DOI:
10.1039/D4SC02214A
(Edge Article)
Chem. Sci., 2024,
15, 10392-10401
A Pd-catalyzed route to carborane-fused boron heterocycles†
Received
3rd April 2024
, Accepted 28th May 2024
First published on 28th May 2024
Abstract
Due to the expanding applications of icosahedral carboranes in medicinal and materials chemistry research, their functionalizations have become one of the central themes in boron-rich cluster chemistry. Although several strategies for incorporating nitrogen-containing nucleophiles on a single boron vertex of the icosahedral carboranes (C2B10H12) have been developed, methods for preparing clusters with vicinal B–N moieties are still lacking. The steric bulk of icosahedral carboranes and disparate electronic and steric nature of the N-containing groups have rendered the vicinal diamination challenging. In this article, we show how a developed Pd-catalyzed process is used to incorporate an array of NH-heterocycles, anilines, and heteroanilines with various electronic and steric profiles onto the vicinal boron vertices of a meta-carborane cluster via sequential or one-pot fashion. Importantly, oxidative cyclizations of the cross-coupling products with indoles and pyrroles appended to boron vertices generate a previously unknown class of all-boron-vertex bound carborane-fused six- and seven-membered ring heterocycles. Photophysical studies of the meta-carborane-fused heterocycles show that these structures can exhibit luminescence with high quantum yields and are amenable to further manipulations.
Introduction
Difunctionalization of two-dimensional aromatic compounds to generate vicinal C–N bonds has proved to be a powerful strategy to increase molecular complexity,1 and these ortho-diamine moieties have been found in natural products, pharmaceuticals, and multifunctional polymers.2 In these structures, arene or heteroarene rings are crucial for supporting the N-substituents, and the proximity of these polar groups is usually essential to confer high-affinity binding interactions with biological targets (Fig. 1A, left).3 Generally considered as three-dimensional analogs of benzene,4 but with significantly larger van der Waals volume (143 Å for meta-carborane and 79 Å for benzene),5 icosahedral closo-dicarbaboranes (colloquially referred to as carboranes) represent an important subset of boron-rich clusters and are emerging as interesting pharmacophore alternatives for drug discovery,5b,6 and especially boron neutron capture therapy (BNCT) agents that require a significant payload of boron.7 Functionalized carborane-based compounds can also present opportunities for the construction of new classes of functional materials and catalysts in which the inherent three-dimensionality of the building block is structurally and/or functionally necessary.8 Thus, site-selective boron vertex-difunctionalization of icosahedral carboranes to install vicinal NH-heterocycles and other N-containing nucleophiles is highly desired in new research frontiers (Fig. 1A, right).
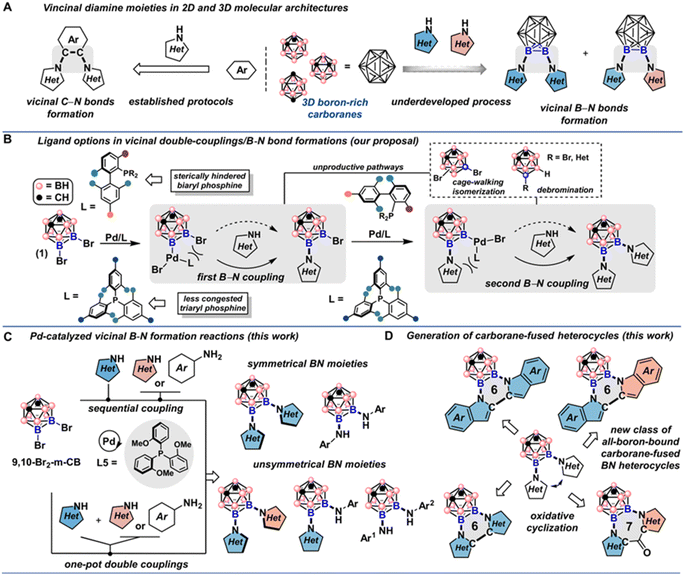 |
| Fig. 1 (A) Established preparations for o-phenylene diamine structures and synthetically challenging carborane vicinal diamine structures for generating N–B–B–N moieties. (B) In Pd-catalyzed diamination reactions using 9,10-Br2-m-carborane 1 and heterocycles, the sterically hindered biaryl monophosphine ligand could be detrimental to both coupling steps; triaryl phosphines serve as alternative less bulky ligands and lead to the desired products. (C) Our developed reaction sequence of preparing symmetrical and unsymmetrical diaminated products using the sequential or one-pot cross-coupling protocol using L5 as the optimal ligand. (D) The oxidative cyclization of the double cross-coupling products affords previously unknown all-boron-vertex-bound carborane-fused six- and seven-membered rings. | |
Early development of icosahedral carborane chemistry relied heavily on deprotonation reactions of relatively acidic C–H vertices (pKa ∼ 20 for icosahedral ortho-carborane) followed by the nucleophilic substitutions of the corresponding C-carboranyl species.9 Recently, two complementary strategies have emerged as the mainstay of icosahedral carborane functionalizations on boron-based vertices: transition metal-catalyzed carborane B–H bond activation10 and metal-catalyzed or mediated cross-coupling reactions between halogenated carboranes and nucleophiles.11 For the cross-coupling route, although the reaction pattern is reminiscent of transition metal-catalyzed cross-couplings between organic electrophiles and nucleophiles, the intrinsic low reactivity driven by steric bulk of the surface B–halogen bonds on carboranes has made these transformations uniquely challenging and distinct from their arene-based counterparts. Furthermore, considering that icosahedral ortho- and meta-carborane isomers have a distinct propensity to undergo nucleophilic deboronation, the resulting nido-carborane species have been shown to poison catalytic Pd-based species, presenting an additional challenge in this chemistry.12 Historically, boron-functionalized iodo-carboranes were first used as the coupling partners in Pd-catalyzed cross-coupling reactions due to the relatively weak B–I bond strength compared to the corresponding B–Br/Cl bonds, where Hawthorne and coworkers reported early examples of Pd-catalyzed amination of meta and ortho iodo-carboranes under mild conditions.13 In 2016, icosahedral B-bromocarboranes were discovered to undergo efficient Pd-catalyzed cross-coupling reactions by employing bulky electron-rich biaryl monophosphine ligands to support catalytically active species.12,14,15 Despite the demonstration that this catalytic reaction can engage B-bromocarboranes with several N-based and other nucleophiles, methods for incorporating vicinal B–N bond on carborane boron vertices are still challenging due to the steric bulk of the carborane scaffold and the steric hindrance from the incoming NH-heterocycles; and for the transition metal-catalyzed B–H bond activation strategy specifically, only one B–N bond can be produced using electrophilic aminating reagents and several electron-poor sulfonyl azides as the nitrogen source, leaving a large swath of electron-rich NH-heterocycles unavailable for the carborane B–N bond formation.16,11e
To achieve vicinal diamination of icosahedral carboranes, we employed 9,10-Br2-meta-carborane 1 as the model electrophile and explored the feasibility of double cross-coupling reactions with NH-heterocycles. In addition to the points described above, several underlying challenges in the context of the proposed cross-coupling chemistry need to be considered: (1) although beneficial in the amination of 9-Br-meta-carboranes, use of electron-rich biaryl mono-phosphine ligands could be deleterious in this double cross-coupling reaction due to the significant steric clash with the neighboring B–Br bond in the first B–N couplings as well as the B–N couplings in the second step; (2) potential B–Br bond isomerization (via cage-walking) and debromination pathways induced by the use of biaryl mono-phosphine ligands further complicate the reaction outcome (Fig. 1B, top).12,15 Nonetheless, many NH-heterocycles are considered weakly coordinating nucleophiles towards the Pd(II) center after the oxidative addition step, and bulky phosphine ligands are often required for successful reductive elimination.1c We surmised that the electron-rich tri-arylphosphine ligands could serve as competent ligand scaffolds to achieve the vicinal B–N bond formations (Fig. 1B, bottom). In this report, we have successfully identified a solution to the Pd-catalyzed double cross-couplings of 9,10-Br2-meta-carborane with diverse NH-heterocycles, anilines, and hetero-anilines. We showed that by utilizing a trisubstituted triarylphosphine ligand to support Pd-based catalysts, one can incorporate structurally diverse indoles, carbazoles, pyrroles and anilines onto the carborane neighboring B(9) and B(10) positions. Substrates containing additional coordinating sites such as pyrazoles and aminopyridines are compatible under the developed conditions. Importantly, symmetrical double cross-coupling products can be obtained with excess nucleophiles (Fig. 1C, top). Unsymmetrical doubly cross-coupled products can also be produced sequentially by isolating the monosubstituted intermediate and subjecting it to a second round of cross-coupling with a different nucleophile. Notably, two NH-heterocycles and anilines of disparate electronic and steric profiles can be installed in a one-pot fashion (Fig. 1C, bottom). Additionally, we were able to develop efficient protocols for oxidative couplings of the C-2 positions of vicinal indoles and pyrroles appended to B-based vertices in icosahedral meta-carboranes (Fig. 1D). This yielded previously unknown all-boron-vertex-bound carborane-fused six- and seven-membered ring heterocycles. Photophysical studies of carborane-fused heterocycles show that these new heterocyclic structures can exhibit high photoluminescence quantum yields.
Results and discussion
Ligand screening
By employing 9,10-Br2-meta-carborane 1 and indole N1 as the cross-coupling partners, we examined a series of mono- and di-phosphine ligands (Fig. 2, for details of the reaction screening, see Table S1†). Besides the desired product 2a, monosubstituted product 2b and debromination byproduct 3 were also obtained during the ligand screening. Biaryl monophosphine ligands L1–L3 all gave 2a in low yields. 2-Dicyclohexylphosphino-2′,4′,6′-triisopropylbiphenyl (Xphos, L1) generated slightly more 2b than when L2 and L3 were employed. Utilizing L4 bearing a carbazole group also produced 2b as the main product. Triarylphosphine ligands L5–L7 bearing different numbers of electron-rich groups led to divergent product distributions. For L5 with three ortho-methoxyl groups, 2a was obtained in 73% GC yield (75% isolated yield) with minimal by-product generation. In contrast, L7 with three ortho-methyl groups instead of OMe produced 2b in only 34% yield as the major product. To our surprise, diminished reactivity was observed when L6 with two additional MeO groups compared to L5 was tested. This result indicates that both electronic and steric factors intricately contribute to the efficiency of triarylphosphine ligands in the double cross-coupling reactions. Diphosphine ligand L8 gave 2a in low yields. For L9, higher selectivity for 2a than L8 was obtained, albeit with low reactivity.
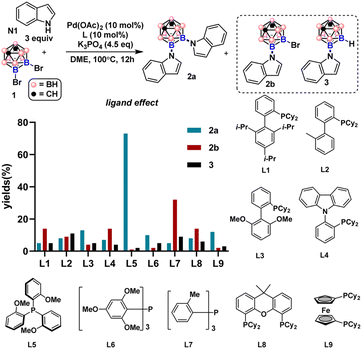 |
| Fig. 2 Ligand screening for the Pd-catalyzed double cross-coupling reactions between 1 and indole N1. Reaction conditions: 9,10-Br2-meta-carborane 1 (0.1 mmol, 1.0 equiv.), indole (0.3 mmol, 3 equiv.), 10 mol% Pd(OAc)2 (0.01 mmol, 10 mol%), 20 mol% ligands (0.02 mmol, 20 mol%) and 1 mL DME at 100 °C for 12 h under a N2 atmosphere. The yields were determined by GC-MS analysis using decane as an internal standard. | |
Substrate scope
Upon identifying L5 as a suitable ligand choice, we first evaluated the generality of the developed catalytic system in the symmetrical double cross-couplings (Fig. 3). Indole and carbazole derivatives bearing electron-rich and electron-deficient functional groups are suitable nucleophiles in this reaction, furnishing the desired vicinal B–N moieties with good to excellent yields. Substrates with methoxy and benzyloxy groups at indole C-5 positions (3a and 4a) gave 66% and 88% yields, respectively. Indole-5-carboxaldehyde is compatible under the developed conditions to give 6a in 32% yield. Trifluoromethylated and fluorinated indoles are converted to the double cross-coupled products 7a and 8a in moderate yields. Indoles with C-4 substitution are also suitable coupling partners (10a–13a). It is worth noting that boronic acid pinacol esters can withstand the cross-coupling conditions. The structures of the double cross-coupling products were verified by 1H, 11B/11B{1H}, and 13C{1H} spectroscopy and high-resolution mass spectrometry (HRMS). The structural identity was also independently confirmed by single crystal X-ray diffraction experiments. Indoles bearing C-4 and C-5 nitrile groups are successfully converted to the corresponding products 14a and 15a in moderate to good yields. Carbazole and its derivatives were suitable substrates, and difunctionalized products 18a–21a can be building blocks for potential applications in optical devices. The compatibility of this catalytic system with an array of indoles with diverse electronic features encouraged us to explore the functional group tolerance under the current conditions further. Sterically hindered indoles, challenging substrates in Pd-catalyzed C–N bond coupling reactions, were tested in the double cross-couplings with bulky B–Br bonds in 1. Under the standard conditions, 2-methyl indoles gave 22a and 23a in 60% and 68% yields, respectively. These results represent rare examples of cross-couplings of carborane-containing electrophiles with sterically hindered nucleophiles. Methyl pyrrole 2-carboxylate was another suitable NH-heterocycle besides indoles and carbazoles for the double cross-couplings to give 24a in 46% yield. Next, indoles with C-3 substitutions were examined. Reaction with ethyl indole-3-carboxylate gave 27a in 33% yields, suggesting that the weak nucleophiles are unfavorable in the double cross-couplings since NH moieties are more susceptible to electronic variations on the pyrrole ring of indole substrates. Selective B–N over B–O bond formation was obtained when tryptophol with a free hydroxyl group was applied under the standard conditions, and the desired product 26a was obtained in 73% yield. Under standard conditions, two 3,3′-diindolymethane (DIM) molecules were incorporated to produce 28a, and unprotected NH moieties are compatible under the developed reaction conditions. There are two types of NH units in melatonin and tryptophan methyl ester, and only NH from the pyrrole ring reacted with 9,10-Br2-meta-carborane to give 29a and 30a in 70% and 64% yields, respectively. Additionally, two electron-deficient anilines were also reactive to give disubstituted carborane products 31a and 32a in moderate yields. Excellent functional group compatibility during the substrate screening prompted us to investigate the limitations of this protocol further. By using 2-phenyl indole, only a monosubstitution product 33b was obtained in 36% yield. 2,4-Dimethyl pyrazole and 7-azaindole are challenging coupling partners for the symmetrical double cross-couplings. Complex reaction mixtures were observed, and we only isolated monosubstituted products 34b and 35b in low yields. The structural assignments of 34b and 35b were confirmed by single crystal X-ray crystallography. These results might be attributed to the extra basic nitrogen coordination sites for the Pd catalyst from pyrazole and 7-azaindole that impeded the second-round cross-coupling reactions.
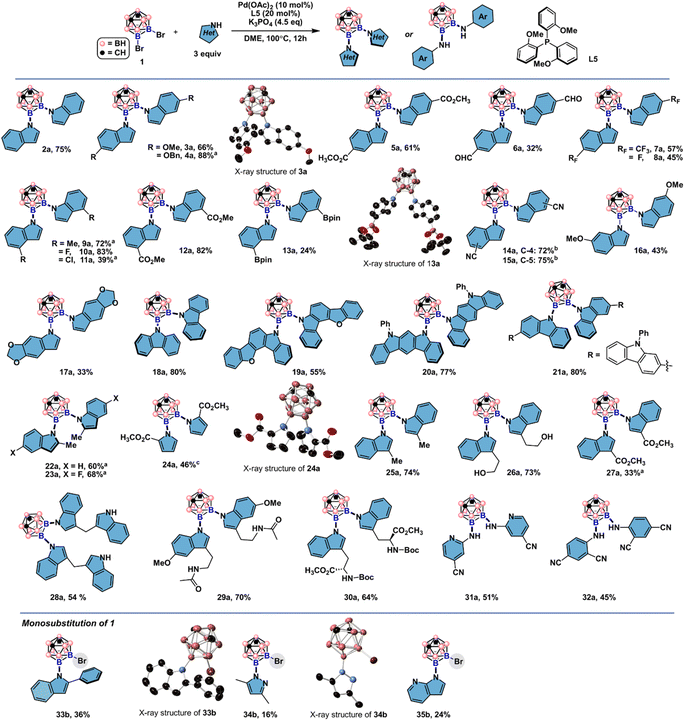 |
| Fig. 3 Substrate scope of Pd-catalyzed symmetrical double cross-coupling reactions. Electron-rich and -poor indoles are compatible under the developed conditions. Reaction conditions: 1 (0.2 mmol, 1 equiv.), heterocycle (0.6 mmol, 3 equiv.), Pd(OAc)2 (0.02 mmol, 10 mol%), L5 (0.04 mmol, 20 mol%), K3PO4 (0.9 mmol, 4.5 equiv.), DME (0.1 M, 2.0 mL), N2, 100 °C, 12 h. Ellipsoids at 50% probability and all H atoms in single crystal X-ray structures depicted are omitted for clarity. (a) Using t-BuONa as the base and dioxane as the solvent. (b) Using dioxane as the solvent. (c) Using 1,3-bis(dicyclohexylphosphino)propane as the ligand. See the ESI† for reaction details. | |
Unsymmetrical difunctionalization would be an ideal strategy for rapidly increasing icosahedral carborane diversity as building blocks in materials and three-dimensional pharmacophores.6m,7b,7i,8k However, existing methods are not capable of introducing two NH-heterocycles and anilines of different electronic and steric characteristics.16 First, we prepared the monosubstituted product 3b in 62% yield using 5-methoxyindole and 1.2 equivalents of 9,10-Br2-meta-carborane 1. Next, we tested an array of NH-heterocycles, including 3,5-dimethyl-pyrazole, methyl 2-pyrrolecarboxylate, substituted indoles, 7-azaindole, and aniline derivatives for the second-round cross-couplings. All tested coupling partners were successfully converted to the desired products in moderate to good yields (Fig. 4).
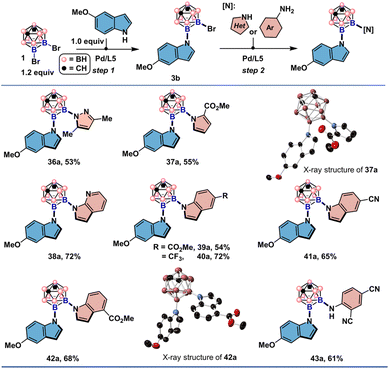 |
| Fig. 4 Substrate scope of sequential Pd-catalyzed un-symmetrical double cross-coupling reactions. See the ESI† for the reaction details. All H atoms in single crystal X-ray structures depicted are omitted for clarity. | |
The structures of 37a and 42a were also crystallographically determined which are consistent with the structural assignment from the proton and heteronuclear NMR spectroscopy. It is noteworthy that 3,5-dimethyl-pyrazole and 7-azaindole, unsuitable for symmetrical double couplings, were successfully incorporated by reacting with 3b under slightly tuned reaction conditions. With 2,4-dicyanoaniline as the second nucleophile, diaminated meta-carborane 43a was obtained in 61% yield. These encouraging results prompted us to explore whether unsymmetrical double cross-couplings could be achieved in a one-pot fashion (Fig. 5). The combination of electron-rich indoles, carbazole derivatives with electron-deficient indoles or anilines gave the unsymmetrically disubstituted carboranes in one step (44a–52a). Anilines of disparate electronic characteristics are also operational coupling combinations to produce the desired results (51a–52a). Exceptional functional group compatibility was displayed when tryptophol and tert-butyl carbamate was added in one pot as the nucleophiles. Both free hydroxyl from tryptophol and NH group from the carbamate were retained and can be subjected to further manipulations (50a).
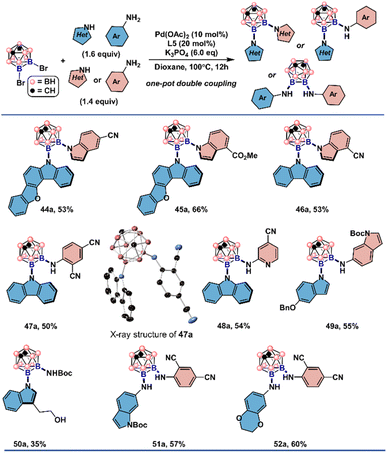 |
| Fig. 5 Substrate scope of one-pot Pd-catalyzed un-symmetrical double cross-coupling reactions. See the ESI† for the reaction details. All H atoms in single crystal X-ray structures depicted are omitted for clarity. | |
Synthesis of carborane-fused heterocycles
Several previous studies have been dedicated to generating icosahedral carborane-fused 2D carbo- and hetero-cycles as extensions of the carborane scaffolds.17 These systems are interesting as they can provide new insights into questions of electronic delocalization between 2D and 3D aromatic scaffolds and give rise to structurally new heterocyclic motifs.18 However, all-boron-vertex-bound icosahedral carborane-fused heterocycles bearing vicinal B–N bonds are still not accessible so far. We proposed that the spatial proximity of the indole and pyrrole C-2 positions after the double cross-couplings might be beneficial for the oxidative coupling reaction to synthesize a new class of all-boron-vertex-bound meta-carborane-fused heterocycles. By using I2 as the oxidant and dichloroethane as the solvent, icosahedral meta-carborane-fused six-membered heterocycles 53–58 were prepared in good to excellent yields from symmetrically and unsymmetrically cross-coupled products using indole and its derivatives (Fig. 6A). The X-ray single crystal structure of 53 suggests a coplanar geometry of the newly formed six-membered ring and neighboring indole moieties (Fig. 6B). The B(9)–B(10) bond length of the resulting heterocycle is shorter than that of its uncyclized counterparts (1.754(6) Å, compared to 1.825(2) Å for 42a and 1.8520(19) Å for 47a). The slight contraction of the B–B bond length may be attributed to the ring strain of the planar hexagon configuration. Both N(1)–B(9)–B(10)/N(2)–B(10)–B(9) angles are smaller than 120° (113.8° and 114.5°, respectively), and the deviation is more significant than C(2)–C(1)–N(1)/C(1)–C(2)–N(2) angles, which are slightly larger than 120° (122.6° and 123.2°, respectively). The co-planar geometry of 53 with the sum of internal hexagonal angles being nearly 720° could be indicative of some degree of aromatic features. The downfield shift of carborane CH proton signal from 3.04 ppm for 2a to 3.11 ppm for 53 suggests a small deshielding effect from the all-boron vertex bound six-membered heterocycle. It is noteworthy that 37a, containing one indole and one substituted pyrrole unit was also successfully cyclized to give 59 in excellent yield under the developed cyclization conditions. Interestingly, when substrate 24a with two C-2 methyl ester-substituted pyrroles was subjected to the same conditions, a formal Friedel–Crafts type acylation took place, and white solids with the carborane-fused seven-membered ring 60 were isolated and characterized by the NMR spectroscopy and single crystal X-ray crystallography. In the crystal structure, the B–B bond length of the heterocycle is 1.778(2) Å, longer than that of 53 (1.754(6) Å), indicating a reduced ring strain configuration. B(9)–N(1) is slightly longer than B(10)–N2 (1.5088(19) Å versus. 1.483(2) Å), which can be attributed to the steric and effects from the methyl ester group (Fig. 6C). The seven-membered ring bent towards the carborane moiety and the dihedral angle between the N(2)–B(10)–B(9)–N(1) and N(2)–N(1)–C(2) planes is 144°, strongly deviating from a coplanar geometry. When cerium(IV) ammonium nitrate (CAN) was used as the oxidant, a dark red solid was obtained. The structure of the dark red solid was identified as 61 with a carborane-fused six-membered ring and one nitro group on each pyrrole ring (Fig. 6D). In the crystal structure of 61, a twisted hexagon was obtained with 15° twisting angle. The B(9)–B(10) bond is the longest among the three crystal structures (1.794(3) Å compared to 1.778(2) Å for 60 and 1.754(6) Å for 53).
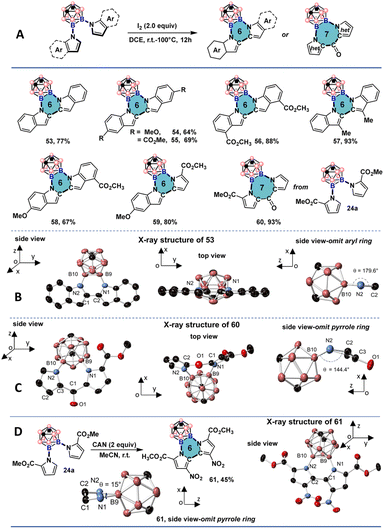 |
| Fig. 6 The developed protocols for the synthesis of all-boron-vertex-bound meta-carborane-fused heterocycles. (A) In the presence of I2, six- and seven-membered carborane-fused heterocycles are generated. (B) Different angles of view of the X-ray single crystal structure of 53, top and side views are provided. (C) Different angles of view of the X-ray single crystal structure of 60, top and side views are provided. (D) By using CAN, a dinitro-substituted six-membered heterocycle was obtained. All H atoms in single crystal X-ray structures depicted are omitted for clarity. | |
Photophysical studies
Having obtained the double cross-coupling products and carborane-fused heterocycles, we were keen to benchmark their fundamental photophysical properties. Photophysical data were gathered for compounds 12a, 42a, 56, and 58 as a representative series (Fig. 7 and S10†). As depicted in Fig. 7A, the absorption spectrum of the oxidative-cyclization product 56 in the dichloromethane solution exhibits a significant redshift compared to that of 12a. In addition, two new distinct fine-level absorption peaks emerge at 396 nm and 418 nm, while the low-energy absorption edge extends into the visible light range. This suggests an increase in the conjugation system of 56 relative to 12a, leading to the corresponding redshifted emission peaks (from 390 nm for 12a to 438 and 460 nm for 56). The prominent vibrational emission peaks of 56 at 438 and 460 nm indicate a reduced level of structural distortion in its excited state due to its significantly higher rigidity than that of 12a after oxidation-cyclization. Consequently, the corresponding absolute quantum yield (QY) in a dilute DCM solution also increases from 0.50 for 12a to 0.79 for 56. The absorption and emission spectra of both 12a and 56 exhibit significant overlap and a mirror relationship, respectively, and their emission lifetimes (τ) are in the range of a few ns. Additionally, their short Stokes shifts suggest a fluorescent nature for these radiative decays. The emission peak (388 nm) and shape of the 12a solid sample are generally consistent with its single-molecule fluorescence in dilute solution, while the emission peaks (470 and 500 nm) of the 56 solid shift noticeably to the red compared to its dilute solution (438 and 460 nm) (Fig. S16†). Moreover, the corresponding absolute QY of the 56 solid decreases more prominently than 12a (0.24 for the 12a solid and 0.05 for the 56 solid, see Table S15† for more details). This may be attributed to the well-arranged planar fused-ring structures in 56, which may tend to stack and exhibit aggregation-caused quenching (ACQ) effects in the solid state. For another pair of compounds, 42a and its cyclized derivative 58, a similar redshifted fluorescence pattern is observed for the unsymmetrically disubstituted product 42a and its cyclized form 58 (from 390 nm to 460 nm, see Fig. 7B). Compound 42a with 5-methoxy indole and 4-methyl indole carboxylate exhibits an absolute QY of 0.40 (τ = 5.6 ns), while the carborane-fused product 58 with a more rigid configuration yields an absolute QY of 0.82 (τ = 3.0 ns) in a dilute DCM solution. Overall, these results demonstrate that the new heterocyclic ring structures generated through connecting multiple boron vertices in carboranes via B–N bonds can exhibit different photoluminescence properties by changing substituents with diverse electronic effects on the NH-heterocycles.
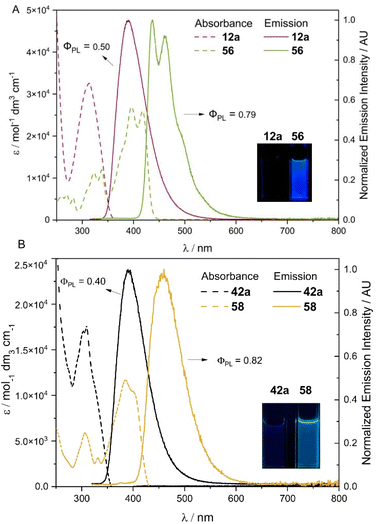 |
| Fig. 7 Electronic absorption (dashed line) and emission spectra (solid line, excitation at 310 nm) in CH2Cl2 solution (10−5 M) at room temperature in air, for compounds: (A) 12a and its corresponding oxidative cyclization product 56; (B) 42a and corresponding oxidative cyclization product 58. Photo insets are DCM solutions of 12a, 56, 42a and 58 (10−5 M, irradiated with UV light, λexc = 365 nm). | |
Conclusions
We have identified an efficient catalytic system using a Pd-catalyst supported by the tris(o-methoxyphenyl)phosphine ligand as the optimal system to synthesize a wide array of densely functionalized icosahedral meta-carboranes with vicinal B–N moieties. In this transformation, a variety of NH-heterocycles such as electron-rich and deficient indoles, carbazole and its derivatives, pyrroles and pyrazoles are compatible under the developed conditions. Anilines and heteroanilines are also competent cross-coupling partners in this transformation. Unsymmetrical double cross-couplings were achieved in both sequential and one-pot fashion. Sterically hindered indoles are also tolerated under the current conditions to generate disubstituted products. The developed strategy provides unprecedented functional group and steric tolerance compared to existing icosahedral carborane functionalization chemistry and sets the groundwork for poly-functionalization chemistry of icosahedral carboranes via metal-catalyzed cross-coupling chemistry. More importantly, oxidative cyclization of the cross-coupling products has allowed for the generation of a new class of previously inaccessible all-boron-vertex bound six- and seven-membered icosahedral carborane-fused heterocycles using either transition metal-catalyzed B–H bond functionalization or cross-coupling strategies. X-ray crystallographic analysis of the heterocycles disclosed several different structural configurations presented in these heterocyclic rings including coplanar, twisted six-membered ring and non-planar seven-membered ring structures. Photophysical investigations of the icosahedral meta-carborane-fused heterocycles indicated that these systems can exhibit luminescence with high quantum yields and are potentially amenable for extensive tuning resulting from the identity of the heterocycle appended onto a boron cluster cage. This work shows how metal-catalyzed cross-coupling can be applied towards unconventional and challenging substrates leading to the formation of never-before-seen heterocyclic motifs with interesting photophysical properties.
Data availability
The datasets associated with this article are available in the ESI.† CCDC 2323379 (3a), 2323380 (13a), 2323386 (24a), 2323387 (33b), 2323388 (34b), 2323389 (37a), 2323390 (42a), 2323391 (47a), 2323393 (53), 2323397 (60), 2323399 (61) contain the supplementary crystallographic data for this paper.†
Author contributions
X. M. designed and supervised the project. M. Z. performed reaction condition optimizations. M. Z. and P. W. explored the substrate scope. C. Z. directed photophysical analysis. Z. W. collected the photophysical data. Y. Z., L. S. and Y. X. helped in collecting some experimental data. M. Z., P. W. and Z. W. prepared the ESI.† X. M., A. S. and C. Z. wrote and edited the manuscript. All authors analyzed the data and commented on the manuscript during its preparation.
Conflicts of interest
There are no conflicts to declare.
Acknowledgements
Support for this study was provided by Natural Science Foundation of Shanghai (22ZR1416700) and start-up fund from Songshan Lake materials Laboratory (Y1D1061C11). We thank Prof. Guosheng Liu and Dr Haoyang Wang from Shanghai Institute of Organic Chemistry for fruitful discussions. We are thankful for the financial support from Engineering Research Center of Pharmaceutical Process Chemistry, Ministry of Education.
Notes and references
- For reviews on generation of aryl C–N bonds see:
(a)
F. Terrier, Modern Nucleophilic Aromatic Substitution, Wiley-VCH, Weinheim, Germany, 2013 CrossRef;
(b) C. Sambiagio, S. P. Marsden, A. J. Blacker and P. C. McGowan, Chem. Soc. Rev., 2014, 43, 3525–3550 RSC;
(c) P. Ruiz-Castillo and S. L. Buchwald, Chem. Rev., 2016, 116, 12564–12649 CrossRef CAS PubMed;
(d) R. Dorel, C. P. Grugel and A. M. Haydl, Angew. Chem., Int. Ed., 2019, 58, 17118–17129 CrossRef CAS PubMed . For recent examples of 1,2-aryl diamine synthesis, see: ;
(e) S. Gonell, M. Poyatos and E. Peris, Angew. Chem., Int. Ed., 2013, 52, 7009–7013 CrossRef CAS PubMed;
(f) L. Li, D. Qiu, J. Shi and Y. Li, Org. Lett., 2016, 18, 3726–3729 CrossRef CAS PubMed;
(g) M. Xiong, Z. Gao, X. Liang, P. Cai, H. Zhu and Y. Pan, Chem. Commun., 2018, 54, 9679–9682 RSC;
(h) R. Sánchez-Bento, B. Roure, J. Llaveria, A. Ruffoni and D. Leonori, Chem, 2023, 9, 3685–3695 CrossRef.
- For recent examples of vicinal diaminated arenes in medicinal research, see:
(a) D. J. O'Neill, A. Adedoyin, J. A. Bray, D. C. Deecher, A. Fensome, J. A. Goldberg, J. Harrison, L. Leventhal, C. Mann, L. Mark, L. Nogle, N. R. Sullivan, T. B. Spangler, E. A. Terefenko, E. J. Trybulski, A. J. Uveges, A. Vu, G. T. Whiteside and P. Zhang, J. Med. Chem., 2011, 54, 6824–6831 CrossRef PubMed;
(b) M. W. Karaman, S. Herrgard, D. K. Treiber, P. Gallant, C. E. Atteridge, B. T. Campbell, K. W. Chan, P. Ciceri, M. I. Davis, P. T. Edeen, R. Faraoni, M. Floyd, J. P. Hunt, D. J. Lockhart, Z. V. Milanov, M. J. Morrison, G. Pallares, H. K. Patel, S. Pritchard, L. M. Wodicka and P. P. Zarrinkar, Nat. Biotechnol., 2008, 26, 127–132 CrossRef CAS PubMed . For reviews and recent applications of ortho-phenylene diamines in functional polymers, see: ;
(c) X.-G. Li, M.-R. Huang, W. Duan and Y.-L. Yang, Chem. Rev., 2002, 102, 2925–3030 CrossRef CAS PubMed;
(d) Q. L. Guan, Y. Sun, R. Huo, Y. Xin, F. Y. Bai, Y. H. Xing and L. X. Sun, Inorg. Chem., 2021, 60, 2829–2838 CrossRef CAS PubMed;
(e) H. Zhang, S. H. Lam, Y. Guo, J. Yang, Y. Lu, L. Shao, B. Yang, L. Xiao and J. Wang, ACS Appl. Mater. Interfaces, 2021, 13, 51855–51866 CrossRef CAS PubMed;
(f) S.-W. Kim, W. K. Lee and J.-S. Lee, ACS Omega, 2023, 8, 46267–46275 CrossRef CAS PubMed.
- D. C. Blakemore, L. Castro, I. Churcher, D. C. Rees, A. W. Thomas, D. M. Wilson and A. Wood, Nat. Chem., 2018, 10, 383–394 CrossRef CAS PubMed.
-
(a)
W. N. Lipscomb, Boron Hydrides, Benjamin, New York, 1963 Search PubMed;
(b) P. V. R. Schleyer and K. Najafian, Inorg. Chem., 1998, 37, 3454–3470 CrossRef CAS PubMed;
(c) Z. Chen and R. B. King, Chem. Rev., 2005, 105, 3613–3642 CrossRef CAS PubMed;
(d) R. B. King, Chem. Rev., 2001, 101, 1119–1152 CrossRef CAS PubMed.
-
(a) Y. H. Zhao, M. H. Abraham and A. M. Zissimos, J. Org. Chem., 2003, 68, 7368–7373 CrossRef CAS PubMed;
(b) M. Scholz and E. Hey-Hawkins, Chem. Rev., 2011, 111, 7035–7062 CrossRef CAS PubMed.
- For recent reviews on carboranes in medicinal research, see:
(a) F. Issa, M. Kassiou and L. M. Rendina, Chem. Rev., 2011, 111, 5701–5722 CrossRef CAS PubMed;
(b) G. Detlef, Pure Appl. Chem., 2015, 87, 173–179 CrossRef;
(c) Z. J. Leśnikowski, J. Med. Chem., 2016, 59, 7738–7758 CrossRef PubMed;
(d) A. Marfavi, P. Kavianpour and L. M. Rendina, Nat. Rev. Chem., 2022, 6, 486–504 CrossRef PubMed . For selected recent applications of carboranes and boron clusters in medicinal chemistry, see: ;
(e) R. L. Julius, O. K. Farha, J. Chiang, L. J. Perry and M. F. Hawthorne, Proc. Natl. Acad. Sci. U. S. A., 2007, 104, 4808–4813 CrossRef CAS PubMed;
(f) M. L. Beer, J. Lemon and J. F. Valliant, J. Med. Chem., 2010, 53, 8012–8020 CrossRef CAS PubMed;
(g) T. Goto, K. Ohta, S. Fujii, S. Ohta and Y. Endo, J. Med. Chem., 2010, 53, 4917–4926 CrossRef CAS PubMed;
(h) S. Fujii, K. Ohta, T. Goto, A. Oda, H. Masuno, Y. Endo and H. Kagechika, Med. Chem. Commun., 2012, 3, 680–684 RSC;
(i) S. Fujii, A. Kano, C. Songkram, H. Masuno, Y. Taoda, E. Kawachi, T. Hirano, A. Tanatani and H. Kagechika, Biorg. Med. Chem., 2014, 22, 1227–1235 CrossRef CAS PubMed;
(j) S. M. Wilkinson, H. Gunosewoyo, M. L. Barron, A. Boucher, M. McDonnell, P. Turner, D. E. Morrison, M. R. Bennett, I. S. McGregor, L. M. Rendina and M. Kassiou, ACS Chem. Neurosci., 2014, 5, 335–339 CrossRef CAS PubMed;
(k) J. Balintová, A. Simonova, M. Białek-Pietras, A. Olejniczak, Z. J. Lesnikowski and M. Hocek, Bioorg. Med. Chem. Lett., 2017, 27, 4786–4788 CrossRef PubMed;
(l) Y. Asawa, K. Nishida, K. Kawai, K. Domae, H. S. Ban, A. Kitazaki, H. Asami, J.-Y. Kohno, S. Okada, H. Tokuma, D. Sakano, S. Kume, M. Tanaka and H. Nakamura, Bioconjug. Chem., 2021, 32, 2377–2385 CrossRef CAS PubMed;
(m) D. Kodr, C. P. Yenice, A. Simonova, D. P. Saftić, R. Pohl, V. Sýkorová, M. Ortiz, L. Havran, M. Fojta, Z. J. Lesnikowski, C. K. O Sullivan and M. Hocek, J. Am. Chem. Soc., 2021, 143, 7124–7134 CrossRef CAS PubMed;
(n) R. Varkhedkar, F. Yang, R. Dontha, J. Zhang, J. Liu, B. Spingler, S. Van der Veen and S. Duttwyler, ACS Cent. Sci., 2022, 8, 322–331 CrossRef CAS PubMed;
(o) B. B. Jei, L. Yang and L. Ackermann, Chem.–Eur. J., 2022, 28, e202200811 CrossRef CAS PubMed;
(p) A. R. Genady, J. Tan, M. E. El-Zaria, A. Zlitni, N. Janzen and J. F. Valliant, J. Organomet. Chem., 2015, 791, 204–213 CrossRef CAS;
(q) A. S. Louie, N. Vasdev and J. F. Valliant, J. Med. Chem., 2011, 54, 3360–3367 CrossRef CAS PubMed.
- For recent reviews on BNCT treatment using carboranes, see:
(a) G. Calabrese, A. Daou, E. Barbu and J. Tsibouklis, Drug Discov. Today, 2018, 23, 63–75 CrossRef CAS PubMed;
(b) D. J. Worm, P. Hoppenz, S. Els-Heindl, M. Kellert, R. Kuhnert, S. Saretz, J. Köbberling, B. Riedl, E. Hey-Hawkins and A. G. Beck-Sickinger, J. Med. Chem., 2020, 63, 2358–2371 CrossRef CAS PubMed;
(c) M. A. Dymova, S. Y. Taskaev, V. A. Richter and E. V. Kuligina, Cancer Commun., 2020, 40, 406–421 CrossRef PubMed;
(d) F. Ali, N. S. Hosmane and Y. Zhu, Molecules, 2020, 25, 828 CrossRef CAS PubMed;
(e) T. D. Malouff, D. S. Seneviratne, D. K. Ebner, W. C. Stross, M. R. Waddle, D. M. Trifiletti and S. Krishnan, Front. Oncol., 2021, 11 DOI:10.3389/fonc.2021.601820 , Review.;
(f) Y. Chen, F. Du, L. Tang, J. Xu, Y. Zhao, X. Wu, M. Li, J. Shen, Q. Wen, C. H. Cho and Z. Xiao, Mol. Ther. Oncolytics., 2022, 24, 400–416 CrossRef CAS PubMed;
(g) T. D. Marforio, A. Carboni and M. Calvaresi, Cancers, 2023, 15, 4944 CrossRef CAS PubMed . For selected examples: see: ;
(h) L. Evangelista, G. Jori, D. Martini and G. Sotti, Appl. Radiat. Isot., 2013, 74, 91–101 CrossRef CAS PubMed;
(i) G. Chen, J. Yang, G. Lu, P. C. Liu, Q. Chen, Z. Xie and C. Wu, Mol. Pharm., 2014, 11, 3291–3299 CrossRef CAS PubMed;
(j) P. Hoppenz, S. Els-Heindl, M. Kellert, R. Kuhnert, S. Saretz, H.-G. Lerchen, J. Köbberling, B. Riedl, E. Hey-Hawkins and A. G. Beck-Sickinger, J. Org. Chem., 2020, 85, 1446–1457 CrossRef CAS PubMed;
(k) R. Li, J. Zhang, J. Guo, Y. Xu, K. Duan, J. Zheng, H. Wan, Z. Yuan and H. Chn, Mol. Pharm., 2020, 17, 202–211 CrossRef CAS PubMed;
(l) F. Zhao, K. Hu, C. Shao and G. Jin, Polym. Test., 2021, 100, 107269 CrossRef CAS.
- For selected recent applications in materials science, see:
(a) B. P. Dash, R. Satapathy, E. R. Gaillard, K. M. Norton, J. A. Maguire, N. Chug and N. S. Hosmane, Inorg. Chem., 2011, 50, 5485–5493 CrossRef CAS PubMed;
(b) A. M. Spokoyny, C. W. Machan, D. J. Clingerman, M. S. Rosen, M. J. Wiester, R. D. Kennedy, C. L. Stern, A. A. Sarjeant and C. A. Mirkin, Nat. Chem., 2011, 3, 590–596 CrossRef CAS PubMed;
(c) K.-R. Wee, Y.-J. Cho, S. Jeong, S. Kwon, J.-D. Lee, I.-H. Suh and S. O. Kang, J. Am. Chem. Soc., 2012, 134, 17982–17990 CrossRef CAS PubMed;
(d) A. M. Spokoyny, C. D. Lewis, G. Teverovskiy and S. L. Buchwald, Organometallics, 2012, 31, 8478–8481 CrossRef CAS PubMed;
(e) C. A. Lugo, C. E. Moore, A. L. Rheingold and V. Lavallo, Inorg. Chem., 2015, 54, 2094–2096 CrossRef CAS PubMed;
(f) H. Naito, Y. Morisaki and Y. Chujo, Angew. Chem., Int. Ed., 2015, 54, 5084–5087 CrossRef CAS PubMed;
(g) J. C. Axtell, O. Kirlikovali, P. I. Djurovich, D. Jung, V. T. Nguyen, B. Munekiyo, A. T. Royappa, A. L. Rheingold and A. M. Spokoyny, J. Am. Chem. Soc., 2016, 138, 15758–15765 CrossRef CAS PubMed;
(h) H. A. Mills, C. G. Jones, K. P. Anderson, A. D. Ready, P. I. Djurovich, S. I. Khan, J. N. Hohman, H. M. Nelson and A. M. Spokoyny, Chem. Mater., 2022, 34, 6933–6943 CrossRef CAS;
(i) M. Y. Tsang, S. Rodríguez-Hermida, K. C. Stylianou, F. Tan, D. Negi, F. Teixidor, C. Viñas, D. Choquesillo-Lazarte, C. Verdugo-Escamilla, M. Guerrero, J. Sort, J. Juanhuix, D. Maspoch, J. G. Planas, J. Sort, J. Juanhuix, D. Maspoch and J. G. Planas, Cryst. Growth Des., 2017, 17, 846–857 CrossRef CAS;
(j) E. Oleshkevich, F. Teixidor, A. Rosell and C. Viñas, Inorg. Chem., 2018, 57, 462–470 CrossRef CAS PubMed;
(k) K. L. Martin, J. N. Smith, E. R. Young and K. R. Carter, Macromolecules, 2019, 52, 7951–7960 CrossRef CAS;
(l) L. Gan, A. Chidambaram, P. G. Fonquernie, M. E. Light, D. Choquesillo-Lazarte, H. Huang, E. Solano, J. Fraile, C. Viñas, F. Teixidor, J. A. R. Navarro, K. C. Stylianou and J. G. Planas, J. Am. Chem. Soc., 2020, 142, 8299–8311 CrossRef CAS PubMed;
(m) D. Tu, J. Li, F. Sun, H. Yan, J. Poater and M. Solà, JACS Au, 2021, 1, 2047–2057 CrossRef CAS PubMed;
(n) J. Ochi, K. Tanaka and Y. Chujo, Inorg. Chem., 2021, 60, 8990–8997 CrossRef CAS PubMed;
(o) M. A. Waddington, X. Zheng, J. M. Stauber, E. Hakim Moully, H. R. Montgomery, L. M. A. Saleh, P. Král and A. M. Spokoyny, J. Am. Chem. Soc., 2021, 143, 8661–8668 CrossRef CAS PubMed;
(p) L. Gan, E. Andres-Garcia, G. Mínguez Espallargas and J. G. Planas, ACS Appl. Mater. Interfaces, 2023, 15, 5309–5316 CrossRef CAS PubMed;
(q) X. Xu, Q. Cui, H. Chen and N. Huang, J. Am. Chem. Soc., 2023, 145, 24202–24209 CrossRef CAS PubMed;
(r) K. B. Idrees, K. O. Kirlikovali, C. Setter, H. Xie, H. Brand, B. Lal, F. Sha, C. S. Smoljan, X. Wang, T. Islamoglu, L. M. Macreadie and O. K. Farha, J. Am. Chem. Soc., 2023, 145, 23433–23441 CrossRef CAS PubMed;
(s) P.-F. Cui, X.-R. Liu and G.-X. Jin, J. Am. Chem. Soc., 2023, 145, 19440–19457 CrossRef CAS PubMed;
(t) L. Gan, M. T. Nord, J. M. Lessard, N. Q. Tufts, A. Chidambaram, M. E. Light, H. Huang, E. Solano, J. Fraile, F. Suárez-García, C. Viñas, F. Teixidor, K. C. Stylianou and J. G. Planas, J. Am. Chem. Soc., 2023, 145, 13730–13741 CrossRef CAS PubMed;
(u) K. Liu, J. Zhang, Q. Shi, L. Ding, T. Liu and Y. Fang, J. Am. Chem. Soc., 2023, 145, 7408–7415 CrossRef CAS PubMed;
(v) C. N. Kona, R. Oku, S. Nakamura, M. Miura, K. Hirano and Y. Nishii, Chem, 2023, 10, 402–413 CrossRef;
(w) F. Sun, S. Tan, H.-J. Cao, J. Xu, V. I. Bregadze, D. Tu, C. Lu and H. Yan, Angew. Chem., Int. Ed., 2022, 61, e202207125 CrossRef CAS PubMed;
(x) A. W. Tomich, J. Park, S.-B. Son, E. P. Kamphaus, X. Lyu, F. Dogan, V. Carta, J. Gim, T. Li, L. Cheng, E. Lee, V. Lavallo and C. S. Johnson, Angew. Chem., Int. Ed., 2022, 61, e202208158 CrossRef CAS PubMed;
(y) R. Jay, A. W. Tomich, J. Zhang, Y. Zhao, A. De Gorostiza, V. Lavallo and J. Guo, ACS Appl. Mater. Interfaces, 2019, 11, 11414–11420 CrossRef CAS PubMed;
(z) M. Zhong, J. Zhou, H. Fang and P. Jena, Phys. Chem. Chem. Phys., 2017, 19, 17937–17943 RSC.
-
R. N. Grimes, Carboranes, Academic Press, New York, 3rd edn, 2016 Search PubMed.
- For reviews on the direct carborane B–H functionalizations, see:
(a) Z. Qiu and Z. Xie, Acc. Chem. Res., 2021, 54, 4065–4079 CrossRef CAS PubMed;
(b) Y. K. Au and Z. Xie, Bull. Chem. Soc. Jpn., 2021, 94, 879–899 CrossRef CAS;
(c) J. Zhang and Z. Xie, Sci. Sin. Chim., 2023, 53, 312–319 CrossRef;
(d) J. Zhang and Z. Xie, Adv. Catal., 2022, 91–167 CrossRef;
(e) Y. Quan and X. Xie, Chem. Soc. Rev., 2019, 48, 3660–3673 RSC;
(f) X. Zhang and H. Yan, Coord. Chem. Rev., 2019, 378, 466–482 CrossRef CAS;
(g) S. Duttwyler, Pure Appl. Chem., 2018, 90, 733–744 CrossRef CAS . For selected recent examples, see: ;
(h) H. Ren, P. Zhang, J. Xu, W. Ma, D. Tu, C.-S. Lu and H. Yan, J. Am. Chem. Soc., 2023, 145, 7638–7647 CrossRef CAS PubMed;
(i) M. Chen, J. Xu, D. Zhao, F. Sun, S. Tian, D. Tu, C. Lu and H. Yan, Angew. Chem., Int. Ed., 2022, 61, e202205672 CrossRef CAS PubMed;
(j) Y.-N. Ma, H. Ren, Y. Wu, N. Li, F. Chen and X. Chen, J. Am. Chem. Soc., 2023, 145, 7331–7342 CrossRef CAS PubMed;
(k) S. Li and Z. Xie, J. Am. Chem. Soc., 2022, 144, 7960–7965 CrossRef CAS PubMed;
(l) H. A. Mills, J. L. Martin, A. L. Rheingold and A. M. Spokoyny, J. Am. Chem. Soc., 2020, 142, 4586–4591 CrossRef CAS PubMed;
(m) R. Cheng, B. Li, J. Wu, J. Zhang, Z. Qiu, W. Tang, S.-L. You, Y. Tang and Z. Xie, J. Am. Chem. Soc., 2018, 140, 4508–4511 CrossRef CAS PubMed;
(n) R. Cheng, J. Zhang, H. Zhang, Z. Qiu and Z. Xie, Nat. Commun., 2021, 12, 7146 CrossRef CAS PubMed;
(o) H. Lyu, J. Zhang, J. Yang, Y. Quan and Z. Xie, J. Am. Chem. Soc., 2019, 141, 4219–4224 CrossRef CAS PubMed;
(p) F. Lin, J.-L. Yu, Y. Shen, S.-Q. Zhang, B. Spingler, J. Liu, X. Hong and S. Duttwyler, J. Am. Chem. Soc., 2018, 140, 13798–13807 CrossRef CAS PubMed;
(q) Y. Zhang, T. Wang, L. Wang, Y. Sun, F. Lin, J. Liu and S. Duttwyler, Chem.–Eur. J., 2018, 24, 15812–15817 CrossRef CAS PubMed;
(r) Y.-F. Liang, L. Yang, B. B. Jei, R. Kuniyil and L. Ackermann, Chem. Sci., 2020, 11, 10764–10769 RSC;
(s) P.-F. Cui, X.-R. Liu, S.-T. Guo, Y.-J. Lin and G.-X. Jin, J. Am. Chem. Soc., 2021, 143, 5099–5105 CrossRef CAS PubMed;
(t) F. Lin, J.-L. Yu, Y. Shen, S.-Q. Zhang, B. Spingler, J. Liu, X. Hong and S. Duttwyler, J. Am. Chem. Soc., 2018, 140, 13798–13807 CrossRef CAS PubMed;
(u) Y. Baek, K. Cheong, G. H. Ko, G. U. Han, S. H. Han, D. Kim, K. Lee and P. H. Lee, J. Am. Chem. Soc., 2020, 142, 9890–9895 CrossRef CAS PubMed.
- For recent reviews on cross-couplings with halogenated carboranes and boron clusters, see:
(a) R. M. Dziedzic and A. M. Spokoyny, Chem. Commun., 2019, 55, 430–442 RSC;
(b) Y. Ge, J. Zhang, Z. Qiu and Z. Xie, Dalton Trans., 2021, 50, 1766–1773 RSC;
(c) M. K. Al-Joumhawy, T. Marei, A. Shmalko, P. Cendoya, J. La Borde and D. Gabel, Chem. Commun., 2021, 57, 10007–10010 RSC;
(d) K. P. Anderson, P. I. Djurovich, V. P. Rubio, A. Liang and A. M. Spokoyny, Inorg. Chem., 2022, 61, 15051–15057 CrossRef CAS PubMed;
(e) X. Mu, M. Hopp, R. M. Dziedzic, M. A. Waddington, A. L. Rheingold, E. M. Sletten, J. C. Axtell and A. M. Spokoyny, Organometallics, 2020, 39, 4380–4386 CrossRef CAS PubMed;
(f) S. Li, Y. Liu and Z. Xie, Chin. J. Chem., 2023, 42, 129–134 CrossRef CAS;
(g) Y. Ge, J. Zhang, Z. Qiu and Z. Xie, Angew. Chem., Int. Ed., 2020, 59, 4851–4855 CrossRef CAS PubMed.
-
(a) M. A. Fox and K. Wade, J. Organomet. Chem., 1999, 573, 279–291 CrossRef CAS;
(b) J. Yoo, J.-W. Hwang and Y. Do, Inorg. Chem., 2001, 40, 568–570 CrossRef CAS PubMed;
(c) R. M. Dziedzic, J. C. Axtell, A. L. Rheingold and A. M. Spokoyny, Org. Process Res. Dev., 2019, 23, 1638–1645 CrossRef CAS PubMed.
-
(a) W. J. Marshall, R. J. Young and V. V. Grushin, Organometallics, 2001, 20, 523–533 CrossRef CAS;
(b) K. Ishita, A. Khalil, R. Tiwari, J. Gallucci and W. Tjarks, Eur. J. Inorg. Chem., 2018, 24, 2821–2825 CrossRef;
(c) Y. Sevryugina, R. L. Julius and M. F. Hawthorne, Inorg. Chem., 2010, 49, 10627–10634 CrossRef CAS PubMed.
- R. M. Dziedzic, L. M. A. Saleh, J. C. Axtell, J. L. Martin, S. L. Stevens, A. T. Royappa, A. L. Rheingold and A. M. Spokoyny, J. Am. Chem. Soc., 2016, 138, 9081–9084 CrossRef CAS PubMed.
- R. M. Dziedzic, J. L. Martin, J. C. Axtell, L. M. A. Saleh, T.-C. Ong, Y.-F. Yang, M. S. Messina, A. L. Rheingold, K. N. Houk and A. M. Spokoyny, J. Am. Chem. Soc., 2017, 139, 7729–7732 CrossRef CAS PubMed.
-
(a) Y.-N. Ma, Y. Gao, Y. Ma, Y. Wang, H. Ren and X. Chen, J. Am. Chem. Soc., 2022, 144, 8371–8378 CrossRef CAS PubMed;
(b) J. Zhang and Z. Xie, Angew. Chem., Int. Ed., 2022, 61, e202202675 CrossRef CAS PubMed;
(c) Y. Ki Au, Q. Ma, J. Zhang and Z. Xie, Chem.–Asian J., 2023, 18, e202300611 CrossRef CAS PubMed;
(d) Y. K. Au, J. Zhang, Y. Quan and Z. Xie, J. Am. Chem. Soc., 2021, 143, 4148–4153 CrossRef CAS PubMed;
(e) A. Kataki-Anastasakou, J. C. Axtell, S. Hernandez, R. M. Dziedzic, G. J. Balaich, A. L. Rheingold, A. M. Spokoyny and E. M. Sletten, J. Am. Chem. Soc., 2020, 142, 20513–20518 CrossRef CAS PubMed;
(f) H. Lyu, Y. Quan and Z. Xie, J. Am. Chem. Soc., 2016, 138, 12727–12730 CrossRef CAS PubMed;
(g) G. U. Han, Y. Baek, K. Lee, S. Shin, H. Noh and P. H. Chan Lee, Org. Lett., 2021, 23, 416–420 CrossRef CAS PubMed;
(h) H. Lyu and Z. Xie, Chem. Commun., 2022, 58, 8392–8395 RSC.
- For recent examples, see:
(a) C. Maeng, G. H. Ko, H. Yang, S. H. Han, G. U. Han, H. Noh, K. Chan Lee, D. Kim and P. H. Lee, Org. Lett., 2022, 24, 3526–3531 CrossRef CAS PubMed;
(b) J. Zhang and Z. Xie, Chem. Sci., 2021, 12, 5616–5620 RSC;
(c) C. X. Cui, J. Zhang, Z. Qiu and Z. Xie, Dalton Trans., 2020, 49, 1380–1383 RSC;
(d) R. Cheng, Z. Qiu and Z. Xie, Chin. J. Chem., 2020, 38, 1575–1578 CrossRef CAS;
(e) R. Zhang, Y. Yuan, Z. Qiu and Z. Xie, Chin. J. Chem., 2018, 36, 273–279 CrossRef CAS;
(f) J. Zhang, Z. Qiu and Z. Xie, Organometallics, 2017, 36, 3806–3811 CrossRef CAS;
(g) D. Zhao, J. Zhang and Z. Xie, J. Am. Chem. Soc., 2015, 137, 13938–13942 CrossRef CAS PubMed;
(h) S. Ren, Z. Qiu and Z. Xie, Organometallics, 2013, 32, 4292–4300 CrossRef CAS;
(i) J. H. Wright, C. E. Kefalidis, F. S. Tham, L. Maron and V. Lavallo, Inorg. Chem., 2013, 52, 6223–6229 CrossRef CAS PubMed;
(j) M. Asay, C. E. Kefalidis, J. Estrada, D. S. Weinberger, J. Wright, C. E. Moore, A. L. Rheingold, L. Maron and V. Lavallo, Angew. Chem., Int. Ed., 2013, 52, 11560–11563 CrossRef CAS PubMed;
(k) S. Yruegas, J. C. Axtell, K. O. Kirlikovali, A. M. Spokoyny and C. D. Martin, Chem. Commun., 2019, 55, 2892–2895 RSC;
(l) Y. Nie, J. Miao, H. Wadepohl, H. Pritzkow, T. Oeser and W. Siebert, Z. Anorg. Allg. Chem., 2013, 639, 1188–1193 CrossRef CAS;
(m) H. Zhang, J. Wang, W. Yang, L. Xiang, W. Sun, W. Ming, Y. Li, Z. Lin and Q. Ye, J. Am. Chem. Soc., 2020, 142, 17243–17249 CrossRef CAS PubMed;
(n) C. Zhang, J. Wang, W. Su, Z. Lin and Q. Ye, J. Am. Chem. Soc., 2021, 143, 8552–8558 CrossRef CAS PubMed;
(o) J. Krebs, A. Häfner, S. Fuchs, X. Guo, F. Rauch, A. Eichhorn, I. Krummenacher, A. Friedrich, L. Ji, M. Finze, Z. Lin, H. Braunschweig and T. B. Marder, Chem. Sci., 2022, 13, 14165–14178 RSC;
(p) J. Wang, L. Xiang, X. Liu, A. Matler, Z. Lin and Q. Ye, Chem. Sci., 2024, 15, 4839–4845 RSC;
(q) Y. Li, M. Tamizmani, M. O. Akram and C. D. Martin, Chem. Sci., 2024, 10, 10.1039/D4SC00990H.
-
(a) J. Poater, C. Vinas, M. Sola and F. Teixidor, Nat. Commun., 2022, 13, 3844 CrossRef CAS PubMed;
(b) T. L. Chan and Z. Xie, Chem. Sci., 2018, 9, 2284–2289 RSC.
|
This journal is © The Royal Society of Chemistry 2024 |
Click here to see how this site uses Cookies. View our privacy policy here.