DOI:
10.1039/D4SC01915F
(Edge Article)
Chem. Sci., 2024,
15, 12431-12441
A de novo zwitterionic strategy of ultra-stable chemiluminescent probes: highly selective sensing of singlet oxygen in FDA-approved phototherapy†
Received
22nd March 2024
, Accepted 10th June 2024
First published on 24th June 2024
Abstract
Singlet oxygen (1O2), as a fundamental hallmark in photodynamic therapy (PDT), enables ground-breaking clinical treatment in ablating tumors and killing germs. However, accurate in vivo monitoring of 1O2 remains a significant challenge in probe design, with primary difficulties arising from inherent photo-induced side reactions with poor selectivity. Herein, we report a generalizable zwitterionic strategy for ultra-stable near-infrared (NIR) chemiluminescent probes that ensure a highly specific [2 + 2] cycloaddition between fragile electron-rich enolether units and 1O2 in both cellular and dynamic in vivo domains. Innovatively, zwitterionic chemiluminescence (CL) probes undergo a conversion into an inert ketone excited state with an extremely short lifetime through conical intersection (CI), thereby affording sufficient photostability and suppressing undesired photoreactions. Remarkably, compared with the well-known commercial 1O2 probe SOSG, the zwitterionic probe QMI exhibited an ultra-high signal-to-noise ratio (SNR, over 40-fold). Of particular significance is that the zwitterionic CL probes demonstrate excellent selectivity, high sensitivity, and outstanding photostability, thereby making a breakthrough in real-time tracking of the FDA-approved 5-ALA-mediated in vivo PDT process in living mice. This innovative zwitterionic strategy paves a new pathway for high-performance NIR chemiluminescent probes and high-fidelity feedback on 1O2 for future biological and medical applications.
Introduction
Singlet oxygen (1O2), a highly reactive oxygen species (ROS), has the remarkable capacity to induce oxidative stress in cells.1–3 This striking property of 1O2 has enabled ground-breaking clinical treatments for ablating tumors and killing germs.4–10 The quantification of 1O2 in photodynamic therapy (PDT) is the dominant feature as a dependable gauge of therapeutic effect, which not only bestows immediate feedback on treatment but also confers optimal therapy protocols.11,12 However, the progress in monitoring cellular 1O2 has been seriously impeded by the inability to extrapolate an effective solution for the probe. The exceedingly short lifetime of 1O2 (<1 μs),13 coupled with interference from other ROS in tumor tissues,14 makes it extremely difficult to precise 1O2 detection across both cellular and dynamic in vivo domains. To date, the gold standard for 1O2 determination relies upon the rigorous instrument for feeble phosphorescence signals at 1270 nm and thereby is merely utilized for in vitro application.15 Hence, there is an urgent demand to develop a design strategy for highly sensitive and selective probes for the in vivo detection of singlet oxygen.
The features of optical probes hold great potential for revolutionizing the accurate in vivo detection of singlet oxygen.16–21 In general, current 1O2 probes predominantly rely on strong oxidative reactions, thereby inability to discriminate 1O2 from stronger oxidative ROS species, such as commercial 9,10-anthracenediyl-bis(methylene)-dimalonic acid (ABDA).22 Most importantly, the contradiction between an undesirable photo-induced reaction and imperative light irradiation makes it seriously difficult to search for an effective solution for the in vivo1O2 probe (Fig. 1A). For instance, the well-known Singlet Oxygen Sensor Green (SOSG, a commercial probe) suffers from its self-sensitization, leading to inevitable overestimations of 1O2.23–25 Indeed, the [2 + 2] cycloaddition reactions between the enolether and 1O2 in chemiluminescence (CL) provide an effective solution to discriminate 1O2 from other ROS,26–29 but the highly electron-rich enolether moiety is generally fragile under light irradiation,30–32 thereby inevitably leading to undesirable photo-induced side reactions.33,34 Furthermore, near-infrared chemiluminescent emission is essential to improving tissue in vivo penetration.35–38 Thus, the elaborate strategy of converting photo-active electron-rich enolether into a photo-inert state would make a breakthrough in 1O2 probe solution for ultra-stable NIR chemiluminescent probes.
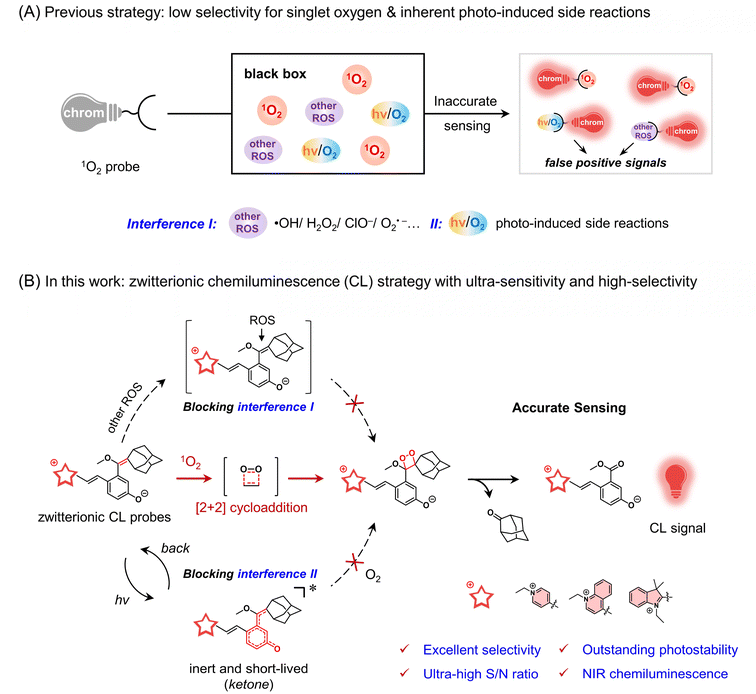 |
| Fig. 1 Zwitterionic chemiluminescence (CL) strategy enables accurate sensing of 1O2. (A) Previous optical probes for sensing 1O2 with low selectivity and photo-induced side reactions inevitably produce misleading responses. (B) In our strategy, zwitterionic ultra-stable CL probes for accurately sensing 1O2 are specifically triggered by the [2 + 2] cycloaddition reaction between the enolether moiety and 1O2; the generation of inert and short-lived ketone excited states of zwitterionic probes enables the effective suppression of photo-induced side reactions. | |
Herein, we present an innovative zwitterionic strategy to construct ultra-stable NIR chemiluminescent probes, which covalently bridge cationic chromophores with a phenolic dioxetane precursor via a short methine chain (Fig. 1B). The unique zwitterionic configuration endows the probes with the following key features: Firstly, the “merocyanine configuration” extends the luminescence of these probes into the NIR region. Secondly, the highly specific [2 + 2] cycloaddition reaction of the enolether moiety in the probe with 1O2 endows excellent selectivity. The subsequent cycloadducts producing bright chemiluminescence ensure the ultra-high sensitivity of the probes towards 1O2. Thirdly, most importantly, the photo-inert ketone excited state of the zwitterionic probe, coupled with its extremely short excited-state lifetime, could effectively mitigate undesired photo-induced side reactions. Compared to the reported CL probes and commercial SOSG, these zwitterionic probes exhibit remarkable photostability and superior sensitivity towards 1O2. Notably, for the first time, utilizing the ultra-stable zwitterionic CL probes allows us to accurately in vivo monitor 1O2 from the FDA-approved 5-aminolevulinic acid (5-ALA)-mediated PDT process in living mice. This zwitterionic ultra-stable CL strategy opens avenues for a new type of in vivo1O2 probe.
Results and discussions
Exploring the zwitterionic design strategy for CL probes
As mentioned, insufficient selectivity, low sensitivity, and inherent photo-induced side reactions become the bottlenecks for 1O2 optical probes. In addressing these challenges, the enolether unit, a recognized chemiluminescent building block that imparts chemical energy by the specific [2 + 2] cycloaddition reaction with 1O2,39–41 was employed as a specific recognition unit for 1O2. However, constrained by the electron-rich nature of the enolether unit, these chemiluminophores are prone to photo-oxidation reactions under light irradiation, leading to undesirable photodegradation and photo-induced CL signals.
To address this dilemma between structural requirements and photostability, we turn our attention to merocyanine with zwitterionic and quinoid forms, which possess the distinctive feature of conical intersection (CI) between the ground and excited-state potential energy surfaces.42 The presence of CI could greatly shorten the excited-state lifetime of merocyanine, thereby effectively suppressing self-oxidation reactions. Consequently, we engineered three zwitterionic CL probes (PyCL, QMI, and InCL) featuring “merocyanine configuration” by bridging three cationic chromophores (N-ethylpyridine, N-ethylquinoline, and N-ethylindole) with the phenolic luminophore containing enolether at the para position, respectively (Fig. 2A and Scheme S1†). We anticipated that the zwitterionic probes would exhibit significant photostability under light irradiation, thus maintaining a photo-inert response in the chemiluminescent channel.
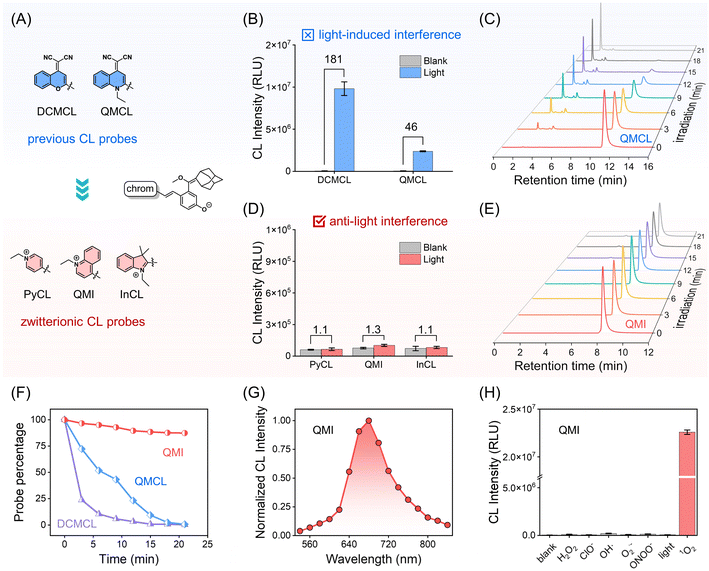 |
| Fig. 2 Construction of ultra-stable and highly selective chemiluminescent probes for singlet oxygen based on a zwitterionic strategy. (A) Chemical structures of previous and zwitterionic CL probes. (B) The light-induced interference of previous CL probes with potential photooxidation reaction upon white light (120 mW cm−2) irradiation for 1 min. (C) Time-resolved HPLC profiles of QMCL under light irradiation (white light, 1.6 W cm−2; bubbling oxygen into the solution). (D) Anti-light interference of zwitterionic CL probes with outstanding photostability upon white light (120 mW cm−2) irradiation for 1 min. (E) Time-resolved HPLC profiles of QMI under the same test conditions as (C). (F) Quantification of DCMCL, QMCL, and QMI with light irradiation in HPLC. (G) Chemiluminescence spectrum of QMI. (H) CL signal intensity of QMI (50 μM) towards 1O2 (produced by 10 μM methylene blue), light irradiation (2 min), and other ROS (50 equiv.) in PBS buffer solution. Data with error bars are expressed as mean ± s.d., n = 3. | |
As shown in Fig. 2B, the CL signals of DCMCL and QMCL were observed with 181-fold and 46-fold enhancement under light irradiation, respectively. It was suggested that this enhancement should be ascribed to the photooxidation reaction. Furthermore, after light irradiation for 21 min, high-performance liquid chromatography (HPLC) experiments revealed that QMCL underwent rapid photodegradation, with up to a 99.4% degradation rate. It is noteworthy that the retention time of the decomposition product was merely 2.27 min, presumably due to the removal of the adamantane substituent (Fig. 2C and F). Similar fast decomposition was also found in the probe DCMCL (Fig. 2F and S1†).
Revealing ultra-stable and highly selective characteristics of zwitterionic CL probes towards singlet oxygen
For three zwitterionic probes (PyCL, QMI, and InCL), there were only neglectable CL signal changes under light irradiation (Fig. 2D), indicating the zwitterionic strategy is beneficial for the improvement of ultra-stability. Specifically, QMI displayed excellent photostability under the same light irradiation as QMCL (Fig. 2E and F). Moreover, high-resolution mass spectrometry (HRMS) further confirmed the decomposition process, which was produced in the chemiluminescent process under light irradiation (Fig. S1–S3†). In addition, the compared experiments of QMCL and zwitterionic CL probes in a mixture of PBS/DMSO with different PBS fractions (fw) verified that the zwitterionic CL probes have sufficient stability under light irradiation (Fig. S4 and S5†). Clearly, these zwitterionic CL probes ensure a significantly stable electron-rich enolether moiety, resulting in minimized photo-induced side effects with outstanding photostability.
Next, we investigated the photophysical properties of the designed zwitterionic CL probes. Obviously, compared with PyCL and InCL, the probe QMI has a longer emission, thereby making it better suitable for in vivo applications (Fig. S6 and S7†). Therefore, we chose QMI as a model for the accurate detection of 1O2in vivo. In fact, the pKa value (pKa = 6.8) implied that QMI was susceptible to deprotonation at the physiological pH condition (Fig. S8†). Similar to negative-solvatochromic dyes,43 QMI tends to perform an amphoteric configuration in a large polar solvent such as water (Fig. S9†). Then, the CL spectrum of QMI was observed extending into the NIR region (emission peak at 680 nm), which is suitable for in vivo applications (Fig. 2G).44
The selectivity of QMI toward reactive oxygen species (ROS) was carried out. As expected, only QMI towards 1O2 emits a remarkably enhanced CL signal, while other ROS or only light irradiation produce negligible responses (Fig. 2H). It means that the CL signal from QMI could only be specifically activated in the presence of 1O2. Collectively, our simple and generalizable zwitterionic design strategy endows CL probes with ultra-stability and excellent selectivity for 1O2 over other potential ROS.
Discovering the conical intersection mechanism of zwitterionic CL probes
Very recently, we demonstrated that the CL probes bring about reactive radical species under photoexcitation and then react with oxygen to generate 1,2-dioxetane, thereby initiating light-induced CL signals.34 Thus, we reasonably believed that the zwitterionic probes should manifest distinct photoexcited states to intercept reactions in the presence of oxygen (Fig. 3A). The remarkable photostability of zwitterionic probes motivates us to gain further insight into their intrinsic mechanism by utilizing time-dependent density functional theory (TD-DFT) calculations and femtosecond transient absorption spectroscopy (fs-TAS).
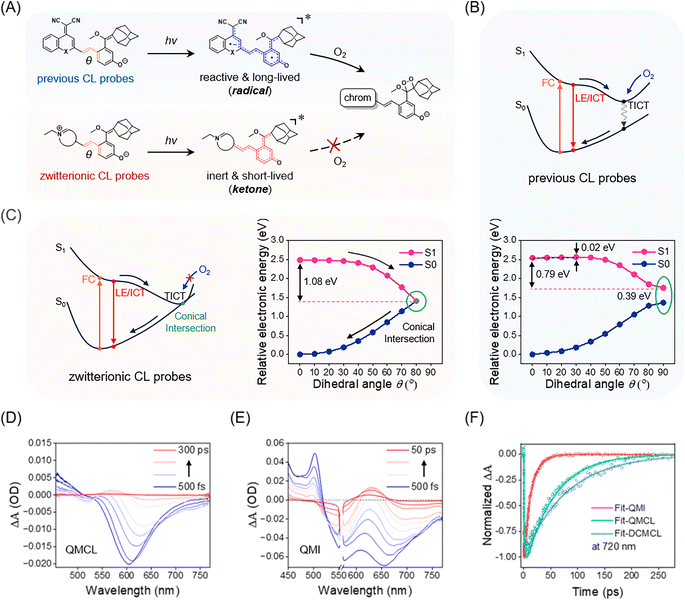 |
| Fig. 3 Insight into the conical intersection mechanism of zwitterionic probes to suppress photo-induced side reactions. (A) Previous CL probes become reactive and long-lived radical species upon photoexcitation, and then react with oxygen to form 1,2-dioxetane; zwitterionic CL probes turn into inert and short-lived ketone excited states upon photoexcitation and then rapidly return to ground states, thus suppressing photoreaction with oxygen. (B) Schematic illustration of previous CL probes. S1 (pink) and S0 (dark blue) potential energy surfaces of QMCL as a function of rotating angle (θ). Note: the dihedral angle (θ) is highlighted in orange in Fig. 3A. (C) Schematic illustration of ultra-stable zwitterionic CL probes. S1 (pink) and S0 (dark blue) potential energy surfaces of QMI as a function of rotating angle (θ). Transient absorption spectra of (D) QMCL (100 μM, λex = 450 nm) and (E) QMI (100 μM, λex = 560 nm) were obtained in a mixture of PBS/DMSO (v/v = 1/1). Note: in Fig. 3D, lines indicate transient absorption spectra at 500 fs, and 1, 2.5, 5, 10, 50, 100, 200, and 300 ps. In Fig. 3E, lines indicate transient absorption spectra at 500 fs, and 1, 2, 3, 5, 7.5, 10, 20, 30, and 50 ps. (F) Kinetic traces of QMI, QMCL, and DCMCL at 720 nm. The solid lines are the fitting curves with a multiexponential decay function. | |
TD-DFT calculation results indicated that QMCL (photoactivatable CL probe) has a small energy barrier (0.02 eV) and possesses a considerable driven force (0.79 eV), thereby entering the twisted intramolecular charge transfer (TICT) states upon photoexcitation (Fig. 3B). Simultaneously, the corresponding oscillator strength (f) decreased to zero, and a total charge separation was observed between the donor and acceptor fragments in the TICT state (Fig. S10†). Notably, the large optical gap (between the S1 and S0 states, 0.39 eV) of QMCL makes its TICT state have a long lifetime (the energy gap law). Consequently, the complete charge separation in the TICT state led to the formation of radical compounds. Thus, the highly reactive and long-lived TICT state ensured that QMCL reacted with oxygen to form 1,2-dioxetane, triggering subsequent chemiluminescence (Fig. 3A).
In contrast, TD-DFT calculation results for QMI revealed the occurrence of a conical intersection (CI) between the S1 and S0 states, so that the photochemical reaction between QMI and oxygen was efficiently inhibited. Specifically, as θ rotates from 0° to 90° to enter the TICT state of QMI (Fig. S10†), the optical gap (between the S1 and S0 states) quickly approaches zero, thereby resulting in a conical intersection (CI) between the S1 and S0 states (Fig. 3C). It should be noted that CI serves as a rapid non-radiative decay channel and leads to an extremely short-lived excited state.45,46 Furthermore, the smaller dipole moment (μ = 23.574 D) and the shorter C–O bond length (1.252 Å) of QMI (Fig. S11 and Table S1†) verified that the electron transfer from the phenoxy anion to the positively charged nitrogen atom brings QMI into the inert ketone form. Similar calculation results were also observed in the zwitterionic PyCL and InCL (Fig. S12 and S13†). Clearly, due to the formation of inert and short-lived excited species, these zwitterionic probes do not generate chemiluminescence upon light irradiation, thereby showing the ultra-stable characteristic.
Then, the fs-TAS was employed to validate the excited-state dynamics of QMI.47,48 Transient absorption spectra showed that QMI had a significantly shorter excited-state lifetime than that of QMCL and DCMCL (Fig. 3D, E and S14†). The stimulated emission at 720 nm was selected as a representative band to evaluate the excited-state lifetimes of the three compounds. As shown in Fig. 3F, the stimulated emission signal of QMI at 720 nm decayed rapidly, with a lifetime of 14 ps. In contrast, the stimulated emission decay of QMCL and DCMCL at 720 nm became significantly slower, with lifetimes of 62 ps and 80 ps, respectively. Moreover, in water solution, the stimulated emission lifetime of QMI was even shortened to 7 ps (Fig. S15†). Furthermore, the transient absorption spectra of QMI in acetonitrile solution provide solid evidence for the CI mechanism proposed in the TD-DFT calculations, which is consistent with the results for merocyanine in previous studies (Fig. S16†).46 All of these calculation results and experimental data agree with our design principle for ultra-stable and high-selective zwitterionic CL probes, that is, the generation of short-lived and inert ketone excited species impedes the potential undesirable photoreaction, thereby effectively eliminating the interference from photo-induced side reactions.
Verifying high-performance zwitterionic CL probes toward singlet oxygen over commercial probe SOSG
It still remains critical and challenging to track transient 1O2 with sufficient sensitivity in living organisms.49,50 We investigated whether the zwitterionic CL probe QMI could meet the demand for 1O2 for efficient signal transduction. As shown in Fig. 4A, through the [2 + 2] cycloaddition reaction, the electron-rich enolether specifically traps 1O2 to generate an unstable high-energy 1,2-dioxetane structure, and subsequent decomposition of the 1,2-dioxetane leads to NIR emission. This mechanism was confirmed by HPLC-MS analysis (Fig. S17 and S18†). It is worth noting that there was no significant change in the fluorescence emission spectra of QMI before and after the reaction with 1O2 (Fig. S19†), making it difficult to trace 1O2 by fluorescence. Based on this activated process, we evaluated the capability of QMI for sensing 1O2 produced by methylene blue (MB), a widely utilized photosensitizer (PS) for PDT of solid tumors. As expected, QMI exhibited a remarkable CL signal in response to 1O2 from MB under light irradiation, achieving an ultra-high S/N ratio (approximately 488-fold) (Fig. S20†) with a typical glow-type CL kinetic profile. In contrast, no CL signal was observed from QMI without light irradiation. The fact that the CL intensity increased linearly with the light irradiation time provided ultra-sensitive feedback on 1O2 generation by the photosensitizer MB. In addition, for other commercial photosensitizers such as rose bengal (RB) and porphyrins (chlorin e6, Ce6; hematoporphyrin monomethyl ether, HMME; protoporphyrin IX, PpIX), we also observed similar properties. Importantly, the CL signal intensity of QMI displayed an excellent linear relationship with the concentration of these photosensitizers (Fig. S21†). This observation underscored its capacity for accurately detecting the level of 1O2 produced by the photosensitizers.
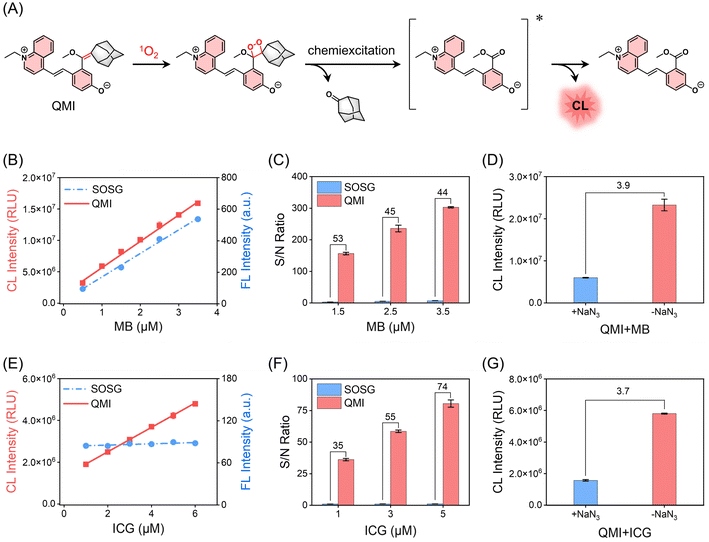 |
| Fig. 4 Zwitterionic CL probes enable ultra-sensitive detection of singlet oxygen. (A) Sensing mechanism of QMI as the 1O2 probe. Linear correlation between signal intensities of QMI/SOSG and concentrations of MB (B) or ICG (E). Comparison of the S/N ratios of QMI and SOSG at different concentrations of MB (C) or ICG (F). CL signal intensities of QMI + MB (D) and QMI + ICG (G) with NaN3 (1O2 scavenger, 1 mM). MB experiment: the mixed solution was exposed to a 690 nm laser (200 mW cm−2) for 1 min. ICG experiment: the mixed solution was exposed to an 808 nm laser (200 mW cm−2) for 3 min. Data with error bars are expressed as mean ± s.d., n = 3. | |
To demonstrate the ultra-sensitivity of QMI towards 1O2, two well-known commercial 1O2 probes, ABDA and SOSG, were selected as references. Herein, for the contrast experiments, photosensitizer MB was chosen as a typically efficient 1O2 generator. As shown in Fig. 4B, there was a good linear correlation (R2 = 0.996) between the CL intensity of QMI and the concentration of MB, indicating that the 1O2 generated by MB can be intuitively detected and quantified by CL signal changes. Similarly, commercial probe SOSG based on fluorescent changes was observed for the detection of 1O2 generated from MB. It was noteworthy that QMI had a significantly lower limit of detection (LOD) for 1O2 in comparison to SOSG (LODQMI = 4.3 nM, LODSOSG = 42.4 nM). Especially, the S/N ratio (SNR) of QMI towards 1O2 was much higher than that of SOSG at the various concentrations of MB (Fig. 4C). Additionally, SOSG has the propensity to self-sensitize, thus producing unexpected additional 1O2 under light irradiation. This inherent characteristic of SOSG inevitably gives rise to false positive signals, thereby providing misleading information (Fig. S22†). Another commercial probe, ABDA, based on absorbance intensity changes, displayed the lowest SNR under the same condition (Fig. S23†). Furthermore, the introduction of NaN3 (1O2-specific scavenger) led to a substantial 74% decrease in the CL intensity of QMI (Fig. 4D), serving as robust evidence that the specific [2 + 2] cycloaddition reaction between QMI and 1O2 initiated the CL signal change.
To further assess the sensitivity of zwitterionic probes for 1O2, we evaluated the capability of QMI to detect and trace the low-efficiency photosensitizers during PDT. Indocyanine green (ICG), an FDA-approved clinical reagent, was selected as a representative of low-efficiency photosensitizers, and was documented for PDT as early as 20 years ago.51,52 The photosensitive efficiency of ICG is ca. 7.7%, and it is difficult to be recognized by traditional probes due to its self-consumption of 1O2.53–55 Excitingly, QMI demonstrated a quantitative response to even subtle 1O2 produced by ICG (1 μM), achieving an impressive 35-fold SNR with a LOD as low as 30.9 nM (Fig. 4E and F). In contrast, under the same condition, there was negligible signal change for SOSG and ABDA, respectively (Fig. 4E and S23†). Following the addition of NaN3, the CL signal intensity of QMI decreased to 27% of the initial CL intensity, confirming that ICG sensitizes oxygen to produce certain 1O2 under light irradiation (Fig. 4G). In addition, the CL signal of QMI increased continuously with the extension of irradiation time at a given ICG concentration (Fig. S24†). Importantly, similar results for sensing 1O2 were also observed in the pentamethine cyanine dyes (Cy5) (Fig. S25†). Taken together, these results highlighted the remarkable advantages of QMI in terms of detection sensitivity and contrast ratio over commercial probes.
Lighting up endogenous singlet oxygen in living cells
The ultra-high sensitivity, excellent selectivity, and outstanding photostability of this zwitterionic CL probe QMI encouraged us to further evaluate its capability for intracellular 1O2. The cytotoxicity of QMI toward the HeLa cells was investigated by the methyl thiazolyl tetrazolium (MTT) assay. In the presence of various concentrations of QMI, the cell survival rate was estimated to be over 95% after incubation for 24 h, indicating its favorable biocompatibility (Fig. S26†). Then, the internalization of HMME (hematoporphyrin monomethyl ether, an exogenous porphyrin photosensitizer) was assessed by fluorescence confocal microscopy (Fig. S27†). After the incubation of cells with HMME, a distinct fluorescence characteristic was clearly observed within the cells, indicating its effective penetration of the cell membrane.
Subsequently, we validated the ability of QMI to visualize intracellular 1O2 generated by internalized HMME in living cells. Specifically, cells were incubated with QMI and HMME individually or in combination in 96-well plates. Due to the absence of 1O2 generation, no signals were detected in the dark groups. Similarly, groups incubated with only QMI or HMME did not emit CL signals even under light irradiation. In contrast, after being co-incubated with QMI and HMME together, an unambiguous CL signal was observed following light irradiation, suggesting that the zwitterionic probe QMI enables the sensing of intracellular 1O2 in living cells (Fig. S27†).
Furthermore, we utilized the zwitterionic probe QMI to track endogenous 1O2 produced during the cellular photosensitizing process. 5-Aminolevulinic acid (5-ALA), a naturally occurring amino acid, is approved by the U.S. Food and Drug Administration (FDA) for cancer diagnosis and treatment as photodynamic therapy.56,57 It can be metabolized to the bioactive photosensitizer protoporphyrin IX (PpIX) through the heme biosynthesis pathway (Scheme S2†).58 As shown in Fig. 5A, 5-ALA was initially added to HeLa cells for 24 hours to establish an endogenous PpIX cell model. Subsequently, the probe QMI was added to HeLa cells and allowed to incubate for 1 hour. Following the incubation, HeLa cells were washed three times with PBS to remove residues of 5-ALA and QMI. Finally, the CL signal from the cells was promptly collected after light irradiation. The observed characteristic fluorescence of PpIX in HeLa cells within 620–710 nm indicated the successful establishment of the endogenous PpIX cell model (Fig. 5B). As expected, a conspicuous CL signal appeared in the QMI and 5-ALA pretreatment groups after irradiation, which was a 46-fold increase compared to the corresponding dark group (Fig. 5C). In contrast, cells treated with either QMI or 5-ALA alone displayed no recognizable CL signals even under light irradiation (Fig. 5C and D). In addition, QMI only consumed a small amount of singlet oxygen; therefore, it did not affect the efficiency of 5-ALA-mediated PDT treatment (Fig. S28†). All these results provide strong support that the zwitterionic CL probe QMI successfully and effectively tracks the intracellular 1O2 generation during the PDT process.
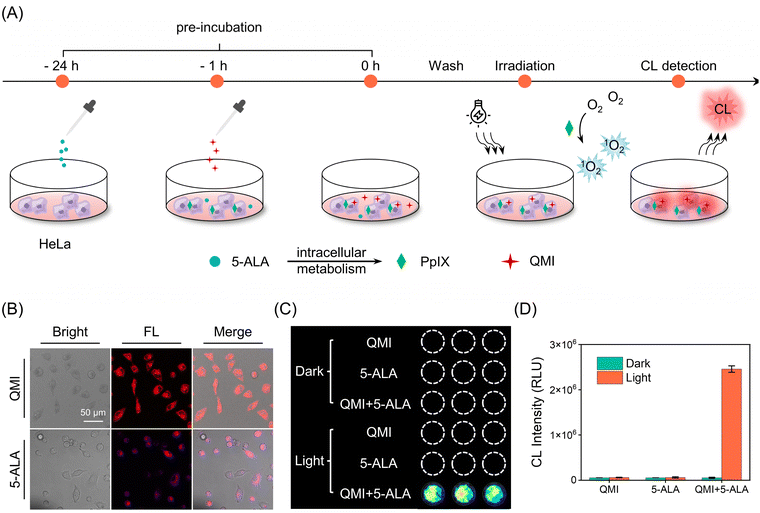 |
| Fig. 5 Lighting up endogenous 1O2 from FDA-approved 5-ALA phototherapy in living cells. (A) Schematic illustration of cell treatment and imaging procedure. (B) Confocal fluorescent images of HeLa cells incubated with QMI (10 μM) for 1 h and 5-ALA (1 mM, PpIX precursor) for 24 h, respectively. QMI group excitation at 520 nm and collection at 600–700 nm; 5-ALA group excitation at 410 nm and collection at 620–710 nm. Scale bar = 50 μm. (C) HeLa cells were incubated with QMI (20 μM), 5-ALA (1 mM) alone, or 5-ALA followed by QMI, and then the CL images were obtained with or without 635 nm laser (200 mW cm−2) irradiation for 3 min. (D) Quantification of CL intensity emitted from the HeLa cells. Data with error bars are expressed as mean ± s.d., n = 3. | |
In situ monitoring of singlet oxygen from FDA-approved 5-ALA in living mice
Motivated by the excellent in vitro performance of the zwitterionic CL probe, we further explore the sensing capabilities of QMI for 1O2 in living mice. As shown in Fig. 6A, the CL signal exhibited neglectable enhancement in the QMI-only group upon light irradiation. Notably, there was an exponential increase of approximately 72.3-fold in the chemiluminescence signal for 1O2 from the co-injected group of the zwitterionic QMI and photosensitizers (rose bengal, RB) under light irradiation (Fig. 6B and S29†). In contrast, the photoactivable probe QMCL, even without the photosensitizer, displayed a significant CL signal (31-fold enhancement) under light irradiation in living mice (Fig. 6C), due to its inherent photoinduced activation characteristic. Consequently, suffering from severely photoinduced interference, the CL enhancement of QMCL towards 1O2 was limited to a modest 7.6-fold with the presence of photosensitizer (Fig. 6D). Similar photo-induced side effects were found in the previously reported CL probe CL-SO (Scheme S2†) towards 1O2,59 with the exception of a slight decrease rather than enhancement in CL signals with photosensitizer (Fig. 6E and F). These results collectively suggest that the zwitterionic strategy for QMI can effectively mitigate photo-induced side reactions and therefore enable accurate in vivo detection of 1O2.
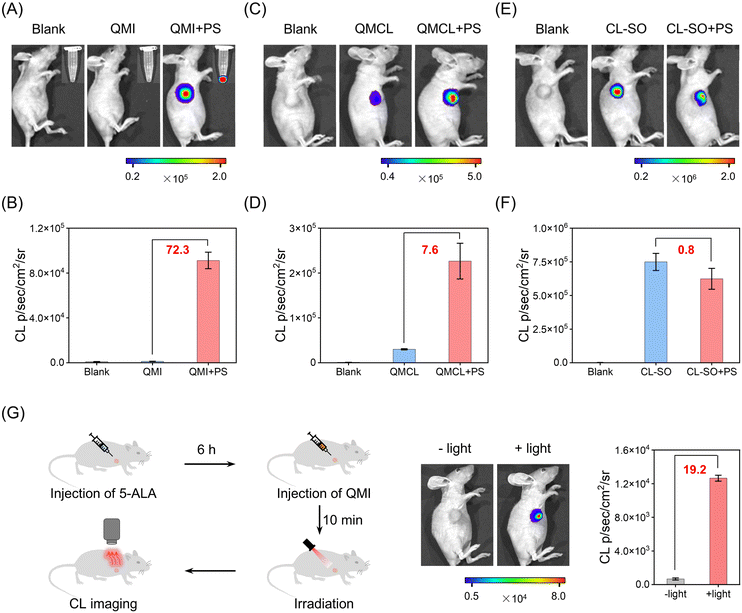 |
| Fig. 6
In vivo CL imaging of intratumor 1O2 during photodynamic therapy. CL images of HeLa xenograft tumor-bearing mice after intratumor injection of the zwitterionic probe QMI or QMI + PS mixed solution (A), QMCL or QMCL + PS mixed solution (C), and CL-SO or CL-SO + PS mixed solution (E). Inset: in vitro CL images of QMI. CL images were acquired upon white light (120 mW cm−2) irradiation for 1 min. Quantification of CL signal intensities evolving from mice: zwitterionic CL probe QMI (B), QMCL (D), and CL-CO (F). (G) Schematic diagram of CL imaging of 1O2 from FDA-approved 5-ALA in living mice. CL images of pretreated xenograft tumor-bearing mice. Quantification of CL signal intensities in corresponding mice. CL images were acquired upon 635 nm laser (200 mW cm−2) irradiation for 3 min. Data with error bars are expressed as mean ± s.d., n = 3. | |
Furthermore, we undertook a comparison with the commercial probes SOSG and ICG, and the in vivo capability of the zwitterionic probe QMI to sense 1O2in vivo was evaluated in living mice. Notably, the fluorescence signal of SOSG in response to 1O2 generated from photosensitizers was merely 1.2-fold higher under light irradiation in living mice (Fig. S30†), primarily due to its photo-induced strong background fluorescence. This slight increase was much lower than the SNR of QMI. Subsequently, we explored and validated the sensitivity of QMI in detecting 1O2 during the ICG-based PDT process. A remarkably enhanced signal was observed in the QMI and ICG groups upon 808 nm laser irradiation (Fig. S31†), which could not be observed using the available probes. Indeed, the zwitterionic probe QMI possesses the capability to effectively sense even slight amounts of 1O2 in living mice. Similarly, a bright CL signal was also obtained in the QMI and Cy5 groups under 635 nm laser irradiation (Fig. S32†). All these in vivo imaging results indicated that the zwitterionic CL probe QMI has an ultra-high sensitivity towards in vivo1O2.
We further investigated the practical application of QMI in PDT treatment. As shown in Fig. 6G, the FDA-approved phototherapeutic drug 5-ALA was pre-injected into the tumor for 6 h, followed by the intratumoral injection of the zwitterionic probe QMI for 10 minutes prior to light irradiation. In this context, 5-ALA serves to induce the tumor to produce endogenous PpIX for subsequent PDT, while QMI functions as an indicator, offering real-time feedback on therapeutic efficacy. Upon light irradiation, a distinct CL signal was evident in the tumor tissue, showing an approximately 19-fold enhancement. The clinical PDT results demonstrated that the zwitterionic probe QMI could efficiently identify in vivo1O2, thus enabling immediate feedback on PDT treatment effectiveness and guiding personalized therapy.
Conclusions
We present a de novo zwitterionic design strategy to construct ultra-stable NIR chemiluminescent probes, making a breakthrough in an effective solution for resolving inherent photo-induced side reactions with poor selectivity towards 1O2. TD-DFT calculation and transient absorption spectroscopy confirmed that the zwitterionic CL probes undergo conversion into an inert ketone excited state with an extremely short lifetime (14 ps) through conical intersection (CI), impeding the undesirable photoreaction. Fundamentally, the ultra-stable zwitterionic strategy ensures transforming the [2 + 2] cycloaddition between the enolether unit and 1O2 into a distinctive recognition process against other strong oxidative ROS. Compared with the well-known commercial probe SOSG, the zwitterionic probe QMI displays ultra-high selectivity and sensitivity (SNR over 40-fold) towards 1O2. This zwitterionic chemiluminescence strategy breaks the bottlenecks of photolysis and fragility from the electron-rich enolether moiety. Of particular significance is that the zwitterionic NIR probe QMI, with excellent selectivity, high sensitivity, and outstanding photostability towards 1O2, successfully monitored the FDA-approved 5-ALA-mediated in vivo PDT process in living mice in real time. We anticipate that this straightforward and versatile zwitterionic design strategy opens a new avenue for high-performance chemiluminescent probes, thereby contributing to the expansion of the in vivo toolbox.
Data availability
Experimental procedures and all relevant data are available in ESI† and from the authors.
Author contributions
All the experiments were conducted by Y. L., Y. Z., and C. Y. with the supervision of Z. G. and W. Z. All the computational work was conducted by X. W. with the supervision of X. L. All the femtosecond transient absorption spectra experiments were conducted by R. P. with the supervision of W. L. All authors discussed the results and co-wrote the manuscript.
Conflicts of interest
The authors declare no competing financial interests.
Acknowledgements
This work was supported by NSFC/China (32121005, 22225805, 22308101, and 32394001), the National Key Research and Development Program (2021YFA0910000), the Shanghai Science and Technology Innovation Action Plan (No. 23J21901600), the Innovation Program of Shanghai Municipal Education Commission, the Shanghai Frontier Science Research Base of Optogenetic Techniques for Cell Metabolism (Shanghai Municipal Education Commission, grant 2021 Sci & Tech 03-28), the China Postdoctoral Science Foundation (2021M701199), the Shanghai Municipal Science and Technology Major Project (Grant 2018SHZDZX03), and the Natural Science Foundation of Shanghai (23ZR1416600).
Notes and references
- J.-T. Hou, K.-K. Yu, K. Sunwoo, W. Y. Kim, S. Koo, J. Wang, W. X. Ren, S. Wang, X.-Q. Yu and J. S. Kim, Chem, 2020, 6, 832–866 CAS.
- J. H. Kim, P. Verwilst, M. Won, J. Lee, J. L. Sessler, J. Han and J. S. Kim, J. Am. Chem. Soc., 2021, 143, 14115–14124 CrossRef CAS PubMed.
- H. Wu, L. Wang, Y. Wang, Y. Shao, G. Li, K. Shao and E. U. Akkaya, Angew. Chem., Int. Ed., 2022, 61, e202210249 CrossRef CAS PubMed.
- X. Wu, M. Yang, J. S. Kim, R. Wang, G. Kim, J. Ha, H. Kim, Y. Cho, K. T. Nam and J. Yoon, Angew. Chem., Int. Ed., 2022, 61, e202200808 CrossRef CAS PubMed.
- W. Chen, Z. Wang, M. Tian, G. Hong, Y. Wu, M. Sui, M. Chen, J. An, F. Song and X. Peng, J. Am. Chem. Soc., 2023, 145, 8130–8140 CrossRef CAS PubMed.
- M.-Y. Wu, L. Chen, Q. Chen, R. Hu, X. Xu, Y. Wang, J. Li, S. Feng, C. Dong, X.-L. Zhang, Z. Li, L. Wang, S. Chen and M. Gu, Adv. Mater., 2022, 35, 2208578 CrossRef PubMed.
- W. Zhu, Y. Li, S. Guo, W.-J. Guo, T. Peng, H. Li, B. Liu, H.-Q. Peng and B. Z. Tang, Nat. Commun., 2022, 13, 7046 CrossRef CAS PubMed.
- X. Li, S. Yu, Y. Lee, T. Guo, N. Kwon, D. Lee, S. C. Yeom, Y. Cho, G. Kim, J.-D. Huang, S. Choi, K. T. Nam and J. Yoon, J. Am. Chem. Soc., 2019, 141, 1366–1372 CrossRef CAS PubMed.
- W. Wu, D. Mao, F. Hu, S. Xu, C. Chen, C.-J. Zhang, X. Cheng, Y. Yuan, D. Ding, D. Kong and B. Liu, Adv. Mater., 2017, 29, 1700548 CrossRef PubMed.
- X. Zhao, Q. Yao, S. Long, W. Chi, Y. Yang, D. Tan, X. Liu, H. Huang, W. Sun, J. Du, J. Fan and X. Peng, J. Am. Chem. Soc., 2021, 143, 12345–12354 CrossRef CAS PubMed.
- N. Hananya, O. Green, R. Blau, R. Satchi-Fainaro and D. Shabat, Angew. Chem., Int. Ed., 2017, 56, 11793–11796 CrossRef CAS PubMed.
- L. Lei, F. Yang, X. Meng, L. Xu, P. Liang, Y. Ma, Z. Dong, Y. Wang, X.-B. Zhang and G. Song, J. Am. Chem. Soc., 2023, 145, 24386–24400 CrossRef CAS PubMed.
- B. Li, L. Lin, H. Lin and B. C. Wilson, J. Biophotonics, 2016, 9, 1314–1325 CrossRef CAS PubMed.
- C. Zhao, J. Chen, R. Zhong, D. S. Chen, J. Shi and J. Song, Angew. Chem., Int. Ed., 2021, 60, 9804–9827 CrossRef CAS PubMed.
- J. Lyu, M. Cheng, J. Liu and J. Lv, ACS Appl. Mater. Interfaces, 2022, 14, 54081–54089 CrossRef CAS PubMed.
- S. Chen, Y. Zhong, W. Fan, J. Xiang, G. Wang, Q. Zhou, J. Wang, Y. Geng, R. Sun, Z. Zhang, Y. Piao, J. Wang, J. Zhuo, H. Cong, H. Jiang, J. Ling, Z. Li, D. Yang, X. Yao, X. Xu, Z. Zhou, J. Tang and Y. Shen, Nat. Biomed. Eng., 2021, 5, 1019–1037 CrossRef CAS PubMed.
- N. Ruan, Q. Qiu, X. Wei, J. Liu, L. Wu, N. Jia, C. Huang and T. D. James, J. Am. Chem. Soc., 2024, 146, 2072–2079 CrossRef CAS PubMed.
- W. Zeng, L. Wu, Y. Ishigaki, T. Harimoto, Y. Hu, Y. Sun, Y. Wang, T. Suzuki, H. Y. Chen and D. Ye, Angew. Chem., Int. Ed., 2022, 134, e202111759 CrossRef.
- E. M. Surender, S. J. Bradberry, S. A. Bright, C. P. McCoy, D. C. Williams and T. Gunnlaugsson, J. Am. Chem. Soc., 2016, 139, 381–388 CrossRef PubMed.
- H. Shen, F. Sun, X. Zhu, J. Zhang, X. Ou, J. Zhang, C. Xu, H. H. Y. Sung, I. D. Williams, S. Chen, R. T. K. Kwok, J. W. Y. Lam, J. Sun, F. Zhang and B. Z. Tang, J. Am. Chem. Soc., 2022, 144, 15391–15402 CrossRef CAS PubMed.
- L. Wang, M. Tran, E. D'Este, J. Roberti, B. Koch, L. Xue and K. Johnsson, Nat. Chem., 2020, 12, 165–172 CrossRef CAS PubMed.
- H. Liu, P. J. H. Carter, A. C. Laan, R. Eelkema and A. G. Denkova, Sci. Rep., 2019, 9, 8393 CrossRef PubMed.
- R. Ruiz-González, R. Bresolí-Obach, Ò. Gulías, M. Agut, H. Savoie, R. W. Boyle, S. Nonell and F. Giuntini, Angew. Chem., Int. Ed., 2017, 56, 2885–2888 CrossRef PubMed.
- S. Kim, T. Tachikawa, M. Fujitsuka and T. Majima, J. Am. Chem. Soc., 2014, 136, 11707–11715 CrossRef CAS PubMed.
- A. Gollmer, J. Arnbjerg, F. H. Blaikie, B. W. Pedersen, T. Breitenbach, K. Daasbjerg, M. Glasius and P. R. Ogilby, Photochem. Photobiol., 2011, 87, 671–679 CrossRef CAS PubMed.
- X. Su, X. Kong, K. Sun, Q. Liu, Y. Pei, D. Hu, M. Xu, W. Feng and F. Li, Angew. Chem., Int. Ed., 2022, 61, e202201630 CrossRef CAS PubMed.
- C. Chen, H. Gao, H. Ou, R. T. K. Kwok, Y. Tang, D. Zheng and D. Ding, J. Am. Chem. Soc., 2022, 144, 3429–3441 CrossRef CAS PubMed.
- Y. Liu, L. Teng, Y. Lyu, G. Song, X.-B. Zhang and W. Tan, Nat. Commun., 2022, 13, 2216 CrossRef CAS.
- J. Cao, W. An, A. G. Reeves and A. R. Lippert, Chem. Sci., 2018, 9, 2552–2558 RSC.
- T. E. Anderson, A. A. Andia and K. A. Woerpel, Tetrahedron, 2021, 82, 131874 CrossRef CAS PubMed.
- J. A. Bull, R. A. Croft, O. A. Davis, R. Doran and K. F. Morgan, Chem. Rev., 2016, 116, 12150–12233 CrossRef CAS PubMed.
- B. Yang and Z. Lu, J. Org. Chem., 2016, 81, 7288–7300 CrossRef CAS PubMed.
- J. Huang, L. Su, C. Xu, X. Ge, R. Zhang, J. Song and K. Pu, Nat. Mater., 2023, 22, 1421–1429 CrossRef PubMed.
- Y. Zhang, C. Yan, C. Wang, Z. Guo, X. Liu and W.-H. Zhu, Angew. Chem., Int. Ed., 2020, 59, 9059–9066 CrossRef CAS PubMed.
- C. Li, Y. Xu, L. Tu, M. Choi, Y. Fan, X. Chen, J. L. Sessler, J. S. Kim and Y. Sun, Chem. Sci., 2022, 13, 6541–6549 RSC.
- Q. Xu, Y. Zhang, M. Zhu, C. Yan, W. Mao, W.-H. Zhu and Z. Guo, Chem. Sci., 2023, 14, 4091–4101 RSC.
- L. Wu, J. Liu, P. Li, B. Tang and T. D. James, Chem. Soc. Rev., 2021, 50, 702–734 RSC.
- W. Zhang, Y. Lv, F. Huo, Y. Yun and C. Yin, Adv. Mater., 2024, 2314021 CrossRef CAS PubMed.
- X. Wei, J. Huang, C. Zhang, C. Xu, K. Pu and Y. Zhang, Angew. Chem., Int. Ed., 2023, 62, e202213791 CrossRef CAS PubMed.
- L. A. MacManus-Spencer and K. McNeill, J. Am. Chem. Soc., 2005, 127, 8954–8955 CrossRef CAS PubMed.
- S. Gutkin, R. Tannous, Q. Jaber, M. Fridman and D. Shabat, Chem. Sci., 2023, 14, 6953–6962 RSC.
- Y. Danten, C. Gatti and C. Frayret, J. Phys. Chem. A, 2022, 126, 9577–9593 CrossRef CAS PubMed.
- K. Kajiwara, H. Osaki, S. Greßies, K. Kuwata, J. H. Kim, T. Gensch, Y. Sato, F. Glorius, S. Yamaguchi and M. Taki, Nat. Commun., 2022, 13, 2533 CrossRef CAS PubMed.
- Z. Guo, C. Yan and W.-H. Zhu, Angew. Chem., Int. Ed., 2020, 59, 9812–9825 CrossRef CAS PubMed.
- M. Hao, W. Chi, C. Wang, Z. Xu, Z. Li and X. Liu, J. Phys. Chem. C, 2020, 124, 16820–16826 CrossRef CAS.
- A. Kahan, A. Wand, S. Ruhman, S. Zilberg and Y. Haas, J. Phys. Chem. A, 2011, 115, 10854–10861 CrossRef CAS PubMed.
- L. Shi, C. Yan, Z. Guo, W. Chi, J. Wei, W. Liu, X. Liu, H. Tian and W.-H. Zhu, Nat. Commun., 2020, 11, 793 CrossRef CAS PubMed.
- Y.-L. Lee, Y.-T. Chou, B.-K. Su, C.-c. Wu, C.-H. Wang, K.-H. Chang, J.-a. A. Ho and P.-T. Chou, J. Am. Chem. Soc., 2022, 144, 17249–17260 CrossRef CAS PubMed.
- Y. You, Org. Biomol. Chem., 2018, 16, 4044–4060 RSC.
- M. A. Filatov, S. Karuthedath, P. M. Polestshuk, H. Savoie, K. J. Flanagan, C. Sy, E. Sitte, M. Telitchko, F. Laquai, R. W. Boyle and M. O. Senge, J. Am. Chem. Soc., 2017, 139, 6282–6285 CrossRef CAS PubMed.
- T. Luo, Q. Zhang and Q.-B. Lu, Cancers, 2017, 9, 63 CrossRef PubMed.
- C. Shirata, J. Kaneko, Y. Inagaki, T. Kokudo, M. Sato, S. Kiritani, N. Akamatsu, J. Arita, Y. Sakamoto, K. Hasegawa and N. Kokudo, Sci. Rep., 2017, 7, 13958 CrossRef PubMed.
- J. Atchison, S. Kamila, H. Nesbitt, K. A. Logan, D. M. Nicholas, C. Fowley, J. Davis, B. Callan, A. P. McHale and J. F. Callan, Chem. Commun., 2017, 53, 2009–2012 RSC.
- R. H. Kang, Y. Kim, H. J. Um, J. Kim, E.-K. Bang, S. G. Yeo and D. Kim, ACS Appl. Nano Mater., 2022, 5, 5387–5397 CrossRef CAS.
- S. Joseph and S. K. Ashok Kumar, Coord. Chem. Rev., 2023, 496, 215408 CrossRef CAS.
- K. Mahmoudi, K. L. Garvey, A. Bouras, G. Cramer, H. Stepp, J. G. Jesu Raj, D. Bozec, T. M. Busch and C. G. Hadjipanayis, J. Neuro-Oncol., 2019, 141, 595–607 CrossRef CAS PubMed.
- L. Marocco, F. Umrath, S. Sachsenmaier, R. Rabiner, N. Wülker and M. Danalache, Biomedicines, 2022, 10, 2900 CrossRef CAS PubMed.
- Y. Shinoda, D. Kato, R. Ando, H. Endo, T. Takahashi, Y. Tsuneoka and Y. Fujiwara, Pharmaceuticals, 2021, 14, 229 CrossRef CAS PubMed.
- M. Yang, J. Zhang, D. Shabat, J. Fan and X. Peng, ACS Sens., 2020, 5, 3158–3164 CrossRef CAS PubMed.
|
This journal is © The Royal Society of Chemistry 2024 |
Click here to see how this site uses Cookies. View our privacy policy here.