti and F. Mathey, [2 + 2] Cycloadditions between electron-poor phospha-alkene complexes and electron-rich alkenes or alkynes, a new route to phosphetane and 1,2-dihydrophosphete rings, J. Chem. Soc., Chem. Commun., 1990, 153–154 RSC.
- K. Doxsee, G. Shen and C. Knobler, Uses of metallacyclo-butenes in heterocyclic synthesis. Synthesis and structural characterization of 1,2-dihydrophosphete, J. Am. Chem. Soc., 1989, 111, 9129–9130 CrossRef CAS.
- F. Roesler, M. Kovács, C. Bruhn, Z. Kelemen and R. Pietschnig, Phosphetes via Transition Metal Free Ring Closure – Taking the Proper Turn at a Thermodynamic Crossing, Chem.–Eur. J., 2021, 27, 9782–9790 CrossRef CAS PubMed.
-
(a) N. Huy, L. Ricard and F. Mathey, Synthesis of the phosphorus-carbon double bond by reaction between pentacarbonylchromium or -tungsten complexes of phosphinidenes and carbenes. Application to the synthesis of the 1,2-dihydrophosphete ring, Organometallics, 1988, 7, 1791–1795 CrossRef;
(b) J. Slootweg, S. Krill, F. Kanter, M. Schakel, A. Ehlers, M. Lutz, A. Spek and K. Lammertsma, Valence Isomerization of 2-Phosphabicyclo-[1.1.0]butanes, Angew. Chem., Int. Ed., 2005, 44, 6579–6582 CrossRef CAS PubMed;
(c) H. Walsgrove, B. Patrick and D. Gates, Transition Metal-Induced Cyclization of 1-Phosphabutadienes: Selective Formation of 1-Phosphet-2-ene or P=C-Substituted Phosphacyclohexene Ligands, Organometallics, 2022, 41, 3399–3410 CrossRef CAS.
-
(a) W. Li, W. Xu, J. Xie, S. Yu and C. Zhu, Distal radical migration strategy: an emerging synthetic means, Chem. Soc. Rev., 2018, 47, 654–667 RSC;
(b) M. Korb and H. Lang, The anionic Fries rearrangement: a convenient route to ortho-functionalized aromatics, Chem. Soc. Rev., 2019, 48, 2829–2882 RSC;
(c) X. Wu and C. Zhu, Radical-Mediated Remote Functional Group Migration, Acc. Chem. Res., 2020, 53, 1620–1636 CrossRef CAS PubMed;
(d) X. Wu, Z. Ma, T. Feng and C. Zhu, Radical-mediated rearrangements: past, present, and future, Chem. Soc. Rev., 2021, 50, 11577–11613 RSC;
(e) S. Dong, X. Liu and X. Feng, Asymmetric Catalytic Rearrangements with α-Diazocarbonyl Compounds, Acc. Chem. Res., 2022, 55, 415–428 CrossRef CAS PubMed.
- C. Lukehart, A. McPhail, D. McPhail, J. Myers Jr and H. Soni, Transformation of a trialkynylphosphine oxide to a 2-alkylidene-1,2-dihydro-3-phosphete P-oxide ligand by Pt-H addition and rearrangement reactions. Activation of molecular hydro-gen by a platinum(II) complex, Organometallics, 1989, 8, 1007–1014 CrossRef CAS.
-
(a) L. Dupuis, N. Pirio, P. Meunier, A. Igau, B. Donnadieu and J. Majoral, Zirconocen–Benzyme-Mediated Intramolecular Coupling of Bis(alkynyl)phosphane: A Way to Mono- and Tricyclic 1,2-Dihydrophosphetes, Angew Chem. Int. Ed. Engl., 1997, 36, 987–989 CrossRef CAS;
(b) N. Pirio, S. Bredeau, L. Dupuis, P. Schutz, B. Donnadieu, A. Igau, J. Majoral, J. Guillemin and P. Meunier, Intramolecular coupling of acetylenic groups of bis(alkynyl)phosphanes and silanes mediated by benzynezirconocene: a route to new mono- and tricyclic heterocycles, Tetrahedron, 2004, 60, 1317–1327 CrossRef CAS.
- T. Xin and C. Cummins, Synthesis of Phosphet-2-one Derivatives via Phosphinidene Transfer to Cyclopropenones, J. Am. Chem. Soc., 2023, 145, 25989–25994 CrossRef CAS PubMed.
-
(a) G. Nishida, K. Noguchi, M. Hirano and K. Tanaka, Enantioselective Synthesis of P-Stereogenic Alkynylphosphine Oxides by Rh-Catalyzed [2+2+2] Cycloaddition, Angew. Chem., Int. Ed., 2008, 47, 3410–3413 CrossRef CAS PubMed;
(b) N. Fukawa, T. Osaka, K. Noguchi and K. Tanaka, Asymmetric Synthesis and Photophysical Properties of Benzopyrano- or Naphthopyrano-Fused Helical Phosphafluorenes, Org. Lett., 2010, 12, 1324–1327 CrossRef CAS PubMed;
(c) A. Kondoh, H. Yorimitsu and K. Oshima, Synthesis of Bulky Phosphines by Rhodium-Catalyzed Formal [2 + 2 + 2] Cycloaddition Reactions of Tethered Diynes with 1-Alkynylphosphine Sulfides, J. Am. Chem. Soc., 2007, 129, 6996–6997 CrossRef CAS PubMed;
(d) X. Li, G. Hu, P. Luo, G. Tang, Y. Gao, P. Xu and Y. Zhao, Palladium(II)-Catalyzed Hydration of Alkynylphosphonates to β-Ketophosphonates, Adv. Synth. Catal., 2012, 354, 2427–2432 CrossRef CAS;
(e) Y. Zheng, L. Guo and W. Zi, Enantioselective and Regioselective Hydroetherification of Alkynes by Gold-Catalyzed Desymmetrization of Prochiral Phenols with P-Stereogenic Centers, Org. Lett., 2018, 20, 7039–7043 CrossRef CAS PubMed;
(f) S. Zhang, H. Cheng, S. Mo, S. Yin, Z. Zhang and T. Wang, Gold(I)-Catalyzed Synthesis of Six-Membered P,O-Heterocycles via Hydration/Intramolecular Cyclization Cascade Reaction, Adv. Synth. Catal., 2019, 361, 4227–4231 CrossRef CAS;
(g) Y. Zhang, F. Zhang, L. Chen, J. Xu, X. Liu and X. Feng, Asymmetric Synthesis of P-Stereogenic Compounds via Thulium(III)-Catalyzed Desymmetrization of Dialkynylphosphine Oxides, ACS Catal., 2019, 9, 4834–4840 CrossRef CAS;
(h) A. Gupta and B. Flynn, Electrophilic Activation of P-Alkynes in the Synthesis of P-Substituted and P-Centered Heterocycles, J. Org. Chem., 2016, 81, 4012–4019 CrossRef CAS PubMed;
(i) S. Doherty, J. Knight, D. Perry, N. Ward, D. Bittner, W. McFarlane, C. Wills and M. Probert, Triaryl-Like MONO-, BIS-, and TRISKITPHOS Phosphines: Synthesis, Solution NMR Studies, and a Comparison in Gold-Catalyzed Carbon–Heteroatom Bond Forming 5-exo-dig and 6-endo-dig Cyclizations, Organometallics, 2016, 35, 1265–1278 CrossRef CAS;
(j) A. Klose, G. Kehr, C. Daniliuc and G. Erker, Phosphole formation by 1,1-carboboration – reactions of bis-alkynyl phosphanes with a frustrated P/B Lewis pair, Dalton Trans., 2016, 45, 2023–2030 RSC;
(k) J. Mçbus, G. Kehr, C. Daniliuc, C. Mück-Lichtenfeld and G. Erker, Observation of a Thermally Induced Bora-Nazarov Cyclization at a Phosphole Framework, Angew. Chem., Int. Ed., 2015, 54, 12366–12369 CrossRef PubMed.
-
(a) G. Liao, T. Zhang, Z.-K. Lin and B.-F. Shi, Transition Metal-Catalyzed Enantioselective C–H Functionalization via Chiral Transient Directing Group Strategies, Angew. Chem., Int. Ed., 2020, 59, 19773–19786 CrossRef CAS PubMed;
(b) B. Li, A. Ali and H. Ge, Recent Advances in Using Transition-Metal-Catalyzed C–H Functionalization to Build Fluorescent Materials, Chem, 2020, 6, 2591–2657 CrossRef CAS;
(c) S. Hong, Y. Hwang, M. Lee and S. Chang, Mechanism-Guided Development of Transition-Metal-Catalyzed C–N Bond-Forming Reactions Using Dioxazolones as the Versatile Amidating Source, Acc. Chem. Res., 2021, 54, 2683–2700 CrossRef CAS PubMed;
(d) B. Liu, A. Romine, C. Rubel, K. Engle and B. Shi, Transition-Metal-Catalyzed, Coordination-Assisted Functionalization of Nonactivated C(sp3)–H Bonds, Chem. Rev., 2021, 121, 14957–15074 CrossRef CAS PubMed;
(e) R. Mandal, B. Garai and B. Sundararaju, Weak-Coordination in C–H Bond Functionalizations Catalyzed by 3d Metals, ACS Catal., 2022, 12, 3452–3506 CrossRef CAS;
(f) Y. He, Z. Huang, K. Wu, J. Ma, Y.-G. Zhou and Z. Yu, Recent advances in transition-metal-catalyzed carbene insertion to C–H bonds, Chem. Soc. Rev., 2022, 51, 2759–2852 RSC;
(g) Y. Pan, X. Qin, C. Yuan and Y. Lu, Application of Ligands in Cp*Rh(III)-Catalyzed C–H Bond Functionalization Reaction, Chin. J. Org. Chem., 2023, 43, 924–948 CrossRef CAS;
(h) H. Cao, Q. Cheng and A. Studer, meta-Selective C–H Functionalization of Pyridines, Angew. Chem., Int. Ed., 2023, 62, e202302941 CrossRef CAS PubMed;
(i) Z. Zeng, H. Gao, Z. Zhou and W. Yi, Intermolecular Redox-Neutral Carboamination of C–C Multiple Bonds Initiated by Transition-Metal-Catalyzed C–H Activation, ACS Catal., 2022, 12, 14754–14772 CrossRef CAS;
(j) P.-S. Wang and L.-Z. Gong, Palladium-Catalyzed Asymmetric Allylic C–H Functionalization: Mechanism, Stereo- and Regioselectivities, and Synthetic Applications, Acc. Chem. Res., 2020, 53, 2841–2854 CrossRef CAS PubMed;
(k) B.-B. Zhan, M.-X. Jiang and B.-F. Shi, Late-stage functionalization of peptides via a palladium-catalyzed C(sp3)–H activation strategy, Chem. Commun., 2020, 56, 13950–13958 RSC;
(l) Y. Qiu, C. Zhu, M. Stangier, J. Struwe and L. Ackermann, Rhodaelectro-Catalyzed C–H and C–C Activation, CCS Chem., 2020, 2, 1529–1552 Search PubMed;
(m) R. Manoharan and M. Jeganmohan, Recent Advancements in Allylic C(sp3)–H Functionalization of Olefins Catalyzed by Rh(III) or Ir(III) Complexes, Eur. J. Org Chem., 2020, 2020, 7304–7319 CrossRef CAS;
(n) S. Pandit, S. Maiti and D. Maiti, Noncovalent interactions in Ir-catalyzed remote C–H borylation: a recent update, Org. Chem. Front., 2021, 8, 4349–4358 RSC;
(o) H. Wen, G. Liu and Z. Huang, Recent Advances in Tridentate Iron and Cobalt Complexes for Alkene and Alkyne Hydrofunctionalizations, Coord. Chem. Rev., 2019, 386, 138–153 CrossRef CAS;
(p) W. Ai, R. Zhong, X. Liu and Q. Liu, Hydride Transfer Reactions Catalyzed by Cobalt Complexes, Chem. Rev., 2019, 119, 2876–2953 CrossRef CAS PubMed;
(q) L. Lukasevics, A. Cizikovs and L. Grigorjeva, C–H Functionalization by High-Valent Cobalt Catalysis: Current Progress, Challenges and Future Perspectives, Chem. Commun., 2021, 57, 10827–10841 RSC;
(r) S. Jana, V. Mayerhofer and C. Teskey, Photo- and Electrochemical Cobalt Catalyzed Hydrogen Atom Transfer for the Hydrofunctionalisation of Alkenes, Angew. Chem., Int. Ed., 2023, 62, e202304882 CrossRef CAS PubMed.
-
(a) H. Zhang, M. Sun, Y. Ma, Q. Tian and S. Yang, Nickel-catalyzed C–P cross-coupling of diphenylphosphine oxide with aryl chlorides, Org. Biomol. Chem., 2012, 10, 9627–9633 RSC;
(b) J. Yang, T. Chen and L. Han, C-P bond-forming reactions
via C–O/P–H cross-coupling catalyzed by nickel, J. Am. Chem. Soc., 2015, 137, 1782–1785 CrossRef CAS PubMed;
(c) W. Fu, C. So and F. Kwong, Palladium-Catalyzed Phosphorylation of Aryl Mesylates and Tosylates, Org. Lett., 2015, 17, 5906–5909 CrossRef CAS PubMed;
(d) Y. He, H. Wu and F. Toste, A dual catalytic strategy for carbon-phosphorus cross-coupling via gold and photoredox catalysis, Chem. Sci., 2015, 6, 1194–1198 RSC;
(e) Y.-Y. Gui, L. Sun, Z.-P. Lu and D.-G. Yu, Photoredox sheds new light on nickel catalysis: from carbon–carbon to carbon–heteroatom bond formation, Org. Chem. Front., 2016, 3, 522–526 RSC;
(f) S. Ung, V. Mechrouk and C.-J. Li, Shining Light on the Light-Bearing Element: A Brief Review of Photomediated C–H Phosphorylation Reactions, Synthesis, 2021, 53, 1003–1022 CrossRef CAS;
(g) Y. Zhu, W. Zu, Q. Tian, Z. Cao, Y. Wei and L. Xu, A nickel/organoboron catalyzed metal-laphotoredox platform for C(sp2)–P and C(sp2)–S bond construction, Org. Chem. Front., 2022, 9, 1070–1076 RSC;
(h) Y. Li, X. Jin, P. Liu, H. Zhang, X. Yu, Y. Liu, B. Liu and W. Yang, Copper-Catalyzed Dynamic Kinetic C–P Cross-Coupling/Cyclization for the Concise Asymmetric Synthesis of Six-, Seven- and Eight-Membered P-Stereogenic Phosphorus Heterocycles, Angew. Chem., Int. Ed., 2022, 61, e202117093 CrossRef CAS PubMed;
(i) S.-F. Wu, G.-K. Zhang, X. Wang, Z.-J. He, Y.-C. Zhang and F. Shi, Organocatalytic Diastereoselective (4 + 1) Cycloaddition of o-Hydroxyphenyl-Substituted Secondary Phosphine Oxides, J. Org. Chem., 2023, 88, 16497–16510 CrossRef CAS PubMed;
(j) J.-H. Wu, S. Fang, X. Zheng, J. He, Y. Ma, Z. Su and T. Wang, Organocatalytic Dynamic Kinetic Resolution Enabled Asymmetric Synthesis of Phosphorus-Containing Chiral Helicenes, Angew. Chem., Int. Ed., 2023, 62, e202309515 CrossRef CAS PubMed.
- Deposition number 2203562 (for 3r) contains the supplementary crystallographic data for this paper.
- Deposition number 2203561 (for 6m) contains the supplementary crystallographic data for this paper.
- F. Della-Felice, M. Zanini, X. Jie, E. Tan and A. Echavarren, Rhodium(III)-Catalyzed Synthesis of Skipped Enynes via C(sp3)–H Alkynylation of Terminal Alkenes, Angew. Chem., Int. Ed., 2021, 60, 5693–5698 CrossRef CAS PubMed.
|
This journal is © The Royal Society of Chemistry 2024 |
Click here to see how this site uses Cookies. View our privacy policy here.