DOI:
10.1039/D4SC00650J
(Edge Article)
Chem. Sci., 2024,
15, 7178-7186
Tuning vibration-induced emission through macrocyclization and catenation†
Received
28th January 2024
, Accepted 6th April 2024
First published on 11th April 2024
Abstract
In order to investigate the effect of macrocyclization and catenation on the regulation of vibration-induced emission (VIE), the typical VIE luminogen 9,14-diphenyl-9,14-dihydrodibenzo[a, c]phenazine (DPAC) was introduced into the skeleton of a macrocycle and corresponding [2]catenane to evaluate their dynamic relaxation processes. As investigated in detail by femtosecond transient absorption (TA) spectra, the resultant VIE systems revealed precisely tunable emissions upon changing the solvent viscosity, highlighting the key effect of the formation of [2]catenane. Notably, the introduction of an additional pillar[5]arene macrocycle featuring unique planar chirality endows the resultant chiral VIE-active [2]catenane with attractive circularly polarized luminescence in different states. This work not only develops a new strategy for the design of new luminescent systems with tunable vibration induced emission, but also provides a promising platform for the construction of smart chiral luminescent materials for practical applications.
Introduction
Smart organic materials with tunable and switchable luminescence have shown great potential for wide applications such as imaging,1 sensors,2 and optical displays.3 Therefore, the development of novel luminogens which are sensitive to external stimuli, such as light,4 solvent polarity,5 solvent viscosity,6 pH,7 ambient temperature,8 and pressure,9 remains an attractive topic. Among the diverse luminogens, single-molecule fluorophores with multiple emissions have received continuous attention due to their basic research interest and potential high-tech applications.10 In particular, a series of dihydrophenazine (DHP) derivatives with anomalously large Stokes shifts and multiple emissions, especially ones based on the core of 9,14-diphenyl-9,14-dihydrodibenzo[a, c]phenazine (DPAC), have been successfully developed by Tian, Chou, and other groups.11 DPAC exhibits a V-shaped bent conformation in the ground state, and under light excitation, its lowest excited state can relax into a nearly planar structure through the vibration of the phenazine unit, ultimately resulting in long wave emissions, and thus they termed this interesting phenomenon as ‘vibration-induced emission (VIE)’.11a,b Previous studies have proven that the vibrational transition from the bending state of the lowest excited state to the planar state can be efficiently regulated by external stimuli such as temperature or solvent viscosity, leading to multiple emission behaviors.11c Attributed to such attractive features, so far DPAC-based materials have been widely used in ion probes,12 thermometers,13 biosensors,14 white light emitting materials15 and so on.
Notably, the design of novel emitters with tunable VIE behaviors is not trivial. In previous studies, more attention has been paid to the locking of the DPAC unit through the formation of either covalent or noncovalent macrocycles. Such a design strategy has been proven to be reliable, leading to the expected tunable multi-color emissions for practical uses.12b,c,16 Notably, considering that macrocycles could serve as the key components for the construction of mechanically interlocked molecules (MIMs) such as rotaxanes and catenanes, the further construction of DPAC-based MIMs will be of great importance. On one hand, the formation of MIMs would serve as an efficient method for emission regulation attributed to the confined yet flexible environment of the mechanical bond, thus possibly adding a new dimension for VIE tunability.17 Notably, in their inspiring studies, Goldup et al. and Qu et al. have already proven the great power of mechanical bonds in regulating the emission performances of luminogens.18 On the other hand, the existence of other components within DPAC-based MIMs makes them promising platforms for integration with other functional groups, thus endowing them with interesting properties and functions. For instance, for DPAC-based [2]catenane with an additional chiral macrocycle, attractive circularly polarized luminescence (CPL) performances would emerge. However, the design and construction of novel DPAC-based MIMs, particularly chiral ones, have been rarely explored.
Based on our ongoing interest in MIMs,19 particularly ones with switchable CPL,20 in this study, the DPAC unit was inserted into the skeletons of a macrocycle and a corresponding [2]catenane with the pillar[5]arene macrocycle (Fig. 1). Detailed femtosecond transient absorption (TA) study suggested that the macrocyclization and catenation led to precisely tunable VIE behaviors upon changing the solvent viscosity. In addition, the introduction of pillar[5]arene with unique planar chirality21 into the [2]catenane endows it with interesting CPL performances in different states, thus providing a new platform for the construction of smart chiral luminescent materials for practical applications.
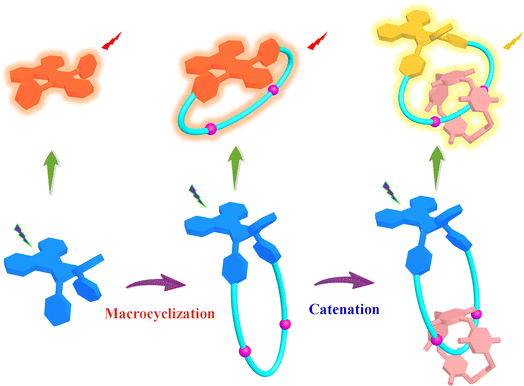 |
| Fig. 1 Design strategy of the DPAC-functionalized macrocycle and chiral [2]catenane with tunable VIE behaviors. | |
Results and discussion
Design and synthesis of the DPAC-functionalized macrocycle and [2]catenane
In our design strategy, to investigate the influence of macrocyclization and catenation on the VIE behaviors, the DPAC unit as a typical VIE luminogen was first introduced into a macrocycle to tune the VIE through possible conformation locking. Moreover, an additional macrocycle was then combined with the resultant DPAC-functionalized macrocycle to give rise to the formation of corresponding DPAC-functionalized [2]catenane. More importantly, along with such a catenation process, enhanced conformation restriction would be achieved, possibly leading to interesting VIE behaviors. With such a design strategy in mind, in this study, in order to increase the synthetic efficiency, the [2]rotaxane 2 with diethyoxypillar[5]arene (DEP[5]A) as the wheel component and pentafluorophenyl ester units as exchange stoppers as well as corresponding axle component 3 without DEP[5]A were first prepared (Scheme S1†).22 Starting from key intermediate 1 with the DPAC core and two NH2 tails, the targeted DPAC-functionalized [2]catenane DPAC-C and macrocycle DPAC-M were successfully synthesized through the transamidation reactions with 2 and 3, respectively, in 27% and 32% yields under high dilute conditions(Fig. 2, Scheme S1†).
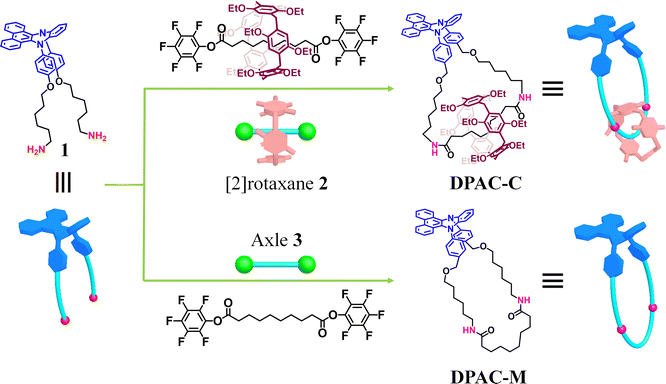 |
| Fig. 2 Synthesis of novel DPAC-functionalized macrocycle DPAC-M and [2]catenane DPAC-C. Reaction conditions: 1 (1.0 equiv.), 2 or 3 (1.0 equiv.), THF (∼0.1 mM), r.t., 48 h, 27% for DPAC-C and 32% for DPAC-M. | |
The resultant macrocycle DPAC-M and [2]catenane DPAC-C were well characterized by one-dimensional (1D) (1H and 13C) NMR and HRMS (ESI-TOF-MS) analysis. For instance, for macrocycle DPAC-M, the characteristic peaks at 5.60–5.57 ppm as typical signals for amide bonds were observed, which preliminarily proved the generation of the targeted macrocyclic structure (Fig. S14†). In the case of [2]catenane DPAC-C, the characteristic peaks attributed to both DPAC and DEP[5]A units with 1
:
1 integration ratio were observed, indicating the formation of an integrated molecule (Fig. S17†). Moreover, the proton signals attributed to the amide bonds (H5) and methylene moieties (H12) revealed remarkable upfield shifts due to the shielding effect of the DEP[5]A macrocycle, suggesting the formation of the expected interlocked structure (Fig. 3a). In addition, all the proton signals attributed to the DEP[5]A macrocycle displayed obvious upfield shifts, indicating that the DPAC-M ring in the [2]catenane structure also has a shielding effect on the DEP[5]A macrocycle. More importantly, in the HRMS (ESI-TOF-MS) spectra, single characteristic peaks (m/z = 859.5127 and 1751.0162) attributed to [DPAC-M + H]+ and [DPAC-C + H]+ were observed, respectively, which are consistent with the theoretical values of 859.5162 and 1751.0165 (Fig. S16 and S19†), again confirming the successful synthesis of the targeted macrocycle and [2]catenane. Notably, in the 1H NMR spectra of [2]catenane DPAC-C (Fig. 3a and S22†), only broadened signals with chemical shifts between −1.5 ppm and 2.5 ppm were observed, while the typical peaks of the pillararene/alkyl chain interlocked system were not found, which might be due to the rapid motion of the long alkyl chain moiety of the DPAC-M component. In order to further investigate such motion behavior of the DPAC-M component within the [2]catenane, 1H NMR spectra of [2]catenane DPAC-C at different temperatures were then collected. As shown in Fig. 3b and S23,† upon cooling the sample to −15 °C, broadened signals with chemical shifts in the range of −2.5 ppm to −1.0 ppm started to appear. Further lowering the temperature to −75 °C led to the emergence of seven sets of signals in the range of −3.0 to 0.0 ppm, which can be attributed to the typical signals of the pillararene/alkyl chain interlocked system, again confirming the successful formation of the targeted [2]catenane skeleton. In addition, considering that the solvent viscosity would also influence the motion behaviors of the ring component, ethylene glycol (EG), which is highly viscous and miscible with THF, was then chosen to investigate the effect of solvent viscosity. Unfortunately, according to the 1H NMR study shown in Fig. S24,† the gradual increase in the proportions of EG-d6 in THF-d8 solution (0.5 mM) of [2]catenane DPAC-C did not lead to the emergence of the typical signals below 0 ppm, suggesting negligible effect of the solvent viscosity on ring component motions within the [2]catenane DPAC-C.
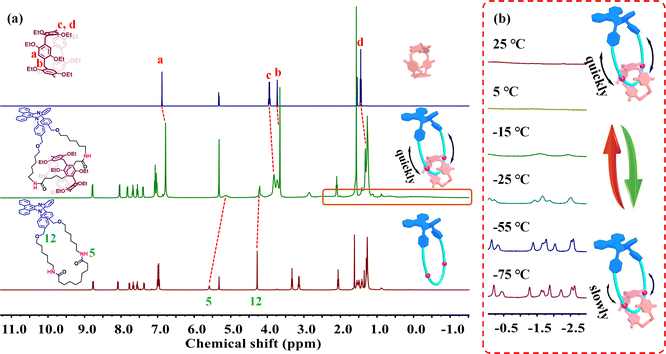 |
| Fig. 3 (a) 1H NMR spectra (CD2Cl2, 298 K, 500 MHz) of macrocycle DPAC-M (bottom), [2]catenane DPAC-C (middle), and the wheel component DEP[5]A (top); (b) partial 1H NMR spectra (CD2Cl2, 500 MHz) of [2]catenane DPAC-C at different temperatures. (Inset) cartoon representation of the motion behaviors of macrocycle DPAC-M within the [2]catenane DPAC-C. | |
Tunable VIE behaviors of the DPAC-functionalized macrocycle and [2]catenane
With the targeted DPAC-functionalized macrocycle DPAC-M and [2]catenane DPAC-C in hand, their steady-state photophysical properties were first measured in THF. As revealed by their normalized absorption and emission spectra shown in Fig. 4b, both the absorption onset and peak wavelength of macrocycle DPAC-M are similar to those of DPAC, both at 410 nm and 350 nm, respectively, indicating that the formation of the macrocycle does not remarkably change the absorption of DPAC in the ground state. Notably, the absorption peak of DPAC-M is slightly widened. However, for [2]catenane DPAC-C, obvious red shifts of both the absorption onset (to 415 nm) and peak wavelength (to 357 nm) were observed. These results suggested that along with the formation of [2]catenane DPAC-C, a more planar conformation of the DPAC unit was favored by rendering large π-delocalization attributed to the existence of the DEP[5]A macrocycle that occupies the cavity of the DPAC-M macrocycle.
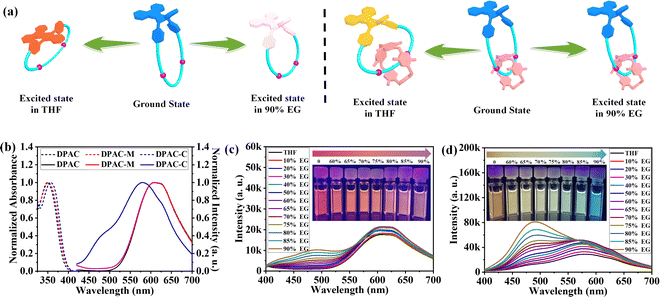 |
| Fig. 4 (a) Cartoon representation of the ground and excited states of macrocycle DPAC-M and [2]catenane DPAC-C; (b) normalized absorption (dashed line) and fluorescence spectra (solid line) of DPAC, macrocycle DPAC-M, and [2]catenane DPAC-C in THF (λex = 360 nm, 0.01 mM); fluorescence spectra of macrocycle DPAC-M (c) and [2]catenane DPAC-C (d) in THF/EG with different EG fractions (λex = 360 nm, 0.01 mM). (Inset): Photographs upon UV lamp excitation at 365 nm. | |
In addition to the absorption spectra of macrocycle DPAC-M and [2]catenane DPAC-C that are slightly different from that of DPAC, their emission spectra are also different. For macrocycle DPAC-M, although the emission peaks at 550–700 nm are similar to that of DPAC, it can be clearly observed that the emission of macrocycle DPAC-M at 420–525 nm was greater than that of DPAC. These results indicate that even though the length of the alkyl chain linker in the macrocycle is long enough, the macrocyclic structure still to some extent affects the transition of the excited state of the DPAC unit from a bent conformation to a planar one. More importantly, in the case of [2]catenane DPAC-C, the maximum emission peak revealed a significant blue shift to 580 nm. In addition, emission peaks at ∼490 nm and ∼625 nm were observed, indicating an interesting multiple emission property, which suggested that compared to the macrocyclic structure, the [2]catenane structure further affects the excited state transition of the inserted DPAC unit.
Subsequently, the effect of solvent viscosity on the VIE behaviors of macrocycle DPAC-M and [2]catenane DPAC-C was investigated. To highlight the key effects of the formation of macrocycle and [2]catenane on the precisely tunable emissions of the VIE system when changing solvent viscosity, the absorption and emission spectra of the DPAC unit at different EG ratios were first collected and the spectra indicated that the increase in EG ratio did not have a significant effect on the steady-state photophysical properties of the DPAC unit (Fig. S25a and S26†), while for macrocycle DPAC-M, as shown in Fig. 4c, the emission peak at 490 nm attributed to blue light emission enhanced with the increase of EG ratio. However, the fluorescence quantum yields (ΦF) did not show significant regular changes, ranging from 7.1% to 12.4% (Table S1†). These results demonstrated that for DPAC-M the intensity of short wavelength emission gradually enhanced with the increase of solvent viscosity, thus significantly strengthening the continuous configuration limitation of the DPAC unit in the excited state. In the case of [2]catenane DPAC-C, compared to macrocycle DPAC-M, the continuous configuration of the DPAC unit in the excited state is more significantly restricted upon increasing solvent viscosity, as revealed by the blue light emission when the proportion of EG reached 90% (Fig. 4d). Notably, a remarkable increase in the fluorescence quantum yields from 18.6% to 32.0% was also observed (Table S1†). As the viscosity of the solvent increases, the solutions of the macrocycle and [2]catenane also show significant colour changes at the macroscopic level: for macrocycle DPAC-M, the emission colour gradually changes from orange red to pink (Fig. 4c: Inset), while for [2]catenane DPAC-C, the emission colour changes from yellow to light yellow, then to cyan, and finally to blue (Fig. 4d: Inset). The 1931 CIE coordinate diagram corresponding to different solvent viscosities clearly shows these colour transformation processes (Fig. S27 and Table S2†).
Femtosecond transient absorption (TA) spectra of the VIE-active macrocycle and [2]catenane
To gain a better understanding of such interesting tunable VIE behaviors of the DPAC-functionalized macrocycle and [2]catenane, femtosecond transient absorption (TA) spectroscopy was performed under 360 nm excitation. Since the TA tests require high concentration, the steady-state photophysical properties of macrocycle DPAC-M and [2]catenane DPAC-C at a higher concentration (0.05 mM) were first measured before the TA tests (Fig. S28 and S29†). For macrocycle DPAC-M, compared with that in lower concentration (0.01 mM), only an increase in absorption and emission intensity was observed at different solvent viscosities (Fig. S28a and S29a†), while the CIE coordinates were similar (Fig. S30a and Table S3†), indicating that the concentration did not significantly affect the molecular vibrational relaxation process. However, in the case of [2]catenane DPAC-C, when the proportion of EG reached 90%, [2]catenane DPAC-C started to aggregate and precipitate (Fig. S28b and S29b†), which might hamper the further investigations of excited-state dynamics. Thus, the maximum proportion of EG was set at 85% when investigating the solvent viscosity effect on the excited state conformations of the DPAC unit in [2]catenane DPAC-C.
As revealed by the TA spectra of macrocycle DPAC-M and [2]catenane DPAC-C in THF (Fig. 5b, d, S31 and S33†), compared to that of the sole DPAC unit,23 their initial excited-state absorption (ESA) wavelengths were identical, and thus we speculated that the excited-state dynamics of both the macrocycle and [2]catenane in THF also include the whole process R* → I1* → I2* → P* (Fig. 5a). Therefore, on the basis of previous reports and with the aid of the GloTarAn program,24 we performed the global analysis with a sequential five-component model on these TA data (Fig. 5b and d). Notably, by comparing stimulated emission (SE) in TA and the steady state fluorescence spectra, it can be proven that these species are emissive due to their corresponding relationship. For example, for the TA spectrum of macrocycle DPAC-M in THF (Fig. 5b), significant SE in the ranges of 400–480 nm (the second species, red line), 440–560 nm (the third species, green line), and 520–660 nm (the fourth species, blue line) was observed in the evolution associated difference spectra (EADS) obtained through the global analysis, which corresponds to weak emissions in the range of 400–525 nm and strong emissions in the range of 600–625 nm (Fig. S29a†).
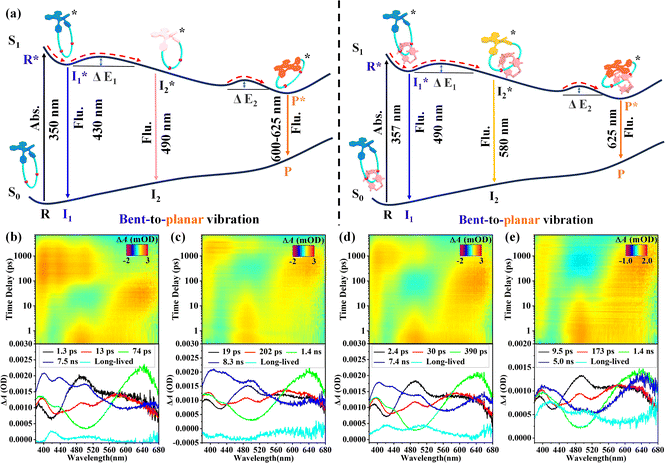 |
| Fig. 5 (a) Schematic potential energy surfaces for the conformational evolution of macrocycle DPAC-M and [2]catenane DPAC-C; femtosecond transient absorption spectra of macrocycle DPAC-M in THF (b) and in THF/EG (v/v = 10/90) (c), and [2]catenane DPAC-C in THF (d) and in THF/EG (v/v = 15/85) (e) with a photoexcitation of 360 nm and corresponding evolution associated difference spectra (EADS) obtained through global analysis. | |
According to the five-component model on the TA data, four spectral species with lifetimes of 1.3 ps, 13 ps, 74 ps, and 7.5 ns, and a long-lived component for macrocycle DPAC-M, and 2.4 ps, 30 ps, 390 ps, and 7.4 ns, and a long-lived component for [2]catenane DPAC-C were obtained (Table 1). For macrocycle DPAC-M, compared to the lifetimes of DPAC (R*, 1.0 ps; I1*, 3.7 ps; I2*, 10 ps; P*, 5.0 ns), the significant increase in the lifetimes of I1* and I2* indicated that the transition energy barriers ΔE1 and ΔE2 of I1* → I2* and I2* → P* in the macrocycle system significantly enhanced, ultimately leading to not only bright P emission consistent with that of the sole DPAC unit, but also small I1 and I2 emissions in the range of 400–525 nm (Fig. 4c and S29a†). These results strongly demonstrated that the locking of the DPAC unit by an alkyl chain linker through the formation of the macrocycle can effectively regulate the excited state conformations of DPAC, while in the case of [2]catenane DPAC-C, the even longer lifetimes of I1* and I2* indicated relatively slower transitions from I1* to I2* and I2* to P* compared with DPAC and macrocycle DPAC-M. Thus, the excited state population can be trapped at I1* and I2* states as well as the P* state, resulting in the ultra-broad triple band yellow fluorescence emission (Fig. 4d and S29b†). According to these results, the formation of the mechanical bond revealed a more significant effect on the regulation of the excited state conformations of DPAC.
Table 1 Lifetimes obtained from global analysis of transient absorption spectra
Lifetimes |
τ
1/ps (R*) |
τ
2/ps (I1*) |
τ
3 (I2*) |
τ
4/ns (P*) |
τ
5 (long-lived) |
DPAC-M in THF |
1.3 ± 0.1 |
13 ± 0.3 |
74 ± 0.9 ps |
7.5 ± 0.3 |
— |
DPAC-C in THF |
2.4 ± 0.1 |
30 ± 0.5 |
390 ± 5.9 ps |
7.4 ± 0.3 |
— |
DPAC-M in THF/EG (v/v = 10/90) |
19 ± 0.8 |
202 ± 5.9 |
1.4 ± 0.1 ns |
8.3 ± 0.5 |
— |
DPAC-C in THF/EG (v/v = 15/85) |
9.5 ± 0.5 |
173 ± 5.2 |
1.4 ± 0.1 ns |
5.0 ± 0.2 |
— |
Subsequently, the effect of solvent viscosity on the excited-state conformations of the DPAC unit in macrocycle DPAC-M and [2]catenane DPAC-C was investigated (Fig. 5c, e, S32 and S34†). For macrocycle DPAC-M (Fig. 5c and Table 1), when the EG proportion was set at 90%, it can be observed that the lifetimes of I1* and I2* were astonishingly different. In particular, the lifetime of I2* reached the nanosecond level. Such significant enhancement in lifespan indicated that in a highly viscous solvent system, the transition energy barriers ΔE1 and ΔE2 of I1* → I2* and I2* → P* significantly increased. Thus, multiple excited states could be stabilized, ultimately leading to a pink emission (Fig. 4c and S29a†). In the case of [2]catenane DPAC-C (Fig. 5f and Table 1), upon setting the EG proportion at 85%, longer lifetimes of I1* and I2* were also observed, again confirming that the high viscosity solvent system significantly enhances these transition energy barriers. More importantly, due to the presence of the mechanical bond, the existence of multiple excited states in [2]catenane led to a cyan emission (Fig. 4d and S29b†). It can be inferred that for [2]catenane, further increasing the viscosity of the solvent system will further limit the vibrational relaxation of the lowest excited state V-shaped bent conformation to the nearly planar structure, ultimately achieving blue emission of [2]catenane DPAC-C. Notably, to probe the surface crossing and population of a triplet state, the microsecond transient absorption spectra of DPAC-M and DPAC-C in THF and viscous solvents were obtained. According to the results shown in Fig. S35,† their kinetics lifetimes in air and N2 are the same, indicating that there is no surface crossing and population of a triplet state in DPAC-M and DPAC-C.
Chiral resolution and circularly polarized luminescence performances of DPAC-functionalized [2]catenane
As confirmed above, the [2]catenane DPAC-C displays attractive tunable emission behaviors attributed to the further regulation of the VIE behavior of the DPAC-M macrocycle through catenation. Moreover, considering that the other macrocyclic component DEP[5]A reveals unique planar chirality, the integrated [2]catenane would display promising CPL performance. Thus, chiral stationary phase-high performance liquid chromatography (HPLC, IC column, mobile phase: ethyl acetate/n-hexane = 30
:
70) was used to resolve the [2]catenane DPAC-C (Fig. 6a). The area ratio of the two fractions was found to be 27.6
:
27.5, indicating the existence of a pair of stereoisomers of [2]catenane DPAC-C (Fig. 6b and S36–S38†). Each fraction was isolated using a semi-preparative chiral HPLC column, and both the enantiomeric e.e. values were measured to be greater than 99%, indicating the successful separation of [2]catenane enantiomers.
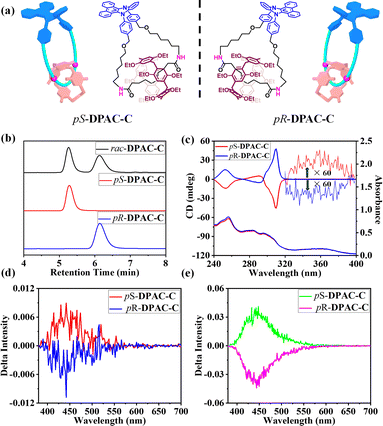 |
| Fig. 6 (a) Cartoon representation and chemical structures of a pair of enantiomers of DPAC-C; (b) chiral HPLC traces (ethyl acetate: n-hexane = 30 : 70, v/v); (c) solution-state ECD spectra and UV/vis absorption spectra (50 μM in THF) of chiral [2]catenanes pS/pR-DPAC-C; delta intensity of chiral [2]catenanes pS/pR-DPAC-C in cast films (d) and in KBr pellets (e). | |
Furthermore, circular dichroism (CD) spectra of these two fractions were then measured, in which mirror-imaged peaks with the same intensity were observed, confirming the existence of enantiomers (Fig. 6c). More importantly, according to the CD spectra, particularly characteristic bands at 250 nm and 310 nm, the absolute configurations of chiral [2]catenanes in the first and second fractions were designated as pS with the DEP[5]A wheel in (pS, pS, pS, pS, pS) conformation and pR with the DEP[5]A wheel in (pR, pR, pR, pR, pR) conformation, respectively. It is worth noting that in the CD spectra, in addition to the peaks attributed to the chiral DEP[5]A wheel, the characteristic absorption peaks ascribed to the DPAC unit were also observed in the 330–400 nm range, indicating the chirality information transfer from DEP[5]A to DPAC in the ground state (Fig. 6c).
It is worth noting that the unique VIE behavior of DPAC provides the possibility for multi-color CPL switching based on chiral [2]catenanes pS/pR-DPAC-C. Unfortunately, although significant color changes were observed after changing the solvent viscosity, no CPL signal was detected (Fig. S39†), which may be due to the lack of efficient chirality information transfer in the excited state in the solution state. Thus, the CPL performances of these chiral [2]catenanes in cast films were then measured. Upon excitation with UV light (λex = 340 nm), both of these chiral [2]catenanes displayed strong CPL emissions at 450 nm that were attributed to the DPAC unit (Fig. 6d, S40a and c†), suggesting an efficient chirality information transmission in the excited state. As expected, the pS/pR-DPAC-C enantiomers revealed almost mirror-imaged CPL peaks with opposite glum, i.e., a positive CPL signal for pS-DPAC-C with a glum value of 2.46 × 10−3 and a negative CPL signal for pR-DPAC-C with a glum value of −2.68 × 10−3, suggesting a pure CPL response. Interestingly, when dispersing these chiral [2]catenanes in the KBr pellet for testing (Fig. 6e, S40b and d†), even higher glum values were observed (pS-DPAC-C, 4.79 × 10−3; pR-DPAC-C, −5.08 × 10−3), which might be attributed to the more orderly arrangements of chiral [2]catenanes in such media. Such impressive CPL performances, together with their tunable VIE behaviors, make these chiral [2]catenanes promising platforms for practical applications.
Conclusions
In summary, aiming at investigating the effects of macrocyclization and catenation on the regulation of VIE behaviors, the typical VIE luminogen DPAC was introduced into a macrocycle and corresponding [2]catenane. By evaluating the VIE behaviors of the resultant DPAC-functionalized macrocycle and [2]catenane in detail with femtosecond transient absorption spectra, the crucial role of macrocyclization and catenation in capturing and stabilizing multiple excited states has been confirmed. In addition, the existence of the DEP[5]A macrocycle with unique planar chirality as the other macrocyclic component endows the DPAC-functionalized [2]catenane with attractive CPL performances, making it the first CPL system based on VIE-active [2]catenane. According to our proof-of-concept study, the mechanical bond shows its great power for the development of novel molecular systems with tunable emission behaviors, thus further enabling the construction of promising platforms for the creation of novel smart chiral luminescent materials.
Data availability
The authors declare that the data supporting the findings of this study are available within the paper and its ESI files.† All relevant data are available from the corresponding author on request.
Author contributions
W. Wang, H.-B. Yang and X.-Q. Wang conceived the project; W.-T. Xu designed and performed the synthetic experiments, VIE and CPL studies with the help of Z. Peng, W.-J. Li and Y. Jiang; P. Wu and J. Chen designed and performed the TA studies; W. Wang, W.-T. Xu and H.-B. Yang prepared the manuscript.
Conflicts of interest
There are no conflicts to declare.
Acknowledgements
W. W. acknowledges the financial support sponsored by the National Natural Science Foundation of China (92356307 and 22001073) and Natural Science Foundation of Shanghai (23ZR1419600). H.-B. Y. acknowledges the financial support sponsored by the National Natural Science Foundation of China (92056203), Science and Technology Commission of Shanghai Municipality (21520710200), and the National Key R&D Program of China (2021YFA1501600). X.-Q. W. acknowledges the financial support sponsored by the National Natural Science Foundation of China (22201077).
References
-
(a) J. Dai, H. Xue, D. Chen, X. Lou, F. Xia and S. Wang, Coord. Chem. Rev., 2022, 464, 214552 CrossRef CAS;
(b) J. Wu, J. Lin and P. Huang, Chem. Soc. Rev., 2023, 52, 3973–3990 RSC;
(c) Y. Huang, L. Ning, X. Zhang, Q. Zhou, Q. Gong and Q. Zhang, Chem. Soc. Rev., 2024, 53, 1090–1166 RSC.
-
(a) X. Liu, D. Huang, C. Lai, G. Zeng, L. Qin, H. Wang, H. Yi, B. Li, S. Liu, M. Zhang, R. Deng, Y. Fu, L. Li, W. Xue and S. Chen, Chem. Soc. Rev., 2019, 48, 5266–5302 RSC;
(b) J.-T. Hou, N. Kwon, S. Wang, B. Wang, X. He, J. Yoon and J. Shen, Coord. Chem. Rev., 2022, 450, 214232 CrossRef CAS;
(c) J. Ouyang, L. Sun, F. Zeng and S. Wu, Coord. Chem. Rev., 2022, 458, 214438 CrossRef CAS;
(d) Z. Wang, Y. Zhou, R. Xu, Y. Xu, D. Dang, Q. Shen, L. Meng and B. Z. Tang, Coord. Chem. Rev., 2022, 451, 214279 CrossRef CAS.
-
(a) O. Ostroverkhova, Chem. Rev., 2016, 116, 13279–13412 CrossRef CAS PubMed;
(b) X. Ai, E. W. Evans, S. Dong, A. J. Gillett, H. Guo, Y. Chen, T. J. H. Hele, R. H. Friend and F. Li, Nature, 2018, 563, 536–540 CrossRef CAS PubMed;
(c) X. Tang, L.-S. Cui, H.-C. Li, A. J. Gillett, F. Auras, Y.-K. Qu, C. Zhong, S. T. E. Jones, Z.-Q. Jiang, R. H. Friend and L.-S. Liao, Nat. Mater., 2020, 19, 1332–1338 CrossRef CAS;
(d) R. Gao, M. S. Kodaimati and D. Yan, Chem. Soc. Rev., 2021, 50, 5564–5589 RSC;
(e) X.-Y. Lou, S. Zhang, Y. Wang and Y.-W. Yang, Chem. Soc. Rev., 2023, 52, 6644–6663 RSC.
-
(a) Z. Zhang, W. Wang, M. O'Hagan, J. Dai, J. Zhang and H. Tian, Angew. Chem., Int. Ed., 2022, 61, e202205758 CrossRef CAS PubMed;
(b) Y.-J. Ma, X. Fang, G. Xiao and D. Yan, Angew. Chem., Int. Ed., 2022, 61, e202114100 CrossRef CAS.
- C. Liu, K. Fu, S. Zheng, L. Yao, W. Zhou and G. Liu, Adv. Opt. Mater., 2023, 11, 2300019 CrossRef CAS.
- C. Ma, W. Sun, L. Xu, Y. Qian, J. Dai, G. Zhong, Y. Hou, J. Liu and B. Shen, J. Mater. Chem. B, 2020, 8, 9642–9651 RSC.
- Q. Zhang, L. Yang, Y. Han, Z. Wang, H. Li, S. Sun and Y. Xu, Chem. Eng. J., 2022, 428, 130986 CrossRef CAS.
- C. Shang, G. Wang, K. Liu, Q. Jiang, F. Liu, P.-T. Chou and Y. Fang, Angew. Chem., Int. Ed., 2020, 59, 8579–8585 CrossRef CAS PubMed.
-
(a) Z. Fu, K. Wang and B. Zou, Chin. Chem. Lett., 2019, 30, 1883–1894 CrossRef CAS;
(b) C. Wang, Y. Yu, Y. Yuan, C. Ren, Q. Liao, J. Wang, Z. Chai, Q. Li and Z. Li, Matter, 2020, 2, 181–193 CrossRef.
-
(a) D. Tu, P. Leong, S. Guo, H. Yan, C. Lu and Q. Zhao, Angew. Chem., Int. Ed., 2017, 56, 11370–11374 CrossRef CAS;
(b) K. Tabata, T. Yamada, H. Kita and Y. Yamamoto, Adv. Funct. Mater., 2019, 29, 1805824 CrossRef;
(c) Z. Zhang, Y.-A. Chen, W.-Y. Hung, W.-F. Tang, Y.-H. Hsu, C.-L. Chen, F.-Y. Meng and P.-T. Chou, Chem. Mater., 2016, 28, 8815–8824 CrossRef CAS;
(d) J. X. Ong, C. S. Q. Lim, H. V. Le and W. H. Ang, Angew. Chem., Int. Ed., 2019, 58, 164–167 CrossRef CAS PubMed;
(e) A. C. Sedgwick, W.-T. Dou, J.-B. Jiao, L. Wu, G. T. Williams, A. T. A. Jenkins, S. D. Bull, J. L. Sessler, X.-P. He and T. D. James, J. Am. Chem. Soc., 2018, 140, 14267–14271 CrossRef CAS PubMed;
(f) J. Zhang, P. Alam, S. Zhang, H. Shen, L. Hu, H. H. Y. Sung, I. D. Williams, J. Sun, J. W. Y. Lam, H. Zhang and B. Z. Tang, Nat. Commun., 2022, 13, 3492 CrossRef CAS PubMed.
-
(a) Z. Zhang, Y.-S. Wu, K.-C. Tang, C.-L. Chen, J.-W. Ho, J. Su, H. Tian and P.-T. Chou, J. Am. Chem. Soc., 2015, 137, 8509–8520 CrossRef CAS PubMed;
(b) W. Huang, L. Sun, Z. Zheng, J. Su and H. Tian, Chem. Commun., 2015, 51, 4462–4464 RSC;
(c) Z. Zhang, G. Sun, W. Chen, J. Su and H. Tian, Chem. Sci., 2020, 11, 7525–7537 RSC;
(d) Z. Zhang, W. Song, J. Su and H. Tian, Adv. Funct. Mater., 2020, 30, 1902803 CrossRef CAS;
(e) Z. Zhang, X. Jin, X. Sun, J. Su and D.-H. Qu, Coord. Chem. Rev., 2022, 472, 214768 CrossRef CAS;
(f) S. Qiu, Z. Zhang, Z. Wang, D.-H. Qu and H. Tian, Precis. Chem., 2023, 1, 129–138 CrossRef CAS;
(g) S. Qiu, Z. Zhang, Y. Wu, F. Tong, K. Chen, G. Liu, L. Zhang, Z. Wang, D.-H. Qu and H. Tian, CCS Chem., 2022, 4, 2344–2353 CrossRef CAS.
-
(a) H. Zhou, J. Mei, Y.-A. Chen, C.-L. Chen, W. Chen, Z. Zhang, J. Su, P.-T. Chou and H. Tian, Small, 2016, 12, 6542–6546 CrossRef CAS PubMed;
(b) W. Chen, C. Guo, Q. He, X. Chi, V. M. Lynch, Z. Zhang, J. Su, H. Tian and J. L. Sessler, J. Am. Chem. Soc., 2019, 141, 14798–14806 CrossRef CAS PubMed;
(c) Z. Zong, Q. Zhang and D.-H. Qu, CCS Chem., 2024, 6, 774–782 CrossRef CAS.
-
(a) L. Shi, W. Song, C. Lian, W. Chen, J. Mei, J. Su, H. Liu and H. Tian, Adv. Opt. Mater., 2018, 6, 1800190 CrossRef;
(b) Y. Zhang, Y. Li, H. Wang, Z. Zhang, Y. Feng, Q. Tian, N. Li, J. Mei, J. Su and H. Tian, ACS Appl. Mater. Interfaces, 2019, 11, 39351–39358 CrossRef CAS PubMed;
(c) W. Song, W. Ye, L. Shi, J. Huang, Z. Zhang, J. Mei, J. Su and H. Tian, Mater. Horiz., 2020, 7, 615–623 RSC;
(d) Y. Su, H. Liu, X. Chen, Q. Wang, J. Su and Z. Zhang, ACS Appl. Polym. Mater., 2022, 4, 1636–1642 CrossRef CAS;
(e) F. Gu, Y. Li, T. Jiang, J. Su and X. Ma, CCS Chem., 2022, 4, 3014–3022 CrossRef CAS.
-
(a) Q. Gong, W. Qin, P. Xiao, X. Wu, L. Li, G. Zhang, R. Zhang, J. Sun, S. Q. Yao and W. Huang, Chem. Commun., 2020, 56, 58–61 RSC;
(b) J. Ramos-Soriano, S. J. Benitez-Benitez, A. P. Davis and M. C. Galan, Angew. Chem., Int. Ed., 2021, 60, 16880–16884 CrossRef CAS PubMed;
(c) W.-T. Dou, X. Wang, T. Liu, S. Zhao, J.-J. Liu, Y. Yan, J. Li, C.-Y. Zhang, A. C. Sedgwick, H. Tian, J. L. Sessler, D.-M. Zhou and X.-P. He, Chem, 2022, 8, 1750–1761 CrossRef CAS.
-
(a) J. Wang, X. Yao, Y. Liu, H. Zhou, W. Chen, G. Sun, J. Su, X. Ma and H. Tian, Adv. Opt. Mater., 2018, 6, 1800074 CrossRef;
(b) G. Sun, J. Pan, Y. Wu, Y. Liu, W. Chen, Z. Zhang and J. Su, ACS Appl. Mater. Interfaces, 2020, 12, 10875–10882 CrossRef CAS PubMed;
(c) S. Liu, J. Wang, F. Tang, N. Wang, L. Li, C. Yao and L. Li, ACS Appl. Mater. Interfaces, 2020, 12, 55269–55277 CrossRef CAS PubMed;
(d) X. Chen, J. Chen, G. Sun, L. Guo, J. Su and Z. Zhang, ACS Appl. Mater. Interfaces, 2021, 13, 38629–38636 CrossRef CAS PubMed;
(e) Y. Hu, Z. Huang, I. Willner and X. Ma, CCS Chem., 2024, 6, 518–527 CrossRef.
-
(a) Z. Zong, Q. Zhang, S.-H. Qiu, Q. Wang, C. Zhao, C.-X. Zhao, H. Tian and D.-H. Qu, Angew. Chem., Int. Ed., 2022, 61, e202116414 CrossRef CAS PubMed;
(b) Z. Zhou, D.-G. Chen, M. L. Saha, H. Wang, X. Li, P.-T. Chou and P. J. Stang, J. Am. Chem. Soc., 2019, 141, 5535–5543 CrossRef CAS PubMed;
(c) W. Chen, C.-L. Chen, Z. Zhang, Y.-A. Chen, W.-C. Chao, J. Su, H. Tian and P.-T. Chou, J. Am. Chem. Soc., 2017, 139, 1636–1644 CrossRef CAS PubMed;
(d) S. Qiu, Y. Zhao, L. Zhang, Y. Ni, Y. Wu, H. Cong, D.-H. Qu, W. Jiang, J. Wu, H. Tian and Z. Wang, CCS Chem., 2023, 5, 1763–1772 CrossRef CAS.
-
(a) J. F. Stoddart, Chem. Soc. Rev., 2009, 38, 1802 RSC;
(b) R. S. Forgan, J. P. Sauvage and J. F. Stoddart, Chem. Rev., 2011, 111, 5434–5464 CrossRef CAS PubMed;
(c) E. A. Neal and S. M. Goldup, Chem. Commun., 2014, 50, 5128–5142 RSC;
(d)
C. J. Bruns and J. F. Stoddart, The Nature of the Mechanical Bond: From Molecules to Machines, John Wiley and Sons, Hoboken, 2016 CrossRef;
(e) J. E. M. Lewis, M. Galli and S. M. Goldup, Chem. Commun., 2017, 53, 298–312 RSC;
(f) M. Denis and S. M. Goldup, Nat. Rev. Chem, 2017, 1, 0061 CrossRef CAS;
(g) H. Y. Zhou, Y. Han and C. F. Chen, Mater. Chem. Front., 2020, 4, 12–28 RSC;
(h) D. Sluysmans and J. F. Stoddart, Trends Chem., 2019, 1, 185 CrossRef CAS;
(i) J.-X. Liu, K. Chen and C. Redshaw, Chem. Soc. Rev., 2023, 52, 1428–1455 RSC;
(j) A. H. G. David and J. F. Stoddart, Isr. J. Chem., 2021, 61, 608–621 CrossRef CAS.
-
(a) S. Yang, C.-X. Zhao, S. Crespi, X. Li, Q. Zhang, Z.-Y. Zhang, J. Mei, H. Tian and D.-H. Qu, Chem, 2021, 7, 1544–1556 CrossRef CAS;
(b) C. Yu, X. Wang, C.-X. Zhao, S. Yang, J. Gan, Z. Wang, Z. Cao and D.-H. Qu, Chin. Chem. Lett., 2022, 33, 4904–4907 CrossRef CAS;
(c) P. Rajamalli, F. Rizzi, W. Li, M. A. Jinks, A. K. Gupta, B. A. Laidlaw, I. D. W. Samuel, T. J. Penfold, S. M. Goldup and E. Zysman-Colman, Angew. Chem., Int. Ed., 2021, 60, 12066–12073 CrossRef CAS PubMed.
-
(a) W. Wang, L.-J. Chen, X.-Q. Wang, B. Sun, X. Li, Y. Zhang, J. Shi, Y. Yu, L. Zhang, M. Liu and H.-B. Yang, Proc. Natl. Acad. Sci. U. S. A., 2015, 112, 5597–5601 CrossRef CAS PubMed;
(b) X.-Q. Wang, W. Wang, W.-J. Li, L.-J. Chen, R. Yao, G.-Q. Yin, Y.-X. Wang, Y. Zhang, J. Huang, H. Tan, Y. Yu, X. Li, L. Xu and H.-B. Yang, Nat. Commun., 2018, 9, 3190 CrossRef PubMed;
(c) X.-Q. Wang, W.-J. Li, W. Wang, J. Wen, Y. Zhang, H. Tan and H.-B. Yang, J. Am. Chem. Soc., 2019, 141, 13923–13930 CrossRef CAS PubMed;
(d) W.-J. Li, Z. Hu, L. Xu, X.-Q. Wang, W. Wang, G.-Q. Yin, D.-Y. Zhang, Z. Sun, X. Li, H. Sun and H.-B. Yang, J. Am. Chem. Soc., 2020, 142, 16748–16756 CrossRef CAS PubMed;
(e) W.-J. Li, W. Wang, X.-Q. Wang, M. Li, Y. Ke, R. Yao, J. Wen, G.-Q. Yin, B. Jiang, X. Li, P. Yin and H.-B. Yang, J. Am. Chem. Soc., 2020, 142, 8473–8482 CrossRef CAS PubMed;
(f) W.-J. Li, X.-Q. Wang, D.-Y. Zhang, Y.-X. Hu, W.-T. Xu, L. Xu, W. Wang and H.-B. Yang, Angew. Chem., Int. Ed., 2021, 60, 18761–18768 CrossRef CAS PubMed;
(g) W.-J. Li, W.-T. Xu, X.-Q. Wang, Y. Jiang, Y. Zhu, D.-Y. Zhang, X.-Q. Xu, L.-R. Hu, W. Wang and H.-B. Yang, J. Am. Chem. Soc., 2023, 145, 14498–14509 CrossRef CAS PubMed;
(h) X.-Q. Wang, W.-J. Li, W. Wang and H.-B. Yang, Acc. Chem. Res., 2021, 54, 4091–4106 CrossRef CAS PubMed;
(i) Y. Zhu, H. Jiang, W. Wu, X.-Q. Xu, X.-Q. Wang, W.-J. Li, W.-T. Xu, G. Liu, Y. Ke, W. Wang and H.-B. Yang, Nat. Commun., 2023, 14, 5307 CrossRef CAS PubMed;
(j) D.-Y. Zhang, Y. Sang, T. K. Das, Z. Guan, N. Zhong, C.-G. Duan, W. Wang, J. Fransson, R. Naaman and H.-B. Yang, J. Am. Chem. Soc., 2023, 145, 26791–26798 CrossRef CAS PubMed.
-
(a) W.-J. Li, Q. Gu, X.-Q. Wang, D.-Y. Zhang, Y.-T. Wang, X. He, W. Wang and H.-B. Yang, Angew. Chem., Int. Ed., 2021, 60, 9507–9515 CrossRef CAS PubMed;
(b) Y. Wang, J. Gong, X. Wang, W.-J. Li, X.-Q. Wang, X. He, W. Wang and H.-B. Yang, Angew. Chem., Int. Ed., 2022, 61, e202210542 CrossRef CAS PubMed.
-
(a) S. Fa, T. Kakuta, T.-a. Yamagishi and T. Ogoshi, Chem. Lett., 2019, 48, 1278–1287 CrossRef CAS;
(b) J.-F. Chen, J.-D. Ding and T.-B. Wei, Chem. Commun., 2021, 57, 9029–9039 RSC;
(c) C. Shi, H. Li, X. Shi, L. Zhao and H. Qiu, Chin. Chem. Lett., 2022, 33, 3613–3622 CrossRef CAS;
(d) T. Zhao, W. Wu and C. Yang, Chem. Commun., 2023, 59, 11469–11483 RSC;
(e) K. Kato, S. Fa and T. Ogoshi, Angew. Chem., Int. Ed., 2023, 62, e202308316 CrossRef CAS PubMed;
(f) K. Kato, S. Fa, S. Ohtani, T.-h. Shi, A. M. Brouwer and T. Ogoshi, Chem. Soc. Rev., 2022, 51, 3648–3687 RSC.
-
(a) I. Nierengarten, E. Meichsner, M. Holler, P. Pieper, R. Deschenaux, B. Delavaux-Nicot and J.-F. Nierengarten, Chem.–Eur. J., 2018, 24, 169–177 CrossRef CAS PubMed;
(b) M. Rémy, I. Nierengarten, B. Park, M. Holler, U. Hahn and J.-F. Nierengarten, Chem.–Eur. J., 2021, 27, 8492–8499 CrossRef PubMed;
(c) N. Becharguia, E. Wasielewski, R. Abidi, I. Nierengarten and J.-F. Nierengarten, Chem.–Eur. J., 2024, 30, e202303501 CrossRef CAS PubMed;
(d) N. Becharguia, I. Nierengarten, J.-M. Strub, S. Cianférani, M. Rémy, E. Wasielewski, R. Abidi and J.-F. Nierengarten, Chem.–Eur. J., 2024, e202304131 CrossRef CAS PubMed;
(e) Z. Peng, P.-P. Jia, X.-Q. Wang, X.-L. Zhao, H.-B. Yang and W. Wang, CCS Chem., 2024 DOI:10.31635/ccschem.024.202303738.
-
(a) W.-T. Xu, X. Li, P. Wu, W.-J. Li, Y. Wang, X.-Q. Xu, X.-Q. Wang, J. Chen, H.-B. Yang and W. Wang, Angew. Chem., Int. Ed., 2024, 63, e202319502 CrossRef CAS PubMed;
(b) X. Jin, S. Guo, X. Wang, M. Cong, J. Chen, Z. Zhang, J. Su, D.-H. Qu and H. Tian, Angew. Chem., Int. Ed., 2023, 62, e202305572 CrossRef CAS PubMed.
- J. J. Snellenburg, S. P. Laptenok, R. Seger, K. M. Mullen and I. H. M. van Stokkum, J. Stat. Software, 2012, 49, 1–22 Search PubMed.
|
This journal is © The Royal Society of Chemistry 2024 |
Click here to see how this site uses Cookies. View our privacy policy here.