DOI:
10.1039/D4SC01891E
(Review Article)
Chem. Sci., 2024,
15, 9026-9046
Photocatalytic NOx removal and recovery: progress, challenges and future perspectives
Received
21st March 2024
, Accepted 18th May 2024
First published on 20th May 2024
Abstract
The excessive production of nitrogen oxides (NOx) from energy production, agricultural activities, transportation, and other human activities remains a pressing issue in atmospheric environment management. NOx serves both as a significant pollutant and a potential feedstock for energy carriers. Photocatalytic technology for NOx removal and recovery has received widespread attention and has experienced rapid development in recent years owing to its environmental friendliness, mild reaction conditions, and high efficiency. This review systematically summarizes the recent advances in photocatalytic removal, encompassing NOx oxidation removal (including single and synergistic removal and NO3− decomposition), NOx reduction to N2, and the emergent NOx upcycling into green ammonia. Special focus is given to the molecular understanding of the interfacial nitrogen-associated reaction mechanisms and their regulation pathways. Finally, the status and the challenges of photocatalytic NOx removal and recovery are critically discussed and future outlooks are proposed for their potential practical application.
1 Introduction
Nitrogen oxides (NOx, consisting of 95% NO and NO2) are pervasive air pollutants which are implicated in various environmental events (acid rain, photochemical smog, and global warming) and cause detrimental impacts on public health.1–3 They serve as key precursors for the formation of emerging tropospheric ozone, PM2.5 as well as secondary organic aerosol pollution.2,4 Although there are NOx from natural sources, anthropogenic activities, particularly fossil fuel combustion, account for a major contribution to the global NOx emissions in the atmosphere.5,6
While NOx is a pollutant, it can also be recognized as an energy feedstock. With a high reactivity, NOx possesses the potential to be utilized for the synthesis of valuable N-containing chemicals. Ammonia (NH3) is indeed an indispensable chemical for fertilizers,7 dyes,8 polymers,9 explosives,10 resins,10etc., and can serve as a carbon-neutral energy carrier.11 The industrial production of NH3 synthesis currently heavily relies on the Haber–Bosch route, which requires harsh conditions (400–500 °C, 150–300 atm), resulting in more than 2% of global energy consumption.12,13 This high energy consumption is also largely derived from fossil fuels.14 Faced with global energy shortages, recovery of NOx to NH3 gives an alternative route for both environmental control and nitrogen resource utilization.
Photocatalytic technology powered by renewable solar energy has been reckoned as one of the most viable strategies that could convert NOx into less harmful or useful products under mild conditions.15–17 At present, conventional photocatalytic technologies, including NOx oxidation, NOx decomposition, and selective NOx reduction, have been vigorously developed, spanning from material design to elucidation of the underlying mechanisms.6,18–20 Meanwhile, the tendency of photocatalytic removal of NOx has been gradually transformed from oxidation, purification, and then to resource utilization. Particularly, the photocatalytic NOx reduction for ammonia synthesis has achieved milestone progress in recent years.
The review aims to summarize recent advancements in photocatalytic NOx removal and recovery. The cutting-edge developments in photocatalytic removal in terms of NOx oxidation, NOx reduction to nitrogen, and NOx upcycling to ammonia are comprehensively reviewed. We also highlighted the one-step (NO → NH3) and two-step (NO → NO3− → NH3) methods as new ideas for simultaneous NOx removal and resource utilization. Finally, we discuss the current challenges and provide some perspectives on future directions for NOx removal and recovery. The illustration of this review on NOx removal and recovery is shown in Scheme 1. We hope that this review will inspire significant research and essential advancements in the field of photocatalytic NOx removal.
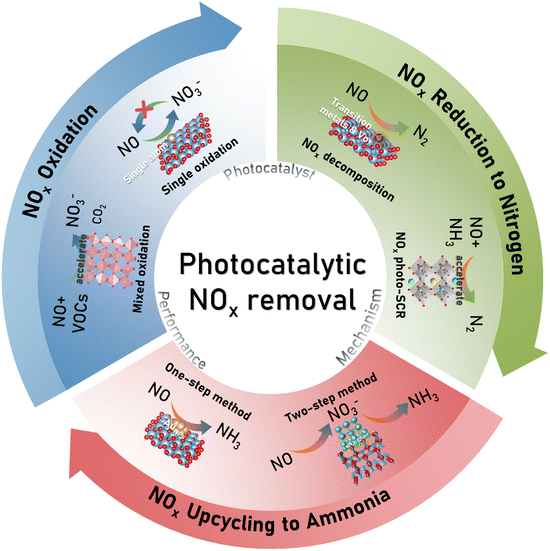 |
| Scheme 1 The NOx removal and recovery by different photocatalytic approaches. | |
2 NOx oxidation
2.1 Single NOx oxidation
2.1.1 NOx oxidation mechanisms.
Photocatalytic oxidation removal is mainly applied to low-concentration pollutant purification. During the photocatalytic reaction process, electron–hole pairs are generated in the catalyst under light irradiation, initiating the oxidation reactions. These pairs migrate to the surface of the photocatalysts and react with adsorbed H2O and O2 molecules, leading to the generation of reactive oxygen species (ROS) such as ˙OH, ˙O2−, and 1O2. These ROS then participate in a series of oxidation reactions with NO, ultimately forming the final product NO3−. Detailed reaction pathways and intermediates involved in this photocatalytic NO oxidation process can be described using specific equations, which are supported by relevant ref. 21 and 22: | Photocatalysts + hν → h+ + e− | (1) |
| HNO2 + ˙OH → NO2 + H2O | (4) |
2.1.2 Materials for NOx oxidation.
There are three main factors affecting the catalytic performance of photocatalytic oxidation: light absorption, charge carrier separation and transfer, and active site construction.23 Based on these factors, various modification methods were conducted on TiO2-based materials,24–27 Bi-based materials,28–30 g-C3N4-based materials,31,32 and perovskite-type materials.33 Modification methods include but are not limited to metal/non-metal doping, defects, heterojunctions, and sensitization.34
Single-atom catalysts have attracted much attention in the field of materials due to their unique activity and effective utilization of active atoms. Several studies have confirmed that single atoms can improve the performance of photocatalysts for NOx removal and provide a convenient way to regulate the generation of ROS for effective pollutant elimination. Liu et al.35 achieved the stabilization of Pt on carbon-defective g-C3N4 by leveraging its affinity with nitrogen atoms resulting from carbon vacancies. The resulting Pd-Cv-CN catalyst enhances charge transfer efficiency, generating sufficient photoelectrons for the formation of ˙OH and ˙O2− species. This, in turn, facilitates the removal of NO and enhances the selectivity towards NO3−. Hu et al.36 discovered that single Pt atom bridged within a metal–organic framework (MOF)-derived ZnO/C via carbon atoms (Pt–ZnO/C) exhibits enhanced adsorption energy and charge transfer characteristics for O2 and H2O (Fig. 1a and b). In contrast to ˙OH, ˙O2− plays a pivotal role in the conversion of NOx to NO3− on a single atom site at the catalytic surface (Fig. 1c). Zhang et al.37 incorporated Zn atoms into the interlayer of g-C3N4, forming the ZnN3 structure by linking zinc with three nitrogen atoms. The presence of a single zinc atom facilitated the formation of a Zn–O2–NO structure upon adsorption of O2 and NO, thereby promoting the dissociation of O2 and the direct conversion of NO to nitrate (Fig. 1d). Simultaneously, the formation of the toxic byproduct NO2 was inhibited. A similar result enhancement was observed upon doping TiO2 hollow microspheres (TiO2-HMSs) with single atomic Fe.38 Particularly noteworthy is the effect of silver atoms loaded onto BiOCl (001) facet-exposed nanosheets, which constructed triangular Cl–Ag1–Cl sites on Cl-terminated BiOCl. These sites selectively activated molecular oxygen to ˙O2− and enhanced nitrate adsorption by altering the coordination mode of nitrate on the catalyst, thereby preventing nitrate decomposition (Fig. 1e and f).39 The NOx removal efficiency of Ag1/BiOCl reached up to 90%, with 97% selectivity for NO3− and minimal emission of NO2 (Fig. 1g–i).
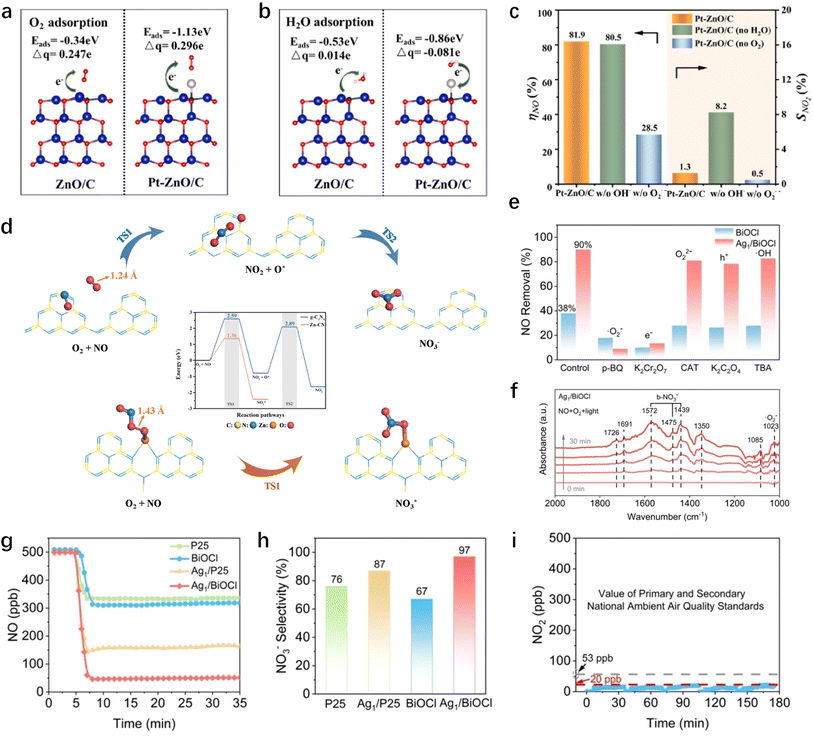 |
| Fig. 1 DFT calculation of (a) O2 adsorption and (b) H2O adsorption on ZnO/C and Pt–ZnO/C surfaces. (c) The NO conversion rate and selectivity of NO2 formation over the Pt–ZnO/C catalyst in the presence and absence of moisture and O2.36 (d) NO oxidation process over g-C3N4 and Zn–CN.37 (e) Photocatalytic NO removal over Ag1/BiOCl using different scavengers. (f) DRIFTS spectra of Ag1/BiOCl for NO oxidation. (g) NO oxidation of P25, BiOCl, Ag1/P25 and Ag1/BiOCl. (h) NO3− selectivity of Ag1/P25 and Ag1/BiOCl under visible light irradiation. (i) The amount of NO2 generation.39 | |
In summary, the introduction of single atoms can significantly enhance the adsorption of O2 and H2O molecules and the generation of ˙O2− and ˙OH, thereby improving both the performance and nitrate selectivity of the photocatalyst. This provides a facile pathway to manipulate the ROS generation for efficient and selective pollutant removal.
2.1.3 Machine learning as an analysis tool for NOx oxidation.
It is well-known that the performance of NOx removal not only depends on catalyst properties but also closely relies on preparation methods and reaction conditions. Although catalyst design and optimization of experimental conditions have been fully explored, the dominant factors affecting the NOx removal rate remain unclear. Machine learning (ML) has achieved remarkable progress over the past few decades and has become the most potent tool in the field of data mining and analytics.40,41 ML methods leverage algorithms to extract insights from vast, intricate, and multidimensional datasets, enabling rapid and precise predictions. Recently, progress has been made in employing ML methods in the photocatalytic NOx oxidation process. Li et al.42 achieved success in predicting the NO removal rate in the photocatalytic purification of g-C3N4 catalysts using ML methods like gradient boosting decision trees, eXtreme gradient boosting, and random forests. Their findings indicate that catalyst characteristics, reaction process, and preparation conditions are the primary empirical categories influencing the NO removal rate. They have also unveiled the intricate relationships between the photocatalytic NO removal rate and various influencing factors. This approach, to some extent, mitigates the downsides of traditional experimental work, including high costs, lengthy timelines, and demanding labor.
2.1.4 NO3− decomposition on the photocatalyst.
The formation of nitrate is a key process of NOx oxidation, as nitrate has been considered a permanent sink of NOx.43 However, it undergoes photolysis processes under light irradiation (also called detoxification), especially on the surface of the photocatalyst, which in turn serves as a source of atmospheric nitrogenated compounds.44–49 TiO2, the predominant photocatalyst,50,51 has been shown to promote nitrate photolysis processes through photochemical interaction.44 Therefore, exploring the decomposition mechanism of nitrate on the surface of photocatalysts assumes pivotal significance for stabilizing nitrate on the catalyst surface. The enhanced photolysis of nitrate by TiO2 under UV irradiation can be explained using the following reactions52–54 (eqn (10)–(19)): | 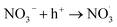 | (11) |
|  | (12) |
|  | (13) |
| NO2 + hν → NO + O(3P) | (14) |
| NO2 + 2e− + 2H+ → NO + H2O | (16) |
| 2NO + 2e− + 2H+ → N2O + H2O | (17) |
| NO2− + H2O → HONO + HO˙ | (18) |
There are two main factors affecting the nitrate decomposition mechanism on the TiO2, which are co-adsorbed cations and the coexisting atmosphere. It is suggested that the cations on TiO2 could inhibit the decomposition of nitrates. The presence of cations enhanced the adsorption of NO3− by forming ion pairs with NO3− and prevented NO3− from binding with light-induced h+ to generate ˙NO3.55,56 The decomposition of nitrates is facilitated by co-existing pollutants in the surrounding environment such as SO2 and volatile organic compounds (VOCs). SO2 preferentially reacts with ˙NO3 radicals rather than photoelectrons, resulting in a significant improvement in nitrate decomposition.57,58 Similar to SO2, formaldehyde (HCHO) participated in the transformation of NO3 to HNO3 through hydrogen abstraction to promote nitrate decomposition. HCHO converts NO3− on particle surfaces into HNO3 by reacting with ˙NO3, and then HNO3 photolyzes at a faster rate.55 Interestingly, NO produced from nitrate decomposition may be re-adsorbed on the surface of TiO2 to promote nitrate decomposition.59 The N–O bond of NO3− could be activated by NO molecules. Subsequently, photogenerated electrons, captured by NO, facilitate the transformation of NO3− under light irradiation through the NO3− + NO− → 2NO2− pathway.59 It is proposed that addressing the photochemical transformation of surface NO3− may entail suppressing the formation of NO− during the photocatalytic NO oxidation process.
Although the well-known photochemical activity of nitrate decomposition is excited around 300 nm,60 it has been verified that nitrate decomposition could be induced by visible light (λ > 380 nm) on TiO2. Wang et al.47 investigated the decomposition of surface NO3− on nmTiO2 under visible light exposure. By regulating the reaction atmosphere (dry argon, wet argon, and wet air), distinct production of NO and NO2 is observed (Fig. 2a–c). Through meticulous control of in situ DRIFTS experiments, they uncovered different decomposition pathways stemming from different surface coordination modes of the NO3− (Fig. 2d and e). Nitrates generated on photocatalysts exhibit two coordination modes: monodentate nitrate (m-NO3−) and bidentate nitrate (b-NO3−). The m-NO3− decomposition initiated by photo-induced electrons primarily leads to the production of NO and NO2. It is indicated that H2O and O2 have a remarkable influence on the decomposition products of NO3−. The introduction of H2O molecules results in dissociation on nmTiO2, forming surface hydroxyl groups, which then facilitate the conversion of b-NO3− to m-NO3− through hydrogen bonding interactions.47 Subsequently, visible-light-driven photocatalytic decomposition led to an enhancement in the concentration of NOx. Although O2 can promote nitrate regeneration through the oxidation process, the effect of H2O on NO3− decomposition could not be restrained in the presence of O2.
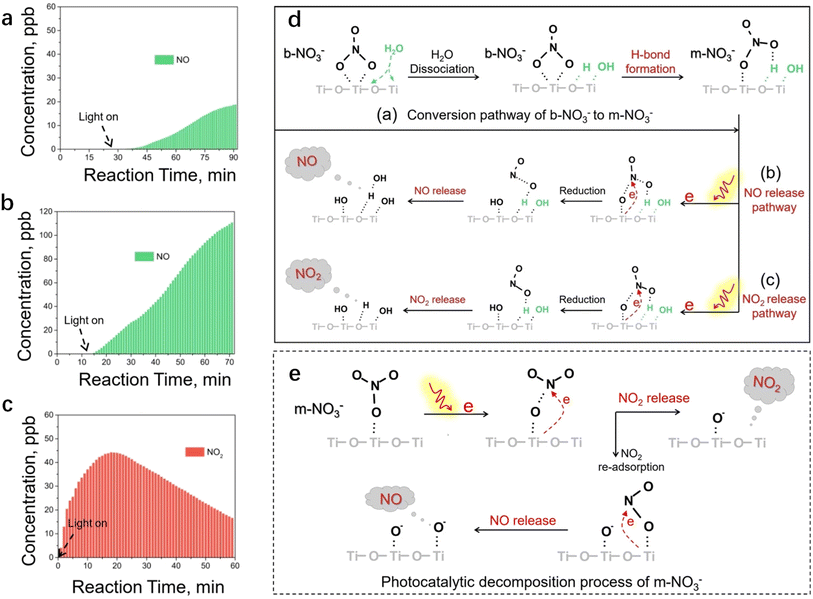 |
| Fig. 2 Generation of gas-phase products in (a) dry argon atmosphere, (b) humidity argon atmosphere, and (c) humidity air atmosphere under visible light irradiation. (d) Proposed decomposition mechanism of b-NO3− triggered by visible light photocatalysis: (a) conversion pathway of b-NO3− to m-NO3− under visible light irradiation. (b) Photocatalytic decomposition pathway of produced m-NO3− to NO. (c) Photocatalytic decomposition pathway of produced m-NO3− to NO2. (e) Proposed decomposition mechanism of m-NO3−.47 | |
To sum up, the decomposition of nitrate on the surface of active particulate matter TiO2 under various conditions has been confirmed. Therefore, when exploring the mechanism of oxidative NOx removal, it is essential to consider the nitrate decomposition process. This perspective offers valuable insights for a more comprehensive understanding of the photocatalytic reaction.
2.2 Synergistic NOx oxidation
In the real scenario, NOx is typically present alongside other air pollutants. The interaction or combination of NOx with other pollutants, such as volatile organic compounds (VOCs) and sulfur dioxide (SO2), can lead to the formation of more severe secondary pollutants, especially under sunlight irradiation.61–65 In addition to designing effective photocatalysts, it is crucial to address the complexities arising from these multiple pollutants when implementing photocatalytic NO oxidation technology in real reactors at pilot or larger scales.66
2.2.1 Synergistic effect between NOx and VOCs.
It has been revealed that there is a certain synergistic effect between NOx and VOCs on the specific photocatalysts to inhibit the generation of by-products. Xue et al.67 proposed an enhanced photocatalytic NO removal through the addition of acetaldehyde to Sr2Sb2O7 to prevent secondary peroxyacetyl nitrate (PAN) formation. The intermediates NO2− from NO and ˙CH3 from acetaldehyde tend to bond and further oxidize to CH3ONO2, thus promoting NO removal. Density functional theory (DFT) calculations show that the Gibbs free energy required for the conversion of NO to NO3− in the mixed degradation process is lower compared to that in the individual removal pathways, thereby favoring the deep oxidation to NO3−. Li et al.68 investigated the simultaneous degradation of NO and C7H8 (toluene) mixed pollutants. On the In(OH)3 photocatalyst, NO and toluene exhibit coupling reaction effects and give rise to a new NO conversion pathway, leading to NO deep oxidation to NO3− (Fig. 3a and b). The key intermediate C7H7NOH is favorable for the NO oxidation to NO2 to inhibit O3 formation (Fig. 3c and d). Then NO2 was inclined to bond with toluene to form C7H6NO2. The incorporation of the nitro group alters the charge distribution within the benzene ring, disrupting the typical distribution of electrons within the π–π stacked structure (Fig. 3e). The rearrangement of electrons opens the benzene ring more readily than toluene, allowing further NO3− formation. This synergistic effect of NO and toluene also occurs in InOOH. The increase in humidity could suppress catalyst deactivation and improve the conversion of mixed pollutants, as shown in Fig. 3f.69 The yield of nitrate is also consistent with the conversion efficiency (Fig. 3g). This finding indicates that the extensive occupation of the catalyst surface by nitrate serves as a deactivation factor in mixed pollutant reactions.69 Under high humidity conditions, H2O and NO3− indicate similar adsorption configurations on InOOH, but H2O generates more free radicals to directly enhance the conversion of C–N intermediates, thus promoting the conversion of NO to NO3− (Fig. 3h and i).69 It was also proposed that NO could prevent the deactivation of the photocatalyst due to the incomplete oxidation product NO2 in the process of o-xylene degradation.70 NO2 ensures the generation of a sufficient amount of TiO2 (˙OL) radicals, allowing for the complete mineralization of o-xylene and inhibiting the deactivation of TiO2.70
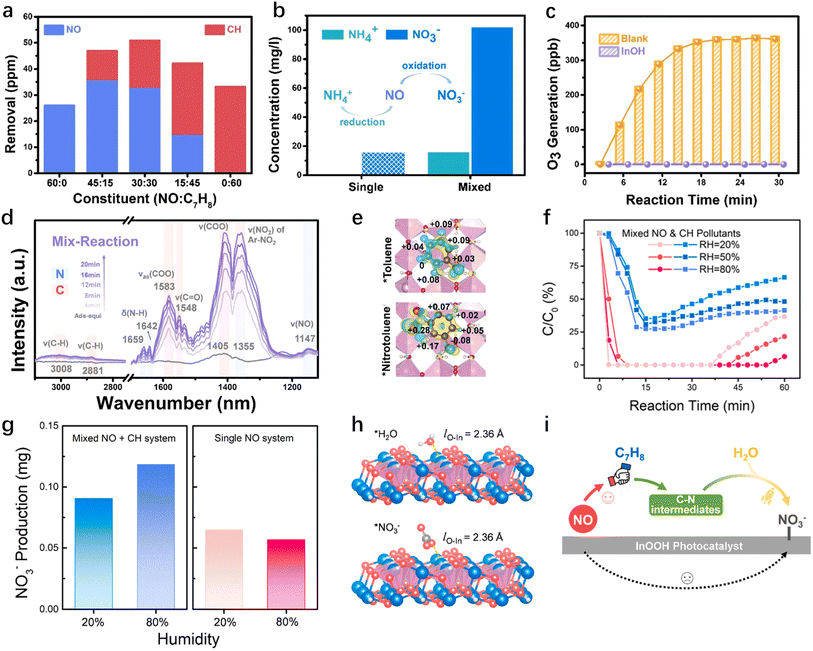 |
| Fig. 3 (a) The removal of NO and C7H8 at different mixing ratios. (b) The nitrogen-containing products identified by IC. (c) The generation of O3 without and with the photocatalyst under light irradiation. (d) In situ DRIFTS spectra for the photocatalytic reaction of NO and C7H8.68 (e) Charge distribution of C7H8 and C7H7NO2 on the In(OH)3 photocatalyst; blue and yellow clouds represent charge depletion and accumulation, and the isosurface level is set to 0.0007 eV Å−3. (f) Conversion of mixed pollutants at different relative humidities. [NO] = [C7H8] = 30 ppm. (g) NO3− production in mixed and single systems at different relative humidities. (h) Simulation of reactant and product binding on the catalyst. (i) Illustration of the synergistic interaction between NO and C7H8 and the promoting effect of H2O.69 | |
2.2.2 Inhibition effect between NOx and SO2.
In contrast to VOCs, research indicates that SO2 tends to hinder the removal of NOx. According to the studies of Ao et al.,71 the presence of SO2 resulted in an 8% decrease in NO conversion and a 10% increase in NO2 generation. The formation of sulfate ions on the catalyst surface obstructed the adsorption sites of TiO2 for converting NO2 to HNO3, consequently increasing the exit concentration of NO2. Chen et al.66 further validated this deactivation mechanism through DFT calculations. The result showed that surface hydroxyls on the (101) facet of TiO2 create unsaturated coordination of adjacent Ti or O atoms, promoting the adsorption of NO and SO2. However, there is a competitive adsorption between NO and SO2, as evidenced by the decreasing adsorption energy and charge density. In the oxidation process, SO2 significantly competes with NO for reaction with ˙O2−. More valence electrons are involved in the oxidation of SO2, resulting in higher binding energy between *O–SOOO* than *O–NOO*.
3 NOx reduction to nitrogen
Contemporary research in photocatalytic NO abatement predominantly centers on oxidizing NO to NO3−. Nevertheless, concerns arise from the production of the more toxic by-product, NO2, catalyst deactivation resulting from the obstruction of reaction sites by nitrate products, and the denitrification of NO3−. These challenges impede the practical application of photocatalysts. An alternative approach for NOx removal involves the reduction of NO to N2 without causing deactivation or secondary pollution. This can be achieved through two approaches: direct reduction of NO to N2 and O2 under oxygen-free conditions (NOx decomposition), and selective reduction of NO to N2 in a reducing atmosphere (NOx photo-selective reduction).
3.1 NOx decomposition
The decomposition of NO into harmless N2 and O2 represents one of the most desirable pathways of NO removal, as it eliminates the need for additional reductants. Transition metal oxides have emerged as typical photocatalysts for NOx decomposition.72–82 Utilizing zeolite supports allows for the production of highly dispersed powders, enhancing the performance of NO decomposition.83 Previous studies have demonstrated the significant contribution of transition metal ions to the photocatalytic NO decomposition. As shown in eqn (20), highly dispersed and isolated transition metal oxides form the charge-transfer excited states under irradiation, which involve electron transfer from O(l)2− to M(l)n+: |  | (20) |
The selectivity of N2 increases as the coordination number of the M–O bond decreases, which triggers the photocatalytic decomposition of NO.83 It was shown that the Ti-oxide/Y-zeolite catalysts with tetrahedral coordination exhibit higher reactivity and selectivity for N2 generation and lower selectivity for N2O formation than those with octahedral coordination.83 The performance of the photoreduction reaction could also be improved by oxygen vacancies and doping the catalyst with transition metals.81,82 van de Krol et al.82 doped TiO2 nanoparticles with Fe3+, which changed the photocatalytic NO removal route from oxidation to reduction (Fig. 4a). The Fe3+ substituted Ti4+ in the lattice, and the negative charge of this acceptor-type dopant contributed to the stabilization of positivity-charged oxygen vacancies. The oxygen vacancies could act as active sites to capture the molecular O of the NO molecule and provide light-induced electrons (Fig. 4b). Besides, Ag0 and Ag+ coexist on the TiO2 surface, which both play different roles in the enhancement of N2 selectivity: the Ag0 nanoparticles enhance the light absorption of the material, while Ag+ can decompose the attachment product N2O into N2.81 A recent study shows that layered structured perovskite SrBi2Nb2O9 with ultrathin nanostructure and oxygen vacancies (SBNO-UT) is capable of decomposing NO into N2 and O2 at the close-to-stoichiometric ratio (Fig. 4c).84 As can be seen from Fig. 4d, N2O intermediates were formed by NO reduction during the photocatalytic process. The in situ formed N2O bounds to the defective surface, ensuring further reduction (Fig. 4e). Finally, the breakage of the N
O bond completes the reduction of NO to N2.
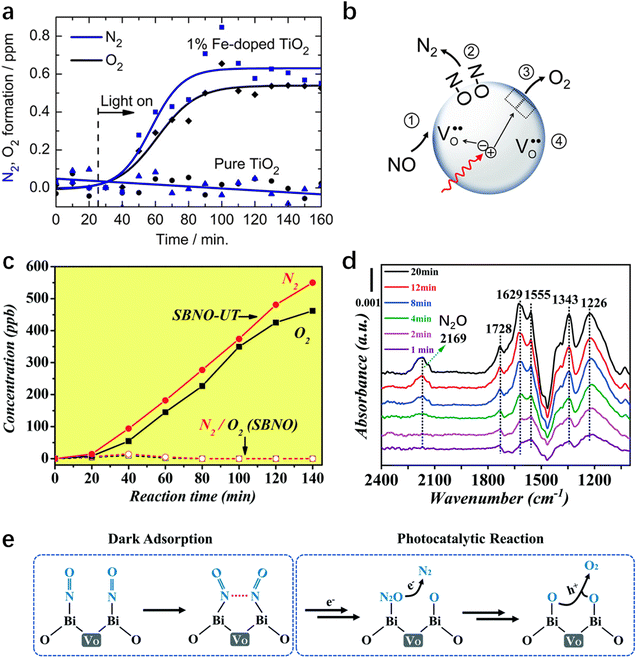 |
| Fig. 4 (a) Photocatalytic conversion of NO to N2 and O2 over 1% Fe-doped TiO2. The sample was irradiated with UV light, and the target pollutant was 100 ppm NO in He. (b) Possible elementary reaction routes of NO on Fe-doped TiO2.82 (c) Formation of photocatalytic NO decomposition products on SBNO and SBNO-UT, respectively, in a sealed system with 13.5 ppm NO. (d) In situ FTIR spectra of SBNO-UT during the photocatalytic NO abatement (0–20 min). The background was collected after the equilibrium of NO adsorption was reached but before illumination. (e) Possible mechanism of the photocatalytic NO decomposition on SBNO-UT.84 | |
3.2 NOx photo-selective reduction
The method of selectively converting NO to N2 by introducing reducing agents such as NH3, CO, H2, and hydrocarbons into the system is known as selective catalytic reduction (SCR). Despite extensive study,85,86 the technology typically operates at high temperatures (300–400 °C),87,88 leading to relatively high energy consumption and potentially excessive CO2 emissions. In addressing these challenges, photo-assisted SCR (photo-SCR) of NOx with NH3 using photocatalysts under light irradiation and low-temperature conditions has been developed as an alternative for NOx reduction.
NH3 serves as a common reductant for NOx removal by photo-SCR, with TiO2 being the most extensively utilized catalyst in this system. The reaction mechanism of NO photo-SCR with NH3 on TiO2 is depicted in Fig. 5a.89 There are numerous TiO2-based materials for photo-SCR, e.g., TiO2/SiO2,90 TiO2 nanotube arrays,91 Bi2WO6/TiO2 Z-scheme heterojunctions,92 g-C3N4/TiO2 (ref. 93) and Pd-loaded TiO2,94 and Si/TiO2.95 Tanaka et al.96 reported achieving the photocatalytic selective reduction of NO with NH3 using TiO2 under xenon lamp illumination at conversion temperatures as low as 50 °C. They also investigated the activity of TiO2 surfaces loaded with 1 wt% of various transition metal oxides.97 Their findings revealed that Nb, Mo, and W oxides promoted the photo-SCR activity of TiO2, with WO3/TiO2 exhibiting the highest activity (Fig. 5b). The number of acidic sites on TiO2 as active sites for the photo-SCR reaction determines the reactivity of the photocatalyst.98 Modification of TiO2 with WO3 resulted in increased polarizability, enhancing the surface acidity and thus facilitating the reduction of NO.99,100 Subsequent studies concluded that agglutination occurs with increasing WO3 addition, with isolated W species enhancing the chemical reduction activity of NO, while agglutinated W species remain inactive.101
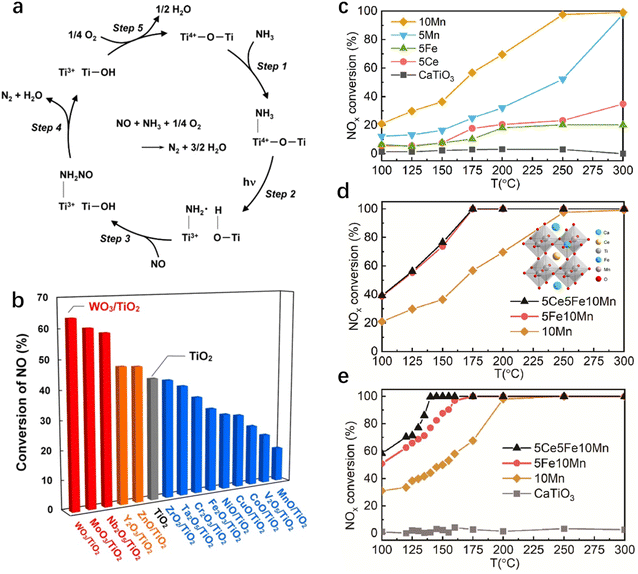 |
| Fig. 5 (a) Proposed reaction mechanism of the photo-SCR over a TiO2 photocatalyst under UV-light irradiation.89 (b) Conversion of NO in the photo-SCR over various metal oxide-promoted TiO2 photocatalysts.102 (c–e) NH3-SCR activity of single- and multi-element doped CaTiO3.103 | |
Perovskite (ABO3) structured materials with flexible structures have recently garnered scientific interest for their application in photo-SCR. Researchers have synthesized Ce–Fe–Mn doped CaTiO3 perovskite from titanium-containing solid waste using the molten salt method.103Fig. 5c–e illustrate that CaTiO3 showed no photocatalytic activity for NO removal at temperatures ranging from 100 to 300 °C. However, (Ca, Ce) (Ti, Mn, Fe) O3 demonstrated almost 100% NO conversion under light irradiation at 135 °C (GHSV = 72
000 h−1). Both Mn and Fe are incorporated into the B-site of CaTiO3, while Ce occupies the A-site. Mn doping enhances material light absorption and the capacity to capture NO. After Fe doping, the oxidation center for NO-to-NO3− shifts from the five-coordinated Mn5c site to the Fe5c site, significantly altering the reaction pathway of conventional SCR. Furthermore, Pr doping of LaCoO3 supported on a natural palygorskite (Pal) surface eliminates over 95% of NOx within the low-temperature range of 150–250 °C.104 Appropriate Mn doping into LaFeO3 to form LaFe1−xMnxO3/attapulgite (ATP) nanocomposites extends its visible light absorption range, resulting in increased NO conversion, reaching a maximum of 85%, with N2 selectivity close to 100%.87 Ni-doped LaFeO3 nanocomposites exhibit a higher NOx conversion rate of 92%.105 Moreover, nitrogen-doped carbon quantum dot modified PrFeO3/Pal, with abundant acid sites, demonstrate 93% NO removal and 100% N2 selectivity under visible light illumination, showing considerable tolerance to SO2 and H2O.106
4 NOx upcycling to ammonia
The upcycling of NOx to ammonia presents an environmentally friendly opportunity for both NOx pollutant elimination and sustainable ammonia production. While electrocatalytic synthesis of ammonia has garnered considerable attention, photocatalytic reduction of NO to ammonia remains in its nascent stage. Nevertheless, recent years have seen significant advancements in photocatalytic ammonia synthesis. The authors outline two potential approaches for NOx resource utilization via photocatalysis. One method involves the use of a chelating agent with the dissolution of NO in water, followed by a one-step reduction to ammonia via photocatalysis (one-step method). Another approach entails oxidizing NO to the highly water-soluble NO3− initially, followed by its reduction to ammonia through an eight-electron reduction process (two-step method).
4.1 One-step method (NO → NH3)
The direct one-step synthesis of NH3 from NO is an ideal strategy for transforming waste into valuable resources. While research efforts in NOx reduction reactions (NOxRR) have predominantly focused on enhancing NH3 synthesis rates, achieving high NOx conversion efficiency remains a crucial yet less explored objective.107,108 Typically, NOxRR experiments necessitate efficiency tests with high concentrations (>10
000 ppm) of NO to ensure an adequate feedstock for enhancing ammonia production.109 However, limited NO conversion persists as a key challenge for the sustainability of the NO-to-NH3 reduction pathway.
The ultra-low solubility of NO in water (1.94 mmol L−1) is one of the primary reasons for limited NO conversion.110 To address this challenge, a novel one-step NOx photoreduction pathway, referred to as the on-site coupling system, has been recently developed. This system enables NO direct upcycling under ambient conditions (Fig. 6a).111 Specifically, the solution was supplemented with Fe(II)EDTA as a NO chemical absorbent, generating Fe(II)EDTA-NO chelates, while formaldehyde (HCHO) served as an antioxidant to prevent the Fe(III) formation from Fe(II) oxidation. This simultaneous chemical absorption and photocatalytic reduction system enabled continuous NO adsorption, NO reduction, and Fe(II)EDTA regeneration on-site.111 TiO2 decorated with 11.6 mg L−1 Au nanoparticles could provide ample active sites to facilitate charge separation, thereby enhancing NH4+ generation. Using this on-site coupling system, the performance of AuNPs–TiO2 is shown in Fig. 6b and c, demonstrating exceptional NO conversion efficiency (89.0%), ammonia production selectivity (95.6%), and ammonia recovery efficiency (>90%). Virtually no other side products are detected. Subsequently, the generated ammonia was recovered via a simple ion exchange method, with recovery rates still exceeding 90% within 50–200 mg L−1 ammonia production (Fig. 6d). Moreover, the NO conversion efficiency and the NH4+ selectivity remained unaffected even in the presence of resistance factors such as H2O, SO2, and metal ions (K+, Ca2+, Cd2+, and Pb2+) (Fig. 6e). These high recovery efficiencies and anti-poisoning capacities underscore the environmental practicality of NOx removal in flue gas. The on-site coupling strategy achieves significant efficiency milestones for sustainable NO removal and also offers insights into NO upcycling for the future of carbon neutrality.
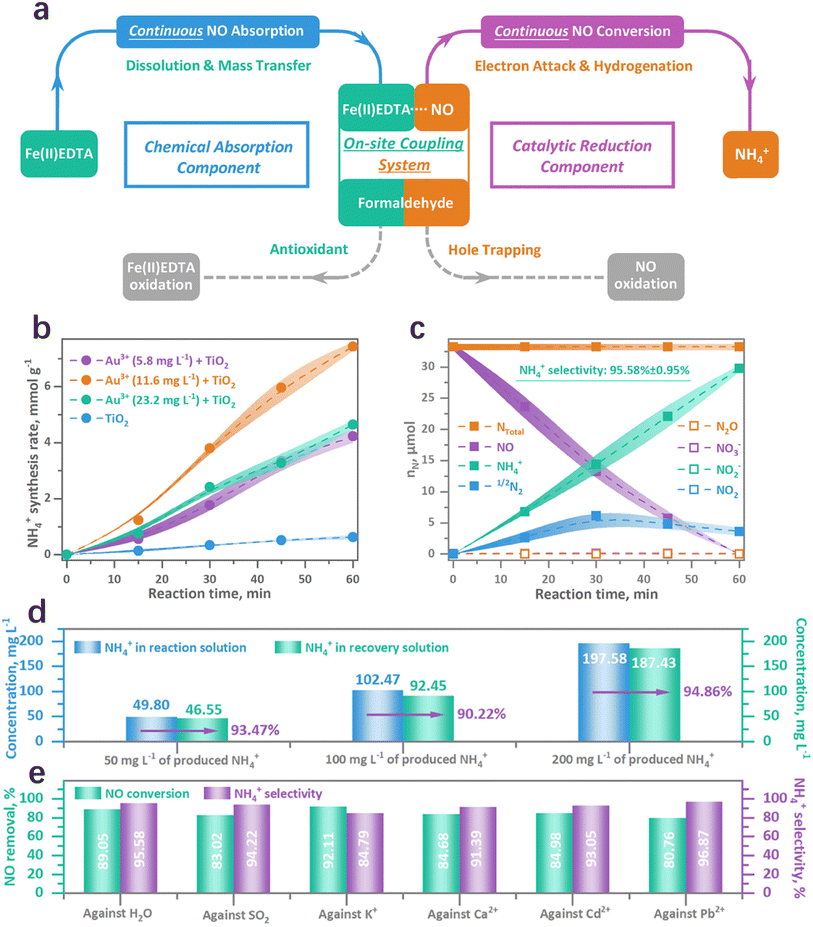 |
| Fig. 6 (a) Illustration of the on-site coupling system of continuous chemical absorption and catalytic reduction. (b) NH4+ synthesis rate between the pristine and Au-decorated TiO2. (c) Selectivity test. (d) Ammonia recovery evaluation after the continuous absorption and photoreduction of NO. (e) Poisoning resistance against vapor, SO2, and metals.111 | |
The simultaneous presence of NO and SO2 in flue gas poses significant challenges for their concurrent removal and recovery.112,113 Since SO2 is easily dissolved and oxidized, it can serve as a potential reductant in the NO photoreduction reaction. Furthermore, the on-site coupled system demonstrated the synergistic removal and recycling of NO and SO2 without the need for scavengers.114 The formation of the SO2–NO redox pair facilitates high conversion rates of both NO and SO2 in continuous flow. Achieving a remarkable selectivity for both NO-to-NH3 upcycling (97%) and SO2-to-SO42− purification (92%) simultaneously underscores the practicability of value-added conversion of air pollutants (Fig. 7a and b).114 Maintaining the efficiency of NO conversion and reduction crucially depends on preventing the oxidation of Fe(II) to Fe(III) in Fe(II) EDTA. Through verified in situ ATR-FTIR experiments, it was revealed that SO2 exhibited a promoting effect on Fe(II) maintenance, while no interaction was observed between SO2 and EDTA (Fig. 7c and d).
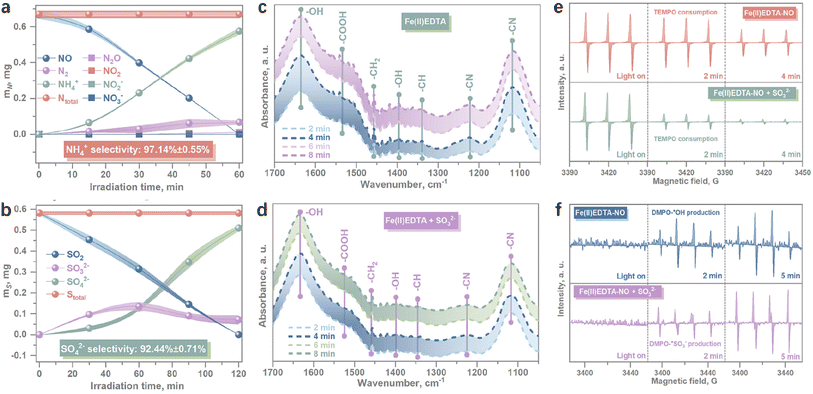 |
| Fig. 7 The continuous selectivity evaluation for (a) NO-to-NH3 reduction and (b) SO2-to-SO42− oxidation. In situ ATR-FTIR spectra for the recording of Fe(II)EDTA characterization signals (c) without and (d) in the presence of SO32− provision. (e) In situ EPR measurements for the consumption of TEMPO in the photocatalytic reduction process of NO without (up) and with (down) SO32− provision, respectively, (f) In situ EPR measurements for the production of DMPO-˙OH by H2O oxidation (up) and DMPO-˙SO3− by SO32− oxidation (down), respectively.114 | |
The chemical redox pair of SO2–Fe(III) is illustrated in eqn (21)–(23).114
| Fe(II) → Fe(III) (spontaneous oxidation) | (21) |
| SO2 + H2O → SO32− + 2H+ (dissolution) | (22) |
| 2Fe(III) + 2SO32− + O2 → 2Fe(II) + 2SO42− (chemical redox pair) | (23) |
As depicted in Fig. 7e and f, compared to h+-induced ˙OH production, the h+-induced SO2 oxidation reaction to ˙SO3− promotes charge separation, thereby generating more e−, and enhancing the efficiency of the nitrogen oxide reduction reaction to produce NH3. The overall mechanism for synchronous NO and SO2 conversion is illustrated in eqn (24)–(26):
| SO2 + H2O → SO32− + 2H+ (dissolution) | (24) |
| 5SO32− + NO + 5h+ + 5e− + 5H+ →5˙SO3− + NH4+ + OH− (photocatalytic redox pair) | (25) |
| 5˙SO3− + NO + 5h+ + 5e− + 5H+ + 9OH− → 5SO42− + NH4+ + 5H2O (photocatalytic redox pair) | (26) |
Thus, a one-step NOx reduction method has been devised by concurrently absorbing and converting NOx. This system also exhibits great resistance to toxicity, including resistance to K+, Ca2+, and Cd2+ metal ions, respectively. The effective separation and retrieval of reaction products provide this system with sustainable stability and potential economic viability. Continuous NOx removal and upcycling under ambient conditions with high conversion efficiencies were achieved, marking significant advancements in photocatalytic NOx removal and recycling compared to SCR and chemical absorption technologies.
4.2 Two-step method (NO → NO3− → NH3)
Among the redox products of NOx, NO3− is the dominant and accessible substance on the surface of the photocatalyst.39,67 Significant quantities of NO3− are released into the environment through processes such as the combustion of fossil fuels and agricultural activities, driven by the progress of urbanization and industrialization.115 The surface of the photocatalyst is the overlooked storage site for NO3−, where the generated nitrate could be easily removed from the catalyst and subsequently concentrated in liquid form.69,116 However, excessive amounts of NO3− in water can pose a pollution concern. To address this issue, photocatalytic technologies have been employed to convert excess nitrate into valuable NH3.
Photocatalytic reduction of NO3− to ammonia via multiple electron transfer (eqn (27)), could emerge as a potent method for ammonia production under ambient conditions of room temperature and atmospheric pressure.117 N2 and H2 formation reactions are two side reactions that consume photoexcited electrons (eqn (28) and (29)).117,118 Therefore, it is necessary to enhance not only the ammonia yield but also the ammonia selectivity. A summary of studies on the reduction process of nitrate to ammonia by various photocatalysts is presented in Table 1.
| NO3− + 9H+ + 8e− → NH3 + 3H2O (1.20 V vs. NHE) | (27) |
| NO3− + 6H+ + 5e− → 1/2N2 + 3H2O (1.25 V vs. NHE) | (28) |
| 2H+ + 2e− → H2 (0.00 V vs. NHE) | (29) |
Table 1 Comparison of the results of NH3 production with different photocatalysts
Photocatalysts |
Hole scavengers |
Light source |
pH |
NO3− conversion (%) |
NH4+ production (mmol gcat−1 h−1) |
NH4+ selectivity (%) |
Ref. |
Cu2ONCs-TNS |
Formaldehyde |
300 W Xe lamp |
N/A |
94.2 |
42.6 |
98.6 |
119
|
CuOx@TNS |
Ethylene glycol |
300 W Xe lamp |
8.3 |
100 |
16 |
96.1 |
120
|
Cu-TNS |
Formic acid |
300 W Xe lamp |
3.5–3.6 |
>99 |
0.1 |
97.6 |
118
|
BaONCs@TNS |
Ethylene glycol |
300 W Xe lamp |
7 |
∼3.5 |
89.8 |
97.7 |
121
|
Photo-reductive TiO2 |
N/A |
450 W medium pressure UV |
7 |
∼71 |
N/A |
60 |
122
|
LaFeO3/HTCC nanocomposites |
Acetic acid |
300 W Xe lamp |
2 |
94.6 |
N/A |
88.7 |
123
|
LaFeO3/biochar nanocomposites |
Formic acid |
300 W Xe lamp |
2 |
98 |
N/A |
97 |
124
|
Ni/HxWO3−y hybrids |
Ethylene glycol |
300 W Xe lamp |
N/A |
∼92 |
10.5 |
98.3 |
125
|
Carbon/bismuth/Bi2O3 |
Ethylene glycol |
Simulated sunlight |
N/A |
N/A |
0.4 |
95 |
126
|
CuAg/TiO2 |
Methanol |
Black light |
6 |
96 |
N/A |
85 |
127
|
Ni2P/Ta3N5 |
N/A |
Fluorescent lamps |
2 |
79 |
0.2 |
68 |
128
|
PdSn/NiO/NaTaO3:La |
Formic acid |
125 W high pressure UV |
N/A |
100 |
N/A |
72 |
129
|
JRC-TIO-6 (rutile TiO2) |
Formic acid |
2 kW Xe lamp |
2.4–3 |
79 |
0.02 |
97 |
117
|
CuPd/TiO2 |
Methanol |
UV |
N/A |
>95 |
N/A |
78 |
130
|
It can be inferred from Table 1 that TiO2-based materials are prominently utilized as catalysts for NO3−-to-NH3 reduction, particularly highlighting metal-modified TiO2 nanosheets (TNSs). Recently, as shown in Fig. 8a, alkaline-earth oxide clusters constructed on TiO2 nanosheets (MONCs-TNSs, M = Mg, Ca, Sr or Ba) were reported to enhance NO3−-to-NH4+ production, among which BaONCs-TNSs achieve both exceedingly high NH4+ selectivity (97.7%) and high NH4+ yield (89.8 mmol gcat−1 h−1).121 To the best of our knowledge, this is the highest production of ammonia by the photocatalytic reduction reaction. Remarkably, there's an incremental rise in the rate of ammonia synthesis throughout the reaction, attributed to the concurrent Operando formation of BaONCs and the ammonia synthesis reaction (Fig. 8b and c). Upon achieving stabilization of BaONCs on TNSs, a notable and consistent production of ammonia on BaONCs-TNS composites was realized.
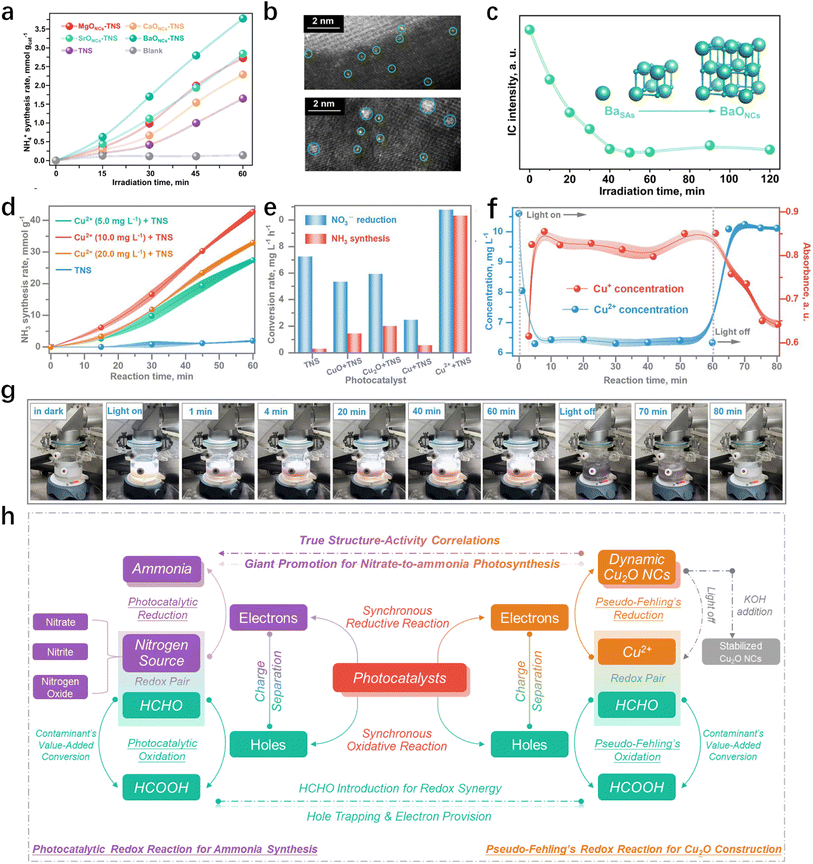 |
| Fig. 8 (a) Catalytic efficiency tests showing the enhancement of Operando construction of alkaline-earth oxide clusters on TNS surfaces. (b) Quasi in situ high-angle annular dark-field scanning transmission electron microscopy (HAADF-STEM) images showing the evolution course from isolated Ba single atoms (BaSAs) to subnanometric (BaONCs) at the irradiation time of 5 min (up) and 10 min (down). (c) Variation of Ba2+ concentration during the Operando construction of BaONCs detected by ion chromatography.121 (d) NH3 photosynthesis rate of the dynamic process. (e) Efficiency comparison between the different composites of Cu species and the TNS substrate. (f) The evolution process of the different chemical states of Cu2+ and Cu+ under the catalysis conditions. (g) Snapshots of the photosynthesis reactor along with the photosynthesis reaction time for the observation of the dynamic evolution of Cu species. (h) Schematic illustrations for the synchronous Cu2O NCs construction and NH3 photosynthesis in the realistic catalysis condition.119 | |
Chen et al.119 further elucidated the criticality of the on-site formation of authentic active sites under realistic catalytic conditions. They engineered Cu2ONCs-TNS materials, wherein dynamic Cu2O sub-nanoclusters were on-site constructed on TNSs using CuCl2 solution through a photoinduced pseudo-Fehling's route. Different from BaONCs-TNS which remains stable once it has been constructed, the morphology and chemical state of Cu2O exhibit dynamic evolution, as the actual reaction cannot progress over preformed Cu2O sites (Fig. 8d and e). Numerous in situ experiments revealed a photoswitchable reversible conversion pattern between Cu2+ and Cu+ in the photosynthesis reaction, aligning with the performance of ammonia synthesis by nitrate reduction (Fig. 8f and g).119 NH3 photosynthesis was directly attributed to the enhanced charge separation and transformation capacity from the Cu2O active sites. Consequently, the establishment of a genuine structure–activity correlation was achieved by uncovering the synchronous formation of the Cu2O active site and catalytic reaction (Fig. 8h).
In addition, a groundbreaking perspective has emerged regarding the interplay between single atoms and oxygen vacancies (OVs) (Fig. 9).118 The incorporation of single-atom Cu into TiO2 nanosheets (Cu-TNSs) resulted in a 62-fold increase in ammonia production compared to pristine TiO2, with a selectivity of 97.6%. The introduction of Cu atoms, replacing Ti sites, induces the generation of OVs and lattice strain. NO3− preferentially adsorbs onto Ti atoms neighboring Cu atoms. The near-isolated Cu atoms and OVs facilitate multiple NO3− adsorptions at a single site, effectively inhibiting the release of NO2− intermediates and minimizing N-to-N interactions, thus ensuring high NH3 selectivity.
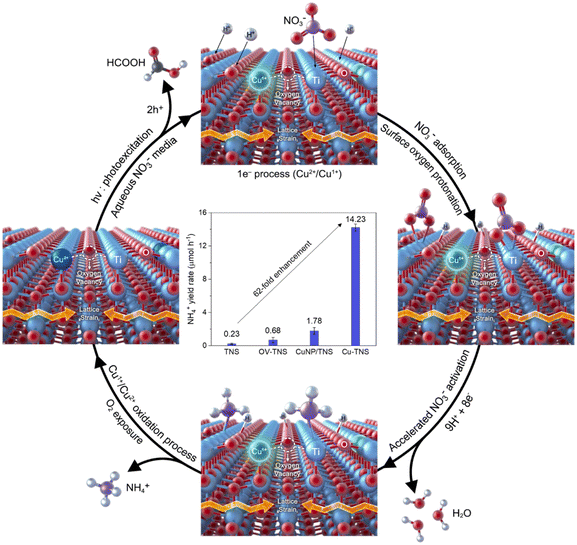 |
| Fig. 9 NO3−-to-NH3 reaction mechanism on Cu-TNSs. TiO2 with isolated Cu2+ atomic sites (blue) and defects generates photoexcited electrons and holes under light irradiation.118 | |
Furthermore, CuOx@TNS was designed for NO3− reduction with a 100% NO3− conversion rate and 96.1% ammonia selectivity by coupling the nitrate reduction reaction with a glycol oxidation reaction system.120 The active site for improving ammonia formation is identified as the CuOx species with an amorphous Cu–O–Ti bimetallic oxide cluster structure (Fig. 10a).120 Given that redox reactions occur concurrently, it's evident that the oxidation half-reaction also significantly influences NH3 production. To explore the impact of various oxidation half-reactions on NO3− reduction, oxidative reactants including deionized water (DI), methanol (CH3OH), ethylene glycol ((CH2OH)2), and acetic acid (HCOOH) are introduced into the reaction system (Fig. 10b and c). It is revealed that the NO3− reduction process is hindered by the formation of potent oxidizing ˙OH radicals (Fig. 10d). The presence of ethylene glycol expedites the consumption of h+ during the formation of alkoxy radicals (˙R), thus mitigating ˙OH production. Moreover, the Cu–O–Ti sites could promote the preferential oxidation of ethylene glycol, thereby enhancing both the efficiency and selectivity of ammonia production (Fig. 10e). These findings underscore the pivotal role of the oxidation half-reaction. The deliberate design of the oxidation half-reaction not only enhances the effective utilization of electrons and holes but also modulates the reaction pathway, thus promoting the progress of the NO3− reduction half-reaction.
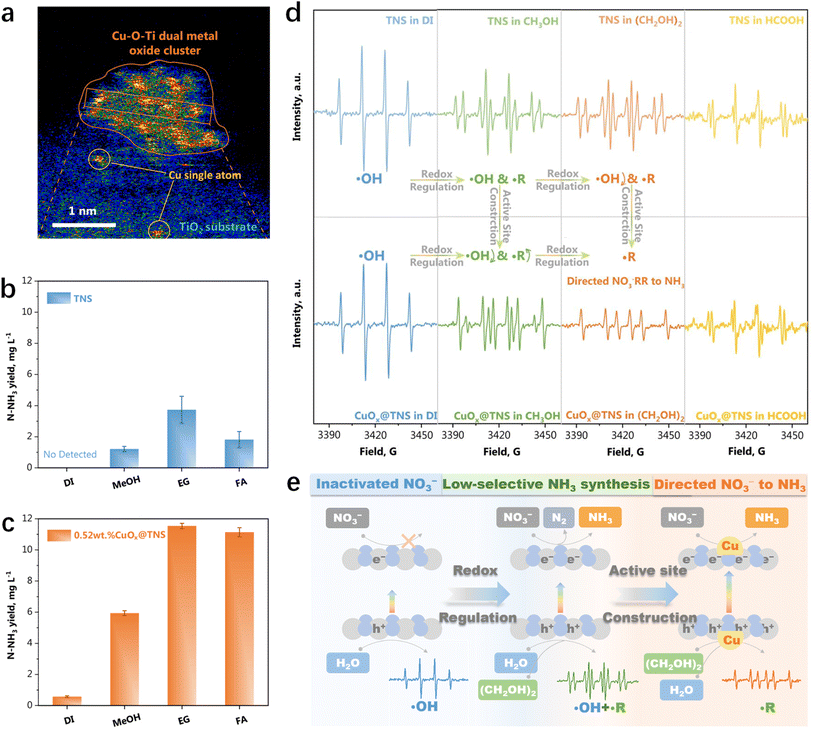 |
| Fig. 10 (a) Magnified HAADF-STEM image of 0.52 wt% CuOx@TNS. NH3 photosynthesis yields under different cooperative oxidative half-reactions for (b) TNSs and (c) 0.52 wt% CuOx@TNS after 1 h irradiation. (d) EPR spectra of DI, CH3OH, (CH2OH)2, and HCOOH oxidative half-reactions on TNSs and 0.52 wt% CuOx@TNS catalyst after light irradiation for 5 min. (e) Illustration of the directed NO3−RR by coordination of different oxidative reactions.120 | |
In summary, the photocatalytic performance of NO3−-to-NH3 photocatalytic reduction highly relies on the strategic construction of active sites and the coordination of redox reactions. This offers an innovative perspective on experimental design aimed at achieving high NH3 selectivity and production. Recent studies have shown that NO3− originating from NO oxidation has achieved nearly 100% NH3 selectivity. It is desired to employ a two-step process (NO → NO3− → NH3) to achieve a highly selective conversion of NO to NH3.
5 Conclusions and perspectives
5.1 Conclusions
Table 2 summarizes and compares the application scenarios, reaction conditions, photocatalysts, and reaction activity for NOx removal under the different reaction pathways. It is found that the application of photocatalytic technology has transcended indoor NOx treatment and is now addressing challenges posed by complex pollutant compositions and industrial flue gases. Interestingly, TiO2 remains the most extensively studied photocatalyst in both NOx oxidation and NOx reduction, probably due to its outstanding carrier separation capability. Besides, the overall redox properties also depend on catalyst modification or adjustment of environmental atmospheres in different systems. The photocatalytic performance showed that the NOx conversion and product selectivity have exceeded 90% for most of the redox pathways except NOx decomposition. This marks a significant advancement towards the future application of photocatalytic NOx removal and recovery.
Table 2 Conclusion and comparison of NOx removal by various reaction pathways
Reaction pathway |
Product |
Scenarios of application |
Typical photocatalysts |
NOx concentration |
Temperature (°C) |
Flow rate (L min−1)/GHSV (h−1) |
Carrier gas |
Supplements |
Conversion (%) |
Selectivity (%) |
NOx oxidation |
Single oxidation |
NO3− |
Air purification |
TiO2, g-C3N4, WO3, Bi-based materials |
∼600 ppb |
Room temperature |
1 L min−1 |
Air |
N/A |
∼90 |
∼97 |
Mixed oxidation |
NO3− |
Air purification |
TiO2, In(OH)3, Sr2Sb2O7 |
30 ppm |
Room temperature |
1 L min−1 |
Air |
VOCs, SO2 |
∼100 |
N/A |
NOx reduction |
NOx decomposition |
N2 |
Air purification |
TiO2, g-C3N4 |
<1 ppm |
N/A |
1 L min−1 |
Air/Ar |
N/A |
∼50 |
N/A |
NOx photo-SCR |
N2 |
Flue gas treatment |
TiO2, perovskite |
1000 ppm |
<200 |
50 000 h−1 |
3% O2, 97% N2 |
NH3, H2, hydrocarbon, etc. |
>90 |
∼100 |
NOx upcycling |
One-step method |
NH3 |
Flue gas treatment |
TiO2 |
500 ppm |
25 |
25 000 h−1 |
N/A |
Fe(II)EDTA/hole scavengers (e.g. HCHO, SO2) |
97.6 |
∼97.1% |
In conclusion, photocatalytic technology is a promising method for NOx removal and recovery, owing to its mild reaction conditions and eco-friendliness. In recent years, significant strides have been made in the field of photocatalytic NOx removal, spanning from the optimization of photocatalysts to the exploration of mechanisms and the modulation of reaction pathways, resulting in unprecedented performance in NOx removal. The emerging photocatalytic reduction of NOx to ammonia offers a viable alternative for NOx recovery. This review summarizes the latest advancements in photocatalytic removal, encompassing NOx oxidation (both single and synergistic removal, as well as NO3− decomposition), NOx reduction to nitrogen, and the upcycling of NOx into ammonia. Furthermore, we highlight photocatalysis-based NOx recovery, including one-step and two-step methods, as a promising approach to tackle existing environmental and energy challenges.
5.2 Future outlook
The utilization of photocatalytic technology for the removal and recovery of NOx is currently in the research phase, with the practical application still a considerable distance away. We recommend that future studies systematically address the following issues:
(1) Comparing the performance of photocatalysts across different studies in the realm of NOx oxidation proves challenging due to the diverse array of reaction conditions such as light source, gas flow rate, initial NOx concentration, mass of the photocatalyst, reactor design, and others. To comprehensively evaluate photocatalyst performance and identify truly efficient photocatalysts, there is a pressing need to establish uniform performance standards.
(2) Despite notable advancements in the performance of photocatalytic NOx removal in recent years, a gap persists when compared to thermal catalytic NOx removal. One of the primary reasons for this gap is the insufficient exploration of the interfacial reaction mechanism governing photocatalytic NOx removal. To address this, in situ detection methods with higher resolution, such as in situ EPR, DRIFTS, and Raman spectroscopy, should be more extensively employed. These techniques can unveil the intricacies of the photocatalytic reaction mechanism, subsequently informing catalyst design and modification efforts.
(3) In the one-step method, the yield of ammonia is significantly influenced by the solubility of NO, thus necessitating further enhancement of NO solubility in water. Introducing chemical absorbents to adsorb NO suggests the need for additional methods to separate the resulting ammonia, thereby increasing costs and rendering it less favorable for practical applications. An absorbent-free approach is preferable to augment the solubility of high concentrations of NO in water and to develop photocatalysts capable of achieving an ammonia yield that meets recovery criteria. Moreover, photocatalysts can be selectively designed to further reduce NOx to N2 instead of ammonia, offering an alternative solution to address current environmental challenges.
(4) In the two-step process, the primary challenge lies in effectively collecting the nitrates produced from NOx. During the photocatalytic NOx oxidation process, nitrates gradually accumulate on the catalyst surface. However, due to the limited availability of adsorption sites on the surface and the simultaneous decomposition of nitrates, only a small portion of nitrates remains on the photocatalyst surface. It is imperative to enhance the thorough oxidation of NOx and suppress the decomposition of nitrates. Additionally, nitrates are commonly found in relatively high concentrations in wastewater, presenting an opportunity to enhance ammonia production.
(5) Machine learning offers a potent tool for predicting catalytic reaction performance accurately, aiding chemists in tasks like material screening, experiment optimization, and mechanistic studies. Nonetheless, it's crucial to emphasize that machine learning should be applied judiciously. While it's a valuable tool, it's not a universal solution and cannot substitute well-considered experimental designs. In practice, a combination of expertise is still vital to ensure that machine learning and traditional methods work together harmoniously to achieve broader environmental science objectives.
Author contributions
All of the authors contributed to the literature search, writing and editing of this review.
Conflicts of interest
There are no conflicts to declare.
Acknowledgements
This work was supported by the National Natural Science Foundation of China (22261142663, 22225606, and 22176029).
References
- J. Lelieveld, J. S. Evans, M. Fnais, D. Giannadaki and A. Pozzer, The contribution of outdoor air pollution sources to premature mortality on a global scale, Nature, 2015, 525, 367–371 CrossRef CAS.
- S. C. Anenberg, J. Miller, R. Minjares, L. Du, D. K. Henze, F. Lacey, C. S. Malley, L. Emberson, V. Franco, Z. Klimont and C. Heyes, Impacts and mitigation of excess diesel-related NOx emissions in 11 major vehicle markets, Nature, 2017, 545, 467–471 CrossRef CAS.
- D. Shindell, G. Faluvegi, M. Walsh, S. C. Anenberg, R. Van Dingenen, N. Z. Muller, J. Austin, D. Koch and G. Milly, Climate, health, agricultural and economic impacts of tighter vehicle-emission standards, Nat. Clim. Change, 2011, 1, 59–66 CrossRef CAS.
- N. Li, C. Wang, K. Zhang, H. Lv, M. Yuan and D. W. Bahnemann, Progress and prospects of photocatalytic conversion of low-concentration NOx, Chin. J. Catal., 2022, 43, 2363–2387 CrossRef CAS.
- M. G. Lawrence and P. J. Crutzen, Influence of NOx emissions from ships on tropospheric photochemistry and climate, Nature, 1999, 402, 167–170 CrossRef CAS.
- J. Lasek, Y. H. Yu and J. C. S. Wu, Removal of NOx by photocatalytic processes, J. Photochem. Photobiol., C, 2013, 14, 29–52 CrossRef CAS.
- V. Smil, Detonator of the population explosion, Nature, 1999, 400, 415 CrossRef CAS.
- S. Su, Y. Liang, G. Yin, Q. Wang, Y. Cai, X. Peng, M. N. Pervez and L. Lin, Anhydrous dyeing processes of ramie fiber in liquid ammonia, Cellulose, 2019, 26, 8109–8120 CrossRef CAS.
- T. Wu, X. Zhu, Z. Xing, S. Mou, C. Li, Y. Qiao, Q. Liu, Y. Luo, X. Shi, Y. Zhang and X. Sun, Greatly Improving Electrochemical N2 Reduction over TiO2 Nanoparticles by Iron Doping, Angew. Chem., Int. Ed., 2019, 58, 18449–18453 CrossRef CAS PubMed.
- R. Schlögl, Catalytic Synthesis of Ammonia—A “Never-Ending Story”, Angew. Chem., Int. Ed., 2003, 42, 2004–2008 CrossRef.
- J. Egerer, V. Grimm, K. Niazmand and P. Runge, The economics of global green ammonia trade - “Shipping Australian wind and sunshine to Germany”, Appl. Energy, 2023, 334, 120662 CrossRef.
- Q. An, M. McDonald, A. Fortunelli and W. A. Goddard III, Si-Doped Fe Catalyst for Ammonia Synthesis at Dramatically Decreased Pressures and Temperatures, J. Am. Chem. Soc., 2020, 142, 8223–8232 CrossRef CAS PubMed.
- X. Guo, P. Wang, T. Wu, Z. Wang, J. Li, K. Liu, J. Fu, M. Liu, J. Wu, Z. Lin, L. Chai, Z. Bian, H. Li and M. Liu, Aqueous Electroreduction of Nitric Oxide to Ammonia at Low Concentration via Vacancy Engineered FeOCl, Angew. Chem., Int. Ed., 2023, e202318792 Search PubMed.
- K. A. Brown, D. F. Harris, M. B. Wilker, A. Rasmussen, N. Khadka, H. Hamby, S. Keable, G. Dukovic, J. W. Peters, L. C. Seefeldt and P. W. King, Light-driven dinitrogen reduction catalyzed by a CdS: nitrogenase MoFe protein biohybrid, Science, 2016, 352, 448–450 CrossRef CAS PubMed.
- Q. Guo, Z. B. Ma, C. Y. Zhou, Z. F. Ren and X. M. Yang, Single Molecule Photocatalysis on TiO2 Surfaces, Chem. Rev., 2019, 119, 11020–11041 CrossRef CAS PubMed.
- T. Wu, X. J. Liu, Y. Liu, M. Cheng, Z. F. Liu, G. M. Zeng, B. B. Shao, Q. H. Liang, W. Zhang, Q. Y. He and W. Zhang, Application of QD-MOF composites for photocatalysis: Energy production and environmental remediation, Coord. Chem. Rev., 2020, 403, 213097 CrossRef CAS.
- F. He, S. Weon, W. Jeon, M. W. Chung and W. Choi, Self-wetting triphase photocatalysis for effective and selective removal of hydrophilic volatile organic compounds in air, Nat. Commun., 2021, 12, 6259 CrossRef CAS PubMed.
- S. Wang, W. Cui, B. Lei, X. A. Dong, Y. Tang and F. Dong, Targeted NO Oxidation and Synchronous NO2 Inhibition via Oriented 1O2 Formation Based on Lewis Acid Site Adjustment, Environ. Sci. Technol., 2023, 57, 12890–12900 CrossRef CAS PubMed.
- R. Hailili, Z.-Q. Wang, H. Ji, C. Chen, X.-Q. Gong, H. Sheng and J. Zhao, Mechanistic insights into the photocatalytic reduction of nitric oxide to nitrogen on oxygen-deficient quasi-two-dimensional bismuth-based perovskites, Environ. Sci.: Nano, 2022, 9, 1453–1465 RSC.
- J. C.-C. Yu, V.-H. Nguyen, J. Lasek and J. C. S. Wu, Titania nanosheet photocatalysts with dominantly exposed (001) reactive facets for photocatalytic NOx abatement, Appl. Catal., B, 2017, 219, 391–400 CrossRef CAS.
- V.-H. Nguyen, B.-S. Nguyen, C.-W. Huang, T.-T. Le, C. C. Nguyen, T. T. Nhi Le, D. Heo, Q. V. Ly, Q. T. Trinh, M. Shokouhimehr, C. Xia, S. S. Lam, D.-V. N. Vo, S. Y. Kim and Q. V. Le, Photocatalytic NOx abatement: Recent advances and emerging trends in the development of photocatalysts, J. Cleaner Prod., 2020, 270, 121912 CrossRef CAS.
- X. Shi, P. Wang, W. Li, Y. Bai, H. Xie, Y. Zhou and L. Ye, Change in photocatalytic NO removal mechanisms of ultrathin BiOBr/BiOI via NO3- adsorption, Appl. Catal., B, 2019, 243, 322–329 CrossRef CAS.
- K. Li, H. Wang, J. Li and F. Dong, Design and mechanism of photocatalytic oxidation for the removal of air pollutants: a review, Environ. Chem. Lett., 2022, 20, 2687–2708 CrossRef CAS.
- Q. Li, J. Zhao, H. Shang, Z. Ma, H. Cao, Y. Zhou, G. Li, D. Zhang and H. Li, Singlet Oxygen and Mobile Hydroxyl Radicals Co-operating on Gas-Solid Catalytic Reaction Interfaces for Deeply Oxidizing NOx, Environ. Sci. Technol., 2022, 56, 5830–5839 CrossRef CAS PubMed.
- A. Martinez-Oviedo, Y. K. Kshetri, B. Joshi and S. W. Lee, Surface modification of blue TiO2 with silane coupling agent for NOx abatement, Prog. Nat. Sci.: Mater. Int., 2021, 31, 230–238 CrossRef CAS.
- Y. Bu, B. Wang, M. Yue, Q. Ren, L. Guo and Y. Fu, Photocatalytic NO removal based on TiO2 photonic crystals through slow photon effect, Mater. Lett., 2023, 346, 134537 CrossRef CAS.
- M. Kamran, T. A. Kandiel, S. Abdel-Azeim, M. A. Morsy and D. W. Bahnemann, Mechanistic Insights into the High Selectivity and Photocatalytic Activity of Brookite TiO2 toward NOx Abatement, J. Phys. Chem. C, 2023, 127, 7707–7717 CrossRef CAS.
- Y. Xin, Q. Zhu, T. Gao, X. Li, W. Zhang, H. Wang, D. Ji, Y. Huang, M. Padervand, F. Yu and C. Wang, Photocatalytic NO removal over defective Bi/BiOBr nanoflowers: The inhibition of toxic NO2 intermediate via high humidity, Appl. Catal., B, 2023, 324, 122238 CrossRef CAS.
- Q. Zhu, Y. Wang, J. Wang, J. Luo, J. Xu and C. Wang, Synergistic polarization and oxygen vacancies engineering for enhancing photocatalytic NO removal over Bi4Ti3O12 nanowires, Appl. Catal., B, 2024, 346, 123734 CrossRef CAS.
- Y. Shi, Z. Yang, L. Shi, H. Li, X. Liu, X. Zhang, J. Cheng, C. Liang, S. Cao, F. Guo, X. Liu, Z. Ai and L. Zhang, Surface Boronizing Can Weaken the Excitonic Effects of BiOBr Nanosheets for Efficient O2 Activation and Selective NO Oxidation under Visible Light Irradiation, Environ. Sci. Technol., 2022, 56, 14478–14486 CrossRef CAS.
- D. Liu, D. Chen, N. Li, Q. Xu, H. Li, J. He and J. Lu, Surface Engineering of g-C3N4 by Stacked BiOBr Sheets Rich in Oxygen Vacancies for Boosting Photocatalytic Performance, Angew. Chem., Int. Ed., 2020, 59, 4519–4524 CrossRef CAS PubMed.
- Y. Geng, D. Chen, N. Li, Q. Xu, H. Li, J. He and J. Lu, Z-Scheme 2D/2D α-Fe2O3/g-C3N4 heterojunction for photocatalytic oxidation of nitric oxide, Appl. Catal., B, 2021, 280, 119409 CrossRef CAS.
- Z. Chen, H. Yin, R. Wang, Y. Peng, C. You and J. Li, Efficient Electron Transfer by Plasmonic Silver in SrTiO3 for Low-Concentration Photocatalytic NO Oxidation, Environ. Sci. Technol., 2022, 56, 3604–3612 CrossRef CAS.
- H. Shang, H. Jia, P. Li, H. Li, W. Zhang, S. Li, Q. Wang, S. Xiao, D. Wang, G. Li and D. Zhang, Highly selective and efficient photocatalytic NO removal: Charge carrier kinetics and interface molecular process, J. Nano Res., 2024, 17, 1003–1026 CrossRef CAS.
- G. Liu, Y. Huang, H. Lv, H. Wang, Y. Zeng, M. Yuan, Q. Meng and C. Wang, Confining single-atom Pd on g-C3N4 with carbon vacancies towards enhanced photocatalytic NO conversion, Appl. Catal., B, 2021, 284, 119683 CrossRef CAS.
- L. Hu, J. Liu, X. Huang, Q. Nie, P. Liu, Z. Tan and H. Yu, Carbon-bridged atomically dispersed platinum on MOF-derived ZnO/C for selective photocatalytic oxidation of NO into Nitrates and Nitrites, Carbon, 2023, 214, 118299 CrossRef CAS.
- R. Zhang, Y. Cao, D. E. Doronkin, M. Ma, F. Dong and Y. Zhou, Single-atom dispersed Zn-N3 active sites bridging the interlayer of g-C3N4 to tune NO oxidation pathway for the inhibition of toxic by-product generation, Chem. Eng. J., 2023, 454, 140084 CrossRef CAS.
- Z. Hu, X. Li, S. Zhang, Q. Li, J. Fan, X. Qu and K. Lv, Fe1/TiO2 Hollow Microspheres: Fe and Ti Dual Active Sites Boosting the Photocatalytic Oxidation of NO, Small, 2020, 16, 2004583 CrossRef CAS.
- F. Guo, C. Mao, C. Liang, P. Xing, L. Yu, Y. Shi, S. Cao, F. Wang, X. Liu, Z. Ai and L. Zhang, Triangle Cl-Ag1-Cl Sites for Superior Photocatalytic Molecular Oxygen Activation and NO Oxidation of BiOCl, Angew. Chem., Int. Ed., 2023, e202314243 CAS.
- A. M. Żurański, J. I. Martinez Alvarado, B. J. Shields and A. G. Doyle, Predicting Reaction Yields via Supervised Learning, Acc. Chem. Res., 2021, 54, 1856–1865 CrossRef PubMed.
- S. Zhong, K. Zhang, M. Bagheri, J. G. Burken, A. Gu, B. Li, X. Ma, B. L. Marrone, Z. J. Ren, J. Schrier, W. Shi, H. Tan, T. Wang, X. Wang, B. M. Wong, X. Xiao, X. Yu, J.-J. Zhu and H. Zhang, Machine Learning: New Ideas and Tools in Environmental Science and Engineering, Environ. Sci. Technol., 2021, 55, 12741–12754 CAS.
- J. Li, X. Liu, H. Wang, Y. Sun and F. Dong, Prediction and interpretation of photocatalytic NO removal on g-C3N4-based catalysts using machine learning, Chin. Chem. Lett., 2024, 35, 108596 CrossRef CAS.
-
B. J. Finlayson-Pitts and J. N. Pitts, Chemistry of the Upper and Lower Atmosphere: Theory, Experiments, and Applications, 1999 Search PubMed.
- Y. Cao, Q. Ma, B. Chu and H. He, Homogeneous and heterogeneous photolysis of nitrate in the atmosphere: state of the science, current research needs, and future prospects, Front. Environ. Sci. Eng., 2023, 17(4), 48 CrossRef CAS.
- J. Schuttlefield, G. Rubasinghege, M. El-Maazawi, J. Bone and V. H. Grassian, Photochemistry of adsorbed nitrate, J. Am. Chem. Soc., 2008, 130, 12210 CrossRef CAS PubMed.
- X. L. Zhou, H. L. Gao, Y. He, G. Huang, S. B. Bertman, K. Civerolo and J. Schwab, Nitric acid photolysis on surfaces in low-NOx environments: Significant atmospheric implications, Geophys. Res. Lett., 2003, 30, 2217 Search PubMed.
- H. Wang, Y. Sun and F. Dong, Insight into the Overlooked Photochemical Decomposition of Atmospheric Surface Nitrates Triggered by Visible Light, Angew. Chem., Int. Ed., 2022, 61, e202209201 CrossRef CAS.
- Q. W. Shi, Y. Tao, J. E. Krechmer, C. L. Heald, J. G. Murphy, J. H. Kroll and Q. Ye, Laboratory Investigation of Renoxification from the Photolysis of Inorganic Particulate Nitrate, Environ. Sci. Technol., 2021, 55, 854–861 CrossRef CAS PubMed.
- C. Ye, X. Zhou, D. Pu, J. Stutz, J. Festa, M. Spolaor, C. Tsai, C. Cantrell, R. L. Mauldin III, T. Campos, A. Weinheimer, R. S. Hornbrook, E. C. Apel, A. Guenther, L. Kaser, B. Yuan, T. Karl, J. Haggerty, S. Hall, K. Ullmann, J. N. Smith, J. Ortega and C. Knote, Rapid cycling of reactive nitrogen in the marine boundary layer, Nature, 2016, 532, 489–491 CrossRef CAS PubMed.
- H. Schwartz-Narbonne, S. H. Jones and D. J. Donaldson, Indoor Lighting Releases Gas Phase Nitrogen Oxides from Indoor Painted Surfaces, Environ. Sci. Technol. Lett., 2019, 6, 92–97 CrossRef CAS.
- F. Karagulian, C. Santschi and M. J. Rossi, The heterogeneous chemical kinetics of N2O5 on CaCO3 and other atmospheric mineral dust surrogates, Atmos. Chem. Phys., 2006, 6, 1373–1388 CrossRef CAS.
- M. Ndour, P. Conchon, B. D’Anna, O. Ka and C. George, Photochemistry of mineral dust surface as a potential atmospheric renoxification process, Geophys. Res. Lett., 2009, 36, L05816 CrossRef.
- Y. Bedjanian and A. El Zein, Interaction of NO2 with TiO2 Surface Under UV Irradiation: Products Study, J. Phys. Chem. A, 2012, 116, 1758–1764 CrossRef CAS PubMed.
- G. Rubasinghege and V. H. Grassian, Photochemistry of Adsorbed Nitrate on Aluminum Oxide Particle Surfaces, J. Phys. Chem. A, 2009, 113, 7818–7825 CrossRef CAS.
- Y. Liu, X. Wang, J. Shang, W. Xu, M. Sheng and C. Ye, The positive effect of formaldehyde on the photocatalytic renoxitication of nitrate on TiO2 particles, Atmos. Chem. Phys., 2022, 22, 11347–11358 CrossRef CAS.
- M. S. Rosseler, V. Nahuel Montesinos, A. Shavorskiy, V. Keller, N. Keller, M. I. Litter, H. Bluhm, M. Salmeron and H. Destaillats, Chemistry of NOx on TiO2 Surfaces Studied by Ambient Pressure XPS: Products, Effect of UV Irradiation, Water, and
Coadsorbed K+, J. Phys. Chem. Lett., 2013, 4, 536–541 CrossRef.
- Q. Ma, C. Zhong, J. Ma, C. Ye, Y. Zhao, Y. Liu, P. Zhang, T. Chen, C. Liu, B. Chu and H. He, Comprehensive Study about the Photolysis of Nitrates on Mineral Oxides, Environ. Sci. Technol., 2021, 55, 8604–8612 CrossRef CAS.
- H. Shang, Z. Chen, X. Wang, M. Li, H. Li, C. Mao, L. Yu, J. Sun, Z. Ai and L. Zhang, SO2-enhanced nitrate photolysis on TiO2 minerals: A vital role of photochemically reactive holes, Appl. Catal., B, 2022, 308, 121217 CrossRef CAS.
- H. Wang, K. Li, J. Li, Y. Sun and F. Dong, Photochemical Transformation Pathways of Nitrates from Photocatalytic NOx Oxidation: Implications for Controlling Secondary Pollutants, Environ. Sci. Technol. Lett., 2021, 8, 873–877 CrossRef CAS.
- M. S. Gen, R. F. Zhang, D. D. Huang, Y. J. Li and C. K. Chan, Heterogeneous Oxidation of SO2 in Sulfate Production during Nitrate Photolysis at 300 nm: Effect of pH, Relative Humidity, Irradiation Intensity, and the Presence of Organic Compounds, Environ. Sci. Technol., 2019, 53, 8757–8766 CrossRef CAS.
- J. N. Pitts, A. M. Winer, R. Atkinson and W. P. Carter, Comment on “Effect of nitrogen oxide emissions on ozone levels in metropolitan regions”, “Effect of nitrogen oxide (NOx) emission rates on smog formation in the California South Coast Air Basin”, and “Effect of hydrocarbon and nitrogen oxide (NOx) on photochemical smog formation under simulated transport conditions, Environ. Sci. Technol., 1983, 17, 54–57 CrossRef CAS PubMed.
- Y. Qiu, Z. Ma, K. Li, W. Lin, Y. Tang, F. Dong and H. Liao, Markedly Enhanced Levels of Peroxyacetyl Nitrate (PAN) During COVID-19 in Beijing, Geophys. Res. Lett., 2020, 47, e2020GL089623 CrossRef CAS.
- C. Li, Q. Zhu, X. Jin and R. C. Cohen, Elucidating Contributions of Anthropogenic Volatile Organic Compounds and Particulate Matter to Ozone Trends over China, Environ. Sci. Technol., 2022, 12906–12916 CrossRef CAS.
- A. Mellouki, T. J. Wallington and J. Chen, Atmospheric Chemistry of Oxygenated Volatile Organic Compounds: Impacts on Air Quality and Climate, Chem. Rev., 2015, 115, 3984–4014 CrossRef CAS PubMed.
- Z. Meng, D. Dabdub and J. H. Seinfeld, Chemical coupling between atmospheric ozone and particulate matter, Science, 1997, 277, 116–119 CrossRef CAS.
- Z. Chen, H. Yin, C. Wang, R. Wang, Y. Peng, C. You and J. Li, New Insights on Competitive Adsorption of NO/SO2 on TiO2 Anatase for Photocatalytic NO Oxidation, Environ. Sci. Technol., 2021, 55, 9285–9292 CrossRef CAS PubMed.
- T. Xue, L. Chen, K. Li, B. Lei, H. Wang, F. Dong and Y. Yang, Highly Enhanced Photocatalytic NO Removal and Inhibited Peroxyacetyl Nitrate Formation in Synergistic Acetaldehyde Degradation, Environ. Sci. Technol., 2023, 57, 8174–8182 CrossRef CAS PubMed.
- K. Li, H. Wang, L. Chen, J. Li and F. Dong, Synergistic degradation of NO and C7H8 for inhibition of O3 generation, Appl. Catal., B, 2022, 312, 121423 CrossRef CAS.
- K. Li, T. Xue, L. Chen, J. Li, F. Dong and Y. Sun, Dual function of H2O on interfacial intermediate conversion and surface poisoning regulation in simultaneous photodegradation of NO and toluene, Environ. Res., 2024, 240, 117526 CrossRef CAS PubMed.
- X. Wang, A. Mahmood, G. Lu, X. Xie and J. Sun, Synergistic photocatalytic treatment of aromatic hydrocarbons/NOx mixtures over TiO2: The activation and replenishment of lattice oxygen, Chem. Eng. J., 2022, 450, 138168 CrossRef CAS.
- C. H. Ao, S. C. Lee, S. C. Zou and C. L. Mak, Inhibition
effect of SO2 on NOx and VOCs during the photodegradation of synchronous indoor air pollutants at parts per billion (ppb) level by TiO2, Appl. Catal., B, 2004, 49, 187–193 CrossRef CAS.
- M. Anpo, S. G. Zhang, H. Mishima, M. Matsuoka and H. Yamashita, Design of photocatalysts encapsulated within the zeolite framework and cavities for the decomposition of NO into N2 and O2 at normal temperature, Catal. Today, 1997, 39, 159–168 CrossRef CAS.
- J. L. Zhang, Y. Hu, M. Matsuoka, H. Yamashita, M. Minagawa, H. Hidaka and M. Anpo, Relationship between the local structures of titanium oxide photocatalysts and their reactivities in the decomposition of NO, J. Phys. Chem. B, 2001, 105, 8395–8398 CrossRef CAS.
- M. Anpo, M. Matsuoka, H. Yamashita, W. S. Ju, S. E. Park and Y. G. Shul, Photocatalytic decomposition of NO on transition metal ion-exchanged zeolite catalysts, J. Ind. Eng. Chem., 2000, 6, 133–143 CAS.
- Y. Hu, S. Higashimoto, G. Martra, J. L. Zhang, M. Matsuoka, S. Coluccia and M. Anpo, Local structures of active sites on Ti-MCM-41 and their photocatalytic reactivity for the decomposition of NO, Catal. Lett., 2003, 90, 161–163 CrossRef CAS.
- Y. Hu, G. Martra, J. L. Zhang, S. Higashimoto, S. Coluccia and M. Anpo, Characterization of the local structures of Ti-MCM-41 and their photocatalytic reactivity for the decomposition of NO into N2 and O2, J. Phys. Chem. B, 2006, 110, 1680–1685 CrossRef CAS PubMed.
- P. Y. Dong, N. Xu, Y. Xu and X. F. Wang, A study of Pt/WO3-carrier catalysts for photocatalytic purification of NO gas, Catal. Commun., 2016, 84, 142–146 CrossRef CAS.
- N. Bowering, G. S. Walker and P. G. Harrison, Photocatalytic decomposition and reduction reactions of nitric oxide over Degussa P25, Appl. Catal., B, 2006, 62, 208–216 CrossRef CAS.
- M. Anpo, M. Takeuchi, K. Ikeue and S. Dohshi, Design and development of titanium oxide photocatalysts operating under visible and UV light irradiation.: The applications of metal ion-implantation techniques to semiconducting TiO2 and Ti/zeolite catalysts, Curr. Opin. Solid State Mater. Sci., 2002, 6, 381–388 CrossRef CAS.
- M. Anpo and M. Takeuchi, Design and development of second-generation titanium oxide photocatalysts to better our environment-approaches in realizing the use of visible light, Int. J. Photoenergy, 2001, 3, 90–94 CrossRef.
- M. Xu, Y. Wang, J. Geng and D. Jing, Photodecomposition of NOx on Ag/TiO2 composite catalysts in a gas phase reactor, Chem. Eng. J., 2017, 307, 181–188 CrossRef CAS.
- Q. Wu and R. van de Krol, Selective Photoreduction of Nitric Oxide to Nitrogen by Nanostructured TiO2 Photocatalysts: Role of Oxygen Vacancies and Iron Dopant, J. Am. Chem. Soc., 2012, 134, 9369–9375 CrossRef CAS PubMed.
- M. Anpo, T.-H. Kim and M. Matsuoka, The design of Ti-, V-, Cr-oxide single-site catalysts within zeolite frameworks and their photocatalytic reactivity for the decomposition of undesirable molecules—The role of their excited states and reaction mechanisms, Catal. Today, 2009, 142, 114–124 CrossRef CAS.
- R. Hailili, Z. Q. Wang, H. W. Ji, C. C. Chen, X. Q. Gong, H. Sheng and J. C. Zhao, Mechanistic insights into the photocatalytic reduction of nitric oxide to nitrogen on oxygen-deficient quasi-two-dimensional bismuth-based perovskites, Environ. Sci.: Nano, 2022, 9, 1453–1465 RSC.
- L. Han, S. Cai, M. Gao, J.-Y. Hasegawa, P. Wang, J. Zhang, L. Shi and D. Zhang, Selective Catalytic Reduction of NOx with NH3 by Using Novel Catalysts: State of the Art and Future Prospects, Chem. Rev., 2019, 119, 10916–10976 CrossRef CAS PubMed.
- D. Wang, Q. Chen, X. Zhang, C. Gao, B. Wang, X. Huang, Y. Peng, J. Li, C. Lu and J. Crittenden, Multipollutant Control (MPC) of Flue Gas from Stationary Sources Using SCR Technology: A Critical Review, Environ. Sci. Technol., 2021, 55, 2743–2766 CrossRef CAS PubMed.
- X. Li, X. Yan, S. Zuo, X. Lu, S. Luo, Z. Li, C. Yao and C. Ni, Construction of LaFe1−xMnxO3/attapulgite nanocomposite for photo-SCR of NOx at low temperature, Chem. Eng. J., 2017, 320, 211–221 CrossRef CAS.
- L. Ma, J. Li, Y. Cheng, C. K. Lambert and L. Fu, Propene Poisoning on Three Typical Fe-zeolites for SCR of NOx with NH3: From Mechanism Study to Coating Modified Architecture, Environ. Sci. Technol., 2012, 46, 1747–1754 CrossRef CAS PubMed.
- K. Teramura, T. Tanaka, S. Yamazoe, K. Arakaki and T. Funabiki, Kinetic study of photo-SCR with NH3 over TiO2, Appl. Catal., B, 2004, 53, 29–36 CrossRef CAS.
- T. Tanaka, K. Teramura, T. Yamamoto, S. Takenaka, S. Yoshida and T. Funabiki, TiO2/SiO2 photocatalysts at low levels of loading: preparation, structure and photocatalysis, J. Photochem. Photobiol., A, 2002, 148, 277–281 CrossRef CAS.
- Y.-C. Chou and Y. Ku, Preparation of high-aspect-ratio TiO2 nanotube arrays for the photocatalytic reduction of NO in air streams, Chem. Eng. J., 2013, 225, 734–743 CrossRef CAS.
- R. Yuan, M. Wang, L. Liao, W. Hu, Z. Liu, Z. Liu, L. Guo, K. Li, Y. Cui, F. Lin, F. Tao and W. Zhou, 100% N2O inhibition in photocatalytic NOx reduction by carbon particles over Bi2WO6/TiO2 Z-scheme heterojunctions, Chem. Eng. J., 2023, 453, 139892 CrossRef CAS.
- Y. Ren, Q. Han, J. Yang, Y. Zhao, Y. Xie, H. Wen and Z. Jiang, A promising catalytic solution of NO reduction by CO using g-C3N4/TiO2: A DFT study, J. Colloid Interface Sci., 2022, 610, 152–163 CrossRef CAS PubMed.
- J. Lasek, Y.-H. Yu and J. C. S. Wu, Water and temperature effects on photo-selective catalytic reduction of nitric oxide on Pd-loaded TiO2 photocatalyst, Environ. Technol., 2012, 33, 2133–2141 CrossRef CAS PubMed.
- R. Jin, Z. Wu, Y. Liu, B. Jiang and H. Wang, Photocatalytic reduction of NO with NH3 using Si-doped TiO2 prepared by hydrothermal method, J. Hazard. Mater., 2009, 161, 42–48 CrossRef CAS PubMed.
- S. Yamazoe, T. Okumura, K. Teramura and T. Tanaka, Development of the efficient TiO2 photocatalyst in photoassisted selective catalytic reduction of NO with NH3, Catal. Today, 2006, 111, 266–270 CrossRef CAS.
- S. Yamazoe, Y. Masutani, T. Shishido and T. Tanaka, Metal oxide promoted TiO2 catalysts for photo-assisted selective catalytic reduction of NO with NH3, Res. Chem. Intermed., 2008, 34, 487–494 CrossRef CAS.
- T. Tanaka, K. Teramura, K. Arakaki and T. Funabiki, Photoassisted NO reduction with NH3 over TiO2 photocatalyst, Chem. Commun., 2002, 2742–2743 RSC.
- J. Papp, S. Soled, K. Dwight and A. Wold, Surface Acidity and Photocatalytic Activity of TiO2, WO3/TiO2, and MoO3/TiO2 Photocatalysts, Chem. Mater., 1994, 6, 496–500 CrossRef CAS.
- S. Yamazoe, Y. Masutani, K. Teramura, Y. Hitomi, T. Shishido and T. Tanaka, Promotion effect of tungsten oxide on photo-assisted selective catalytic reduction of NO with NH3 over TiO2, Appl. Catal., B, 2008, 83, 123–130 CrossRef CAS.
- S. Yamazoe, Y. Masutani, T. Shishido and T. Tanaka, XAFS study of active tungsten species on WO3/TiO2 as a catalyst for photo-SCR, EXAFS Spectrosc.: Tech. Appl., Proc. Symp. Appl. EXAFS Mater. Sci., 2007, 696 CAS.
- A. Yamamoto, K. Teramura and T. Tanaka, Selective Catalytic Reduction of NO by NH3 over Photocatalysts (Photo-SCR): Mechanistic Investigations and Developments, Chem. Rec., 2016, 16, 2268–2277 CrossRef CAS PubMed.
- Y. Ji, B. Ding, W. Ni, X. Li, X. He, Z. Chen, S. Ran and H. Lü, Tailoring the crystal structure of CaTiO3 by multielement doping for photo-assisted activation of NO, Chem. Eng. J., 2022, 450, 138255 CrossRef CAS.
- K. Wei, X. Yan, S. Zuo, W. Zhu, F. Wu, X. Li, C. Yao and X. Liu, Photo-assisted Catalytic Removal of NOx Over La1–xPrxCoO3/Palygorskite Nanocomposites: Role of Pr Doping, Clays Clay Miner., 2019, 67, 348–356 CrossRef CAS.
- X. Li, H. Shi, W. Zhu, S. Zuo, X. Lu, S. Luo, Z. Li, C. Yao and Y. Chen, Nanocomposite LaFe1-xNixO3/Palygorskite catalyst for photo-assisted reduction of NOx: Effect of Ni doping, Appl. Catal., B, 2018, 231, 92–100 CrossRef CAS.
- X. Li, H. Shi, X. Yan, S. Zuo, Y. Zhang, T. Wang, S. Luo, C. Yao and C. Ni, Palygorskite Immobilized Direct Z-Scheme Nitrogen-Doped Carbon Quantum dots/PrFeO3 for Photo-SCR Removal of NOx, ACS Sustain. Chem. Eng., 2018, 6, 10616–10627 CrossRef CAS.
- L. Chen, D. Shen, B. Li, Z. Xiao, W. Sun, X. Liu, J. Ma, C. Li and W. Wang, The efficient electrocatalytic and photocatalytic reduction of nitric oxide into ammonia over 0D/3D g-C3N4 quantum dots/3DOMM-TiO2-x heterojunction, Ceram. Int., 2023, 49, 23129–23139 CrossRef CAS.
- L. Ju, X. Tang, Y. Zhang, X. Li, X. Cui and G. Yang, Single Selenium Atomic Vacancy Enabled Efficient Visible-Light-Response Photocatalytic NO Reduction to NH3 on Janus WSSe Monolayer, Molecules, 2023, 28(7), 2959 CrossRef CAS PubMed.
- D. Yao, C. Tang, P. Wang, H. Cheng, H. Jin, L.-X. Ding and S.-Z. Qiao, Electrocatalytic green ammonia production beyond ambient aqueous nitrogen reduction, Chem. Eng. Sci., 2022, 257, 117735 CrossRef CAS.
- I. G. Zacharia and W. M. Deen, Diffusivity and Solubility of Nitric Oxide in Water and Saline, Ann. Biomed. Eng., 2005, 33, 214–222 CrossRef.
- J. Li, J. Wang, S. Shen, R. Chen, M. Liu and F. Dong, Beyond Purification: Highly Efficient and Selective Conversion of NO into Ammonia by Coupling Continuous Absorption and Photoreduction under Ambient Conditions, Environ. Sci. Technol., 2023, 57, 5445–5452 CrossRef CAS PubMed.
- W. Chen, R. Zou and X. Wang, Toward an Atomic-Level Understanding of the Catalytic Mechanism of Selective Catalytic Reduction of NOx with NH3, ACS Catal., 2022, 12, 14347–14375 CrossRef CAS.
- Z. Si, Y. Shen, J. He, T. Yan, J. Zhang, J. Deng and D. Zhang, SO2-Induced Alkali Resistance of FeVO4/TiO2 Catalysts for NOx Reduction, Environ. Sci. Technol., 2022, 56, 605–613 CrossRef CAS PubMed.
- R. Chen, J. Li, J. Wang, W. Yang, S. Shen and F. Dong, Continuous NO Upcycling into Ammonia Promoted by SO2 in Flue Gas: Poison Can Be a Gift, Environ. Sci. Technol., 2023, 57, 12127–12134 CrossRef CAS PubMed.
- W. Yang, J. Wang, R. Chen, L. Xiao, S. Shen, J. Li and F. Dong, Reaction mechanism and selectivity regulation of photocatalytic nitrate reduction for wastewater purification: progress and challenges, J. Mater. Chem. A, 2022, 10, 17357–17376 RSC.
- H. Li, H. Zhu, Y. Shi, H. Shang, L. Zhang and J. Wang, Vacancy-Rich and Porous
NiFe-Layered Double Hydroxide Ultrathin Nanosheets for Efficient Photocatalytic NO Oxidation and Storage, Environ. Sci. Technol., 2022, 56, 1771–1779 CrossRef CAS.
- H. Hirakawa, M. Hashimoto, Y. Shiraishi and T. Hirai, Selective Nitrate-to-Ammonia Transformation on Surface Defects of Titanium Dioxide Photocatalysts, ACS Catal., 2017, 7, 3713–3720 CrossRef CAS.
- H. S. Moon, B. Song, J. Jeon, T.-H. Lai, Y.-P. Chang, Y.-D. Lin, J. K. Park, Y.-G. Lin, Y.-J. Hsu, H. Shin, Y. Yun and K. Yong, Atomically isolated copper on titanium dioxide for ammonia photosynthesis via nitrate reduction with unprecedently high apparent quantum yield, Appl. Catal., B, 2023, 339, 123185 CrossRef CAS.
- J. Li, R. Chen, J. Wang, K. Wang, Y. Zhou, M. Xing and F. Dong, Dynamic in situ Formation of Cu2O Sub-Nanoclusters through Photoinduced pseudo-Fehling’s Reaction for Selective and Efficient Nitrate-to-Ammonia Photosynthesis, Angew. Chem., Int. Ed., 2024, 63, e202317575 CrossRef CAS PubMed.
- R. Chen, S. Shen, K. Wang, J. Wang, W. Yang, X. Li, J. Li and F. Dong, Promoting the efficiency and selectivity of NO3--to-NH3 reduction on Cu-O-Ti active sites via preferential glycol oxidation with holes, Proc. Natl. Acad. Sci. U.S.A., 2023, 120, e2312550120 CrossRef CAS PubMed.
- J. Li, R. Chen, J. Wang, Y. Zhou, G. Yang and F. Dong, Subnanometric alkaline-earth oxide clusters for sustainable nitrate to ammonia photosynthesis, Nat. Commun., 2022, 13, 1098 CrossRef CAS PubMed.
- A. Sanchez, Z. Ye, Y. Yin and H. Liu, Photochemical conversion of nitrate to ammonium ions by a newly developed photo-reductive titanium dioxide catalyst: implications on nitrogen recovery, Environ. Sci.: Water Res. Technol., 2023, 9, 3318–3324 RSC.
- L. Wei, Y. Zhang, C. Zhang, C. Yao, C. Ni and X. Li, In Situ Growth of Perovskite on 2D Hydrothermal Carbonation Carbon for Photocatalytic Reduction of Nitrate to Ammonia, ACS Appl. Nano Mater., 2023, 6, 13127–13136 CrossRef.
- W. Liu, X. Li, X. Chu, S. Zuo, B. Gao, C. Yao, Z. Li and Y. Chen, Boosting photocatalytic reduction of nitrate to ammonia enabled by perovskite/biochar nanocomposites with oxygen defects and O-containing functional groups, Chemosphere, 2022, 294, 133763 CrossRef CAS PubMed.
- Y. Wang, H. Yin, X. Zhao, Y. Qu, A. Zheng, H. Zhou, W. Fang and J. Li, Photocatalytic ammonia synthesis from nitrate reduction on nickel single-atom decorated on defective tungsten oxide, Appl. Catal., B, 2024, 341, 123266 CrossRef CAS.
- D. Hao, Y. Wei, L. Mao, X. Bai, Y. Liu, B. Xu, W. Wei and B.-J. Ni, Boosted selective catalytic nitrate reduction to ammonia on carbon/bismuth/bismuth oxide photocatalysts, J. Cleaner Prod., 2022, 331, 129975 CrossRef CAS.
- R. Kato, M. Furukawa, I. Tateishi, H. Katsumata and S. Kaneco, Novel Photocatalytic NH3 Synthesis by NO3- Reduction over CuAg/TiO2, Chem. Eng., 2019, 3(2), 49 CAS.
- L. Wei, M. A. S. Adamson and J. Vela, Ni2P-Modified Ta3N5 and TaON for Photocatalytic Nitrate Reduction, ChemNanoMat, 2020, 6, 1179–1185 CrossRef CAS.
- N. Tong, Y. Wang, Y. Liu, M. Li, Z. Zhang, H. Huang, T. Sun, J. Yang, F. Li and X. Wang, PdSn/NiO/NaTaO3: La for photocatalytic ammonia synthesis by reduction of NO3- with formic acid in aqueous solution, J. Catal., 2018, 361, 303–312 CrossRef CAS.
- M. Yamauchi, R. Abe, T. Tsukuda, K. Kato and M. Takata, Highly Selective Ammonia Synthesis from Nitrate with Photocatalytically Generated Hydrogen on CuPd/TiO2, J. Am. Chem. Soc., 2011, 133, 1150–1152 CrossRef CAS PubMed.
|
This journal is © The Royal Society of Chemistry 2024 |
Click here to see how this site uses Cookies. View our privacy policy here.