DOI:
10.1039/D4SC02204A
(Edge Article)
Chem. Sci., 2024,
15, 11367-11373
Blue light emission enhancement and robust pressure resistance of gallium oxide nanocrystals†
Received
3rd April 2024
, Accepted 9th June 2024
First published on 21st June 2024
Abstract
Exploration of pressure-resistant materials largely facilitates their operation under extreme conditions where a stable structure and properties are highly desirable. However, under extreme conditions, such as a high pressure over 30.0 GPa, fluorescence quenching generally occurs in most materials. Herein, pressure-induced emission enhancement (PIEE) by a factor of 4.2 is found in Ga2O3 nanocrystals (NCs), a fourth-generation ultrawide bandgap semiconductor. This is mainly attributed to pressure optimizing the intrinsic lattice defects of the Ga2O3 nanocrystals, which was further confirmed by first-principles calculations. Note that the bright blue emission could be stabilized even up to a high pressure of 30.6 GPa, which is of great significance in the essential components of white light. Notably, after releasing the pressure to ambient conditions, the emission of the Ga2O3 nanocrystals can completely recover, even after undergoing multiple repeated pressurizations. In addition to stable optical properties, synchrotron radiation shows that the Ga2O3 nanocrystals remain in the cubic structure described by space group Fd3m upon compression, demonstrating the structural stability of the Ga2O3 nanocrystals under high pressure. This study pays the way for the application of oxide nanomaterials in pressure anti-counterfeiting and pressure information memory devices.
Introduction
Luminescent materials in response to environmental stimuli have drawn increasing research interest for their potential applications in information storage, optoelectronic devices and sensors.1–5 Over the past few decades, various kinds of luminescent materials, including halide perovskites, organic molecules and quantum dots, have shown interesting pressure-induced photoluminescence (PL) changes.6–9 For example, pressure-induced emission (PIE) and piezochromism were found in the compelling materials of Cs4PbBr6 nanocrystals (NCs) and Rb2TeCl6 microcrystals (MCs), respectively.10–12 However, above-mentioned materials usually suffered from PL quenching under extremely high pressures of over 30 GPa,5–7,10 resulting from the non-radiative contribution. In addition, few materials could retain their initial optical properties after full pressure release, which greatly limits their practical applications. Therefore, there is an urgent need to explore materials that can keep their intense PL intensity under high pressure.
Compared to the above-mentioned materials, semiconductor oxides have shown relative stability in response to external pressure.13–15 At the turn of the century, Jiang et al. performed a study of the effects of pressure on ZnO NCs, revealing that the ZnO NCs maintained structural stability over 15.0 GPa, while it was only 9.9 GPa for bulk ZnO. This finding sparked interest in the pressure-related stabilization of semiconductor oxide NCs.13 Wang et al. further reported that β-Ga2O3 NCs exhibited no structural transition after reaching 16.4 GPa.14 The optical properties of the oxide NCs also have attracted research interest because of their unique defect emission mechanism. Oxygen vacancies in nanocrystalline oxides are known to be common and prevalent defects, acting as emission centers and radiative traps in luminescence processes.16–21 They can also form defect levels in the band gap and contribute to mid-gap luminescence.16 However, few studies have focused on the pressure-dependent change in their optical properties. Considering the structural stability of the semiconductor oxides under pressure, it is imperative to study their optical properties that can glow sustainably under pressure.
Herein, we conducted a systematic investigation on the optical properties and crystal structure of γ-Ga2O3 NCs under high pressure. We observed a 4.2-fold increase in the PL intensity of Ga2O3 NCs under pressure. This phenomenon was attributed to pressure-induced optimized lattice defects. First-principles calculations showed the defect reduction and lattice optimization of the Ga2O3 NCs under pressure are related to a decrease in oxygen vacancy formation energy, which corresponds to a resulting increase in the PL intensity. Notably, even at an elevated pressure of 30.6 GPa, the PL intensity of the Ga2O3 NCs remained stronger than that observed under normal conditions. Furthermore, our results from high-pressure absorption spectra and ADXRD revealed that the band gap and crystal structure of the Ga2O3 NCs exhibited remarkable stability and exceptional resistance to high pressure, respectively. Because of their outstanding stability and super “pressure resistance,” the Ga2O3 NCs can potentially enable multiple copying functions under pressure, thereby enhancing device longevity and positioning themselves as promising candidates for next-generation stable ultra-pressure-resistant luminescent materials.
Results and discussion
The Ga2O3 NCs were synthesized by a modified colloidal hot injection experiment.18–22Fig. 1a shows the morphology and microstructure of the Ga2O3 NCs, characterized by transmission electron microscopy (TEM) and high-resolution transmission electron microscopy (HRTEM). It is observed that synthesized Ga2O3 NCs are homogeneous nanoparticles with a well monodispersed spherical shape. The inset in Fig. 1a shows a Gaussian fit of the Ga2O3 NCs with an average diameter of approximately 3.2 nm. The Ga2O3 NCs exhibit a broad blue PL emission at an excitation wavelength of 355 nm (Fig. 1b). The biexponential equation fitted the results well (Fig. S1 ESI†), indicating that the average fluorescence lifetime of the Ga2O3 NCs is approximately 2.5 ns. The photoluminescence quantum yield (PLQY) is measured to be about 16% for the Ga2O3 NCs. We modified XRD patterns with the corresponding refinement and residual by GSAS. In terms of the peak width of (311) planes, the particle size is estimated to be about 3.07 nm, as determined by the Scherrer formula.23 XRD analysis and structural simulations show that the synthesized Ga2O3 NCs belong to the γ-Ga2O3 cubic structure described by space group Fd3m (Fig. 1c and d).18,21,24
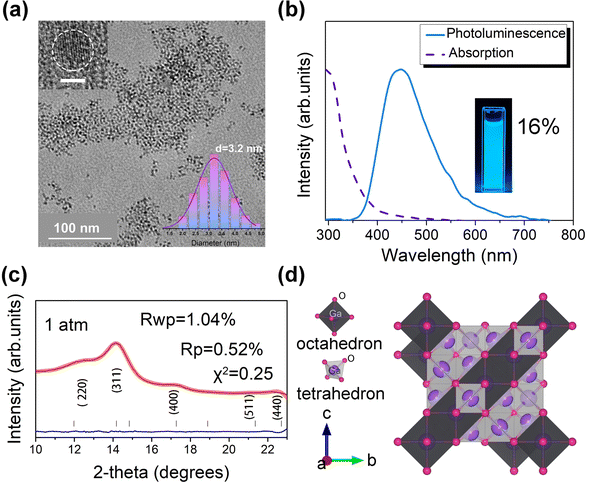 |
| Fig. 1 (a) TEM image of the Ga2O3 NCs. The top inset shows the high-resolution TEM image whose scale bar is 5 nm. The bottom inset shows the corresponding size distribution of as-prepared Ga2O3 NCs. (b) Steady-state absorption spectra and PL spectra excited by 355 nm monochromatic light. The inset shows a fluorescence photograph under 365 nm excitation. (c) Rietveld refinements of the Ga2O3 NCs at 1 atm. (d) Schematic of the cubic crystal spinel structure of the Ga2O3 NCs. | |
We further investigated the optical properties of the Ga2O3 NCs under high pressure. Experiments were performed using silicone oil as a pressure medium, which remains quasi-hydrostatic up to 4 GPa.25 For more details on the experiments, see ESI.† As shown in Fig. 2a, the PL intensity of the Ga2O3 NCs increased significantly and reached its highest intensity at 6.0 GPa, which was 4.2 times the initial intensity. Comparative experiments with commercial bulk Ga2O3 demonstrated that bulk Ga2O3 did not show the PIEE phenomenon. The luminescence stability of the Ga2O3 NCs is better than that of the bulk counterpart, as shown Fig. S2.† Note that, although the PL intensity of the Ga2O3 NCs started to weaken slowly when the pressure exceeded 6.0 GPa, the emission of the Ga2O3 NCs still stronger than that under normal pressure up to 30.6 GPa (Fig. 2b). Meanwhile, the PL peak position of the Ga2O3 NCs remained almost unchanged under high pressure, which highlights the stability and super “pressure resistance” of the Ga2O3 NCs. Pressure-dependent colorimetry coordinates (CIE) indicate that the fluorescent color of the Ga2O3 NCs is less affected by pressure, indicating that the optical properties of the Ga2O3 NCs show excellent stability under pressure (Fig. 2c). In Fig. 2d, the absorption and emission spectra of the Ga2O3 NCs are almost identical under 1 atm, 30.6 GPa and release. The optical microscope images of the Ga2O3 NCs versus pressure in a diamond anvil cell chamber clearly illustrate the variation in PL brightness (Fig. 2e). We also designed repeated high-pressure experiments for the synthesized Ga2O3 NCs to further demonstrate the “pressure resistance” of the Ga2O3 NCs under high pressure (Fig. S3†). As shown in Fig. 2f, the changes in emission intensity and peak position of the Ga2O3 NCs remained consistent, with good stability and reproducibility throughout four compression cycles. When the pressure was fully released to ambient conditions, the sample almost reverted to the initial PL intensity and color (Fig. S4†). The reversibility of the Ga2O3 NCs under pressure can effectively improve the material reuse life of a pressure sensor (Fig. S5†).
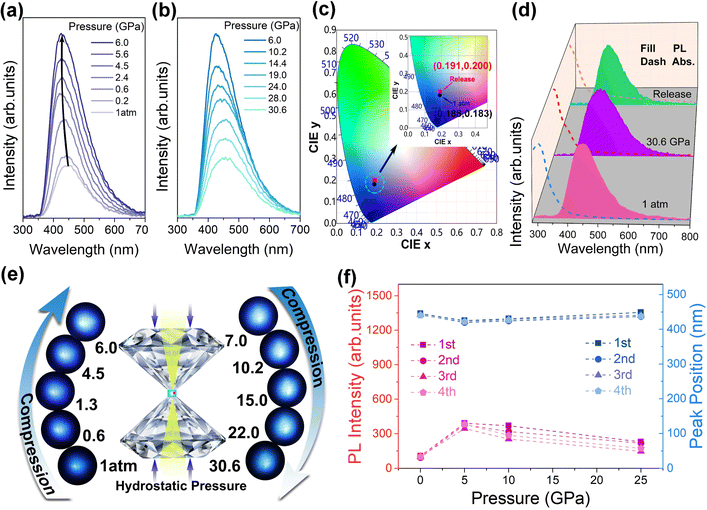 |
| Fig. 2 (a) and (b) PL spectra of the Ga2O3 NCs under high pressure. (c) Chromaticity coordinate diagram where the green dashed line shows a region of ambient pressure and release for the selected chromaticity values of the Ga2O3 NCs. (d) Absorption and emission spectra of the Ga2O3 NCs at 1 atm pressure, 30.6 GPa and decompression. (e) PL micrographs of the Ga2O3 NCs upon compression under 355 nm photoexcitation. (f) Changes in peak position and intensity of repeated compression emission from the Ga2O3 NCs. (The error of all pressures measured in the experiments is ±0.1 GPa.) | |
An in situ high-pressure cycle experiment on ultraviolet-visible absorption spectra was performed to characterize the bandgap evolution in the Ga2O3 NCs (Fig. 3a and S6†). It is observed that the 355 nm absorption edge of the Ga2O3 NCs underwent only a minor redshift during pressurization. The alteration in the band gap was estimated using the Tauc plot fitted with the absorption spectrum. The band structure and density of state of the Ga2O3 NCs were calculated using first-principles density functional theory (DFT) calculations, which confirmed that the Ga2O3 NCs belong to the direct bandgap (Fig. 3b).24Fig. 3b shows that DFT underestimates the band-gap energy, which is a typical feature of DFT calculation.26 According to Fig. 3c and S7,† the Ga2O3 NCs exhibited a decrease in band gap when compressed from ambient conditions to 4.0 GPa, followed by a slow decrease. It is found that there is only a marginal overall variation of approximately 0.2 eV in absorbed and emitted energy during compression. After the pressure was fully released, absorbed energy and Stokes displacement remained nearly unchanged compared to their initial values, indicating structural reversibility (Fig. 3c). The relationship between the stable optical properties and structure of the Ga2O3 NCs was investigated by high-pressure ADXRD experiments.10,27–30 Experimental results from ADXRD showed that the Ga2O3 NCs maintained a stable phase structure as the pressure reached 30.6 GPa (Fig. 3d). We carried out a transmission electron microscope (TEM) test of samples after the restoration of environmental conditions. It was found that the morphology of the samples under atmospheric pressure and after releasing pressure are nanoparticles without obvious changes (Fig. S8†). Therefore, all of the optical properties, structure and morphology of the Ga2O3 NCs can remain stable after pressure treatment. The volume data was fitted with a third-order Birch–Murnaghan equation of state:31–33
where
V0 is the volume at zero pressure,
B0 is the bulk modulus at ambient pressure, and

is a parameter for the pressure derivative. The fitting of the Birch–Murnaghan equation indicated that the isothermal bulk modulus
B0 for the Ga
2O
3 NCs was estimated as 137.34 GPa, much higher than that (85.9 GPa) of the bulk counterpart
34 (Fig. S9
†). Materials showing higher bulk modulus are more difficult to compress, which further illustrates the stability of the Ga
2O
3 NCs.
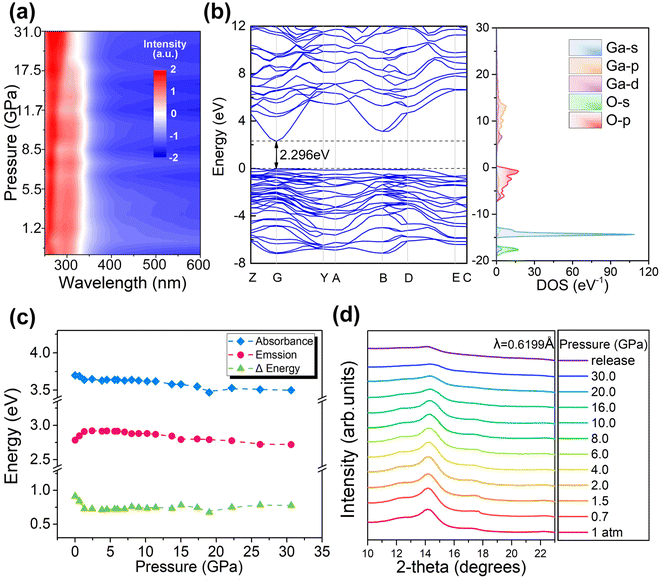 |
| Fig. 3 (a) Pressure-dependent optical absorption spectra of the Ga2O3 NCs. (b) DFT-calculated band structure and projected density of states of Ga2O3 NCs under ambient conditions. (c) Absorption and emission energies of the Ga2O3NCs upon compression. (d) High-pressure ADXRD pattern for the Ga2O3 NCs. | |
The cause of the luminescence in Ga2O3 is generally believed to be acceptor–donor pair (DAP) recombination, and oxygen vacancy defects are crucial in DAP recombination.18,19,27,35–37 For the DAP recombination model, the energy (E) of the emitted photon depends on the distance (r) between donor and acceptor sites and can be expressed by the following equation:
| 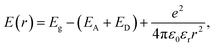 | (1) |
where the first term
Eg is the band gap energy of Ga
2O
3; second and third terms EA and ED are the binding energies of the acceptor and donor; and the fourth term is the coulombic energy between the acceptor and donor, where
r is the distance between the acceptor and donor.
We analyzed the behavior of the oxygen vacancy defects under pressure by simulating the formation energy of the oxygen vacancy defects at different pressures,18,19,38 which can be represented by following equations:
| 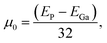 | (3) |
where
EO is the energy of containing the oxygen vacancy defects;
EP is the energy of completeness;
EGa is the energy of containing only Ga atoms;
μ0 is the chemical potential of oxygen in Ga
2O
3 NCs; and Δ
H is the energy of formation of the oxygen vacancy defects.
The oxygen vacancy formation energy (ΔH) of the Ga2O3 NCs was calculated at atmospheric pressure, 1.5 GPa, 3.0 GPa and 4.5 GPa (Fig. S10†). The results show that ΔH increases in the low-pressure region (<1.5 GPa), resulting in the suppression of oxygen vacancy defect formation. This contributes to a certain degree of lattice optimization of the Ga2O3 NCs.27,38–40 In the high-pressure region (>1.5 GPa), because of the excessive degree of lattice distortion in the Ga2O3 NCs, ΔH decreases. The proposed mechanism regarding the pressure increase of the oxygen vacancies is fully coherent with the broadening of XRD peaks, as shown in Fig. 3d, and decrease in signal intensity, which is probably due to pressure-driven disorder in the crystal structure.41 This trend promotes an increase in defects, giving rise to a slow decrease in emission. The suppression of oxygen vacancy defect formation will lead to a decrease in the distance (r) between the acceptor and donor in the DAP complex,27,38 and hence a shift in DAP emission towards higher energy (Fig. 4a).19,20,36,37 This finding further supports the blueshift observed in the Ga2O3 NCs under low-pressure conditions. Furthermore, defects in crystals exhibit two primary types of behavior: (1) non-radiative leaps and (2) DAP luminescence.18,19,27,35,36 The majority of the oxygen vacancy defects in the Ga2O3 NCs participate in non-radiative transitions, while only a small proportion are involved in DAP complexes.27 Combining theoretical calculations, a reasonable conclusion is that the non-radiative leaps in the Ga2O3 NCs are suppressed under pressure, which eventually leads to the enhancement in emission of the Ga2O3 NCs.27
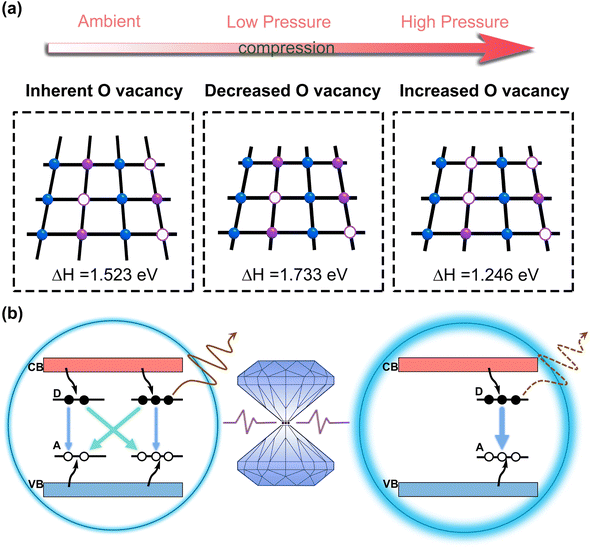 |
| Fig. 4 (a) Schematic representation of the mechanism of oxygen-vacancy-induced defect luminescence and pressure-induced emission enhancement. (Blue dots are Ga atoms, purple dots are O atoms, and purple circles are O vacancies.) (b) Schematic of the DAP model with the binding energy of acceptor and donor. | |
Conclusions
Based on the well-established theory of oxygen-vacancy point-defect-induced emission in the Ga2O3 NCs, we devised a high-pressure engineering approach and successfully achieved remarkable PIEE, as well as significant repeated compression-stable emission of the Ga2O3 NCs. During the compression process, the blue emission intensity of the Ga2O3 NCs is enhanced by approximately 4.2 times, which is attributed to pressure optimizing the intrinsic lattice defects of the Ga2O3 NCs. Note that the bright blue emission could be stabilized even up to a high pressure of 30.6 GPa. In contrast, bulk materials exhibited an opposite trend with reduced emission intensity upon compression. High-pressure ADXRD data further confirmed that the Ga2O3 NCs do not undergo a structural phase transition because of their inherent stability and resistance to pressure effects. Our study elucidated the correlation between the structure and properties of the Ga2O3 NCs, which aligned with the general theory of oxygen-vacancy point-defect-induced emission and offered valuable insights for potential applications in third-generation oxide semiconductors and optical pressure sensor materials.
Data availability
The data that support the findings of this study are available in the ESI† of this article.
Author contributions
G. X. and B. Z. designed the experiments. Z. J. and Y. X., performed the experiments and analyzed data. P. L., Y. L. and D. Q. performed partial experiments and calculations. Z. J., P. L., G. X. and B. Z. wrote the manuscript.
Conflicts of interest
There are no conflicts to declare.
Acknowledgements
This work is supported by the National Key R&D Program of China (2023YFA1406200), Jilin Provincial Science & Technology Development Program (20220101002JC), National Science Foundation of China (12174144), Zhejiang Provincial Natural Science Foundation of China (LR22B010001), Graduate Innovation Fund of Jilin University (2023CX040; 2023CX185) and Fundamental Research Funds for the Central Universities. This work was mainly performed at BL15U1 at the Shanghai Synchrotron Radiation Facility (SSRF).
Notes and references
- S. Kim, S. J. Yoon and S. Y. Park, Highly Fluorescent Chameleon Nanoparticles and Polymer Films, Multicomponent Organic Systems that Combine FRET and Photochromic Switching, J. Am. Chem. Soc., 2012, 134, 12091–12097 CrossRef CAS PubMed.
- D. H. Kim, A. D’ Aléo, X. K. Chen, A. D. S. Sandanayaka, D. Yao, L. Zhao, T. Komino, E. Zaborova, G. Canard, Y. Tsuchiya, E. Choi, J. W. Wu, F. Fages, J. L. Brédas, J. C. Ribierre and C. Adachi, High-efficiency electroluminescence and amplified spontaneous emission from a thermally activated delayed fluorescent near-infrared emitter, Nat. Photonics, 2018, 12, 98–104 CrossRef CAS.
- Y. Wang, X. Tan, Y. M. Zhang, S. Zhu, I. Zhang, B. Yu, K. Wang, B. Yang, M. Li, B. Zou and S. X. A. Zhang, Dynamic behavior of molecular switches in crystal under pressure and its reflection on tactile sensing, J. Am. Chem. Soc., 2015, 137, 931–939 CrossRef CAS PubMed.
- Y. Zhao, N. Li, C. Xu, Y. Li, H. Zhu, P. Zhu, X. Wang and W. Yang, Abnormal pressure-Induced photoluminescence enhancement and phase decomposition in pyrochlore La2Sn2O7, Adv. Mater., 2017, 29, 1701513 CrossRef PubMed.
- Q. Lou, X. Yang, K. Liu, Z. Ding, J. Qin, Y. Li, C. Lv, Y. Shang, Y. Zhang, Z. Zhang, J. Zang, L. Dong and C. X. Shan, Pressure-induced photoluminescence enhancement and ambient retention in confined carbon dots, Nano Res., 2022, 15, 2545–2551 CrossRef CAS.
- Z. Chi, X. Zhang, B. Xu, X. Zhou, C. Ma, Y. Zhang, S. Liu and J. Xu, Recent advances in organic mechanofluorochromic materials, Chem. Soc. Rev., 2012, 41, 3878–3896 RSC.
- N. Chauvin, A. Mavel, G. Patriarche, B. Masenelli, M. Gendry and D. Machon, Pressure-dependent photoluminescence study of wurtzite InP nanowires, Nano Lett., 2016, 16, 2926–2930 CrossRef CAS PubMed.
- G. Xiao, X. Yang, X. Zhang, K. Wang, X. Huang, Z. Ding, Y. Ma, G. Zou and B. Zou, A protocol to fabricate nanostructured new phase: B31-type MnS synthesized under high pressure, J. Am. Chem. Soc., 2015, 137, 10297–10303 CrossRef CAS PubMed.
- G. Xiao, Y. Wang, D. Han, K. Li, X. Feng, P. Lv, K. Wang, L. Liu, S. A. T. Redfern and B. Zou, Pressure-induced large emission enhancements of cadmium selenide nanocrystals, J. Am. Chem. Soc., 2018, 140, 13970–13975 CrossRef CAS PubMed.
- Z. Ma, Z. Liu, S. Lu, L. Wang, X. Feng, D. Yang, K. Wang, G. Xiao, L. Zhang, S. A. T. Redfern and B. Zou, Pressure-induced emission of cesium lead halide perovskite nanocrystals, Nat. Commun., 2018, 9, 4506 CrossRef PubMed.
- W. Zhao, Z. Ma, Y. Shi, R. Fu, K. Wang, Y. Sui, G. Xiao and B. Zou, Pressure tailoring electron-phonon coupling toward enhanced yellow photoluminescence quantum yield and piezochromism, Cell Rep. Phys. Sci., 2023, 4, 101663 CrossRef CAS.
- Z. W. Ma, G. J. Xiao and B. Zou, Step forward to light up the future: pressure-induced emission, Sci. Bull., 2023, 68, 1588–1590 CrossRef PubMed.
- J. Z. Jiang, J. S. Olsen, L. Gerward, D. Frost, D. Rubie and J. Peyronneau, Structural stability in nanocrystalline ZnO, Europhys. Lett., 2000, 50, 48 CrossRef CAS.
- H. Wang, Y. He, W. Chen, Y. W. Zeng, K. Stahl, T. Kikegawa and J. Z. Jiang, High-pressure behavior of β-Ga2O3 nanocrystals, J. Appl. Phys., 2010, 107, 033520 CrossRef.
- L. Wang, Y. Pan, Y. Ding, W. Yang, W. L. Mao, S. V. Sinogeikin, Y. Meng, G. Shen and H.-k. Mao, High-pressure induced phase transitions of Y2O3 and Y2O3:Eu3+, Appl. Phys. Lett., 2009, 94, 061921 CrossRef.
- T. Lim, S. Lee, M. Meyyappan and S. Ju, Control of semiconducting and metallic indium oxide nanowires, ACS Nano, 2011, 5, 3917–3922 CrossRef CAS PubMed.
- T. Harwig and F. Kellendonk, Some observations on the photoluminescence of doped β-galliumsesquioxide, J. Solid State Chem., 1978, 24, 255–263 CrossRef CAS.
- T. Wang and P. V. Radovanovic, Size-dependent electron transfer and trapping in strongly luminescent colloidal gallium oxide nanocrystals, J. Phys. Chem. C, 2011, 115, 18473–18478 CrossRef CAS.
- T. Wang, S. S. Farvid, M. Abulikemu and P. V. Radovanovic, Size-tunable phosphorescence in colloidal metastable γ-Ga2O3 nanocrystals, J. Am. Chem. Soc., 2010, 132, 9250–9252 CrossRef CAS PubMed.
- B. Fernandes, M. Hegde, P. C. Stanish, Z. L. Mišković and P. V. Radovanovic, Photoluminescence decay dynamics in γ-Ga2O3 nanocrystals: The role of exclusion distance at short time scales, Chem. Phys. Lett., 2017, 684, 135–140 CrossRef CAS.
- T. Wang and P. V. Radovanovic, In situ enhancement of the blue photoluminescence of colloidal Ga2O3 nanocrystals by promotion of defect formation in reducing conditions, Chem. Commun., 2011, 47, 7161–7163 RSC.
- H. Jiang, W. Zhao, R. Fu and G. Xiao, Pressure-resistance of magic-sized cadmium selenide nanocrystals, J. Inorg. Mater., 2021, 36, 502–506 CrossRef.
- A. L. Patterson, The Scherrer formula for X-ray particle size determination, Phys. Rev., 1939, 56, 978–982 CrossRef CAS.
- Y. Huang, A. Gao, D. Guo, X. Lu, X. Zhang, Y. Huang, J. Yu, S. Li, P. Li and W. Tang, Fe doping-stabilized γ-Ga2O3 thin films with a high room temperature saturation magnetic moment, J. Mater. Chem. C, 2020, 8, 536–542 RSC.
- D. Errandonea, Y. Meng, M. Somayazulu and D. Häusermann, Pressure-induced α→ω transition in titanium metal: a systematic study of the effects of uniaxial stress, Phys. B, 2005, 355, 116–125 CrossRef CAS.
- T. Ouahrani, R. M. Boufatah, M. Benaissa, Á. Morales-García, M. Badawi and D. Errandonea, Effect of intrinsic point defects on the catalytic and electronic properties of Cu2WS4 single layer: Ab initio calculations, Phys. Rev. Mater., 2023, 7, 025403 CrossRef CAS.
- P. Lv, D. Zhao, F. Wang, Z. Ma, L. Sui, K. Yuan, K. Wang, J. Ning, G. Xiao and B. Zou, Clarifying the controversy over defect emission of I-III-VI2 nanocrystals via pressure engineering, Adv. Opt. Mater., 2023, 12, 2301758 CrossRef.
- D. Zhao, M. Cong, Z. Liu, Z. Ma, K. Wang, G. Xiao and B. Zou, Steric hindrance effects on the retention of pressure-induced emission toward scintillators, Cell Rep. Phys. Sci., 2023, 4, 101445 CrossRef CAS.
- Z. Ma, F. Li, D. Zhao, G. Xiao and B. Zou, Whether or not emission
of Cs4PbBr6 nanocrystals: High-pressure experimental evidence, CCS Chem., 2020, 2, 71–80 CrossRef CAS.
- F. Bai, K. Bian, X. Huang, Z. Wang and H. Fan, Pressure induced nanoparticle phase behavior, property, and applications, Chem. Rev., 2019, 119, 7673–7717 CrossRef CAS PubMed.
- F. Birch, Finite elastic strain of cubic crystals, Phys. Rev., 1947, 71, 809–824 CrossRef CAS.
- Y. Shi, Y. Fu, Z. Ma, D. Zhao, K. Wang, G. Xiao and B. Zou, Pressure regulating self-trapped states toward remarkable emission enhancement of zero-dimensional lead-free halides nanocrystals, Small, 2023, 19, 2300455 CrossRef CAS PubMed.
- T. Geng, Y. Shi, Z. Liu, D. Zhao, Z. Ma, K. Wang, Q. Dong, G. Xiao and B. Zou, Pressure-induced emission from all-Inorganic two-Dimensional vacancy-ordered lead-free metal halide perovskite nanocrystals, J. Phys. Chem. Lett., 2022, 13, 11837–11843 CrossRef CAS PubMed.
- A. Devamanoharan, V. Venkatachalapathy, V. Veerapandy and P. Vajeeston, Investigating stable low-energy Gallium Oxide (Ga2O3) polytypes: Insights into electronic and optical properties from first principles, ACS Omega, 2024, 9, 16207–16220 CrossRef CAS PubMed.
- S. Yoshioka, H. Hayashi, A. Kuwabara, F. Oba, K. Matsunaga and I. Tanaka, Structures and energetics of Ga2O3 polymorphs, J. Phys.: Condens. Matter, 2007, 19, 346211 CrossRef.
- M. Hegde, T. Wang, Z. L. Miskovic and P. V. Radovanovic, Origin of size-dependent photoluminescence decay dynamics in colloidal γ-Ga2O3 nanocrystals, Appl. Phys. Lett., 2012, 100, 141903 CrossRef.
- P. Lv, D. Zhao, Z. Ma, M. Cong, Y. Sui, G. Xiao and B. Zou, Pressure-Modulated Interface Engineering toward Realizing Core@Shell Configuration Transition, Nano Lett., 2023, 23, 11982–11988 CrossRef CAS PubMed.
- I. Chatratin, F. P. Sabino, P. Reunchan, S. Limpijumnong, J. B. Varley, C. G. Van de Walle and A. Janotti, Role of point defects in the electrical and optical properties of In2O3, Phys. Rev. Mater., 2019, 3, 074604 CrossRef CAS.
- H. Wu, Z. Wang and H. Fan, Stress-induced nanoparticle crystallization, J. Am. Chem. Soc., 2014, 136, 7634–7636 CrossRef CAS PubMed.
- P. Lv, Z. Ma, J. Ning, G. Xiao and B. Zou, Identification of defect origin and white-light emission tuning of chalcogenide quantum dots through pressure engineering, CCS Chem., 2024 DOI:10.31635/ccschem.024.202403971.
- A. Liang, R. Turnbull, C. Popescu, I. Fernandez-Guillen, R. Abargues, P. P. Boix and D. Errandonea, Pressure-induced phase transition versus amorphization in hybrid methylammonium lead bromide perovskite, J. Phys. Chem. C, 2023, 127, 12821–12826 CrossRef CAS PubMed.
|
This journal is © The Royal Society of Chemistry 2024 |
Click here to see how this site uses Cookies. View our privacy policy here.