DOI:
10.1039/D4SC05585C
(Edge Article)
Chem. Sci., 2024,
15, 18455-18462
A three-dimensional lead iodide perovskite analog featuring hydrogen-bonded dual monovalent cations†
Received
20th August 2024
, Accepted 11th October 2024
First published on 11th October 2024
Abstract
Three-dimensional (3D) halide perovskites have attracted considerable research interest, yet the selection of A-site cations is restricted by the Goldschmidt tolerance factor. To accommodate cations beyond this acceptable range, novel 3D perovskite analog structures with edge- and face-sharing motifs have been developed. Until now, these structures have been limited to divalent cations due to significant electrostatic repulsion when incorporating two monovalent cations. Herein, we employ a supramolecular synthon mechanism to address the issue and an effective hydrogen-bonding pattern is achieved in a novel 3D lead iodide hybrid perovskite, (ammonium)(morpholinium)Pb2I6 (1). The inorganic framework of 1 consists of two edge-shared [PbI6] octahedra connected via corner-sharing, thus forming a continuous 3D network. Structural analysis indicates that the spatial separation of N atoms and the existence of N–H⋯O hydrogen bonds effectively eliminate electrostatic repulsion. This work has demonstrated the potential to mitigate constraints of cation selection on 3D frameworks and could spur the development of novel 3D perovskite materials and related fields.
Introduction
Halide perovskites (HPs) have attracted significant attention due to their remarkable properties, including ferroelectricity,1,2 piezoelectricity,3,4 photoluminescence,5 and their applications in photodetection and photovoltaics.6–11 Among them, three-dimensional (3D) HPs are extensively studied for their high absorption coefficients,12 high defect tolerance,13 long carrier diffusion lengths,14–16 and cost-effective solution processing.17 These characteristics have accelerated advancements in technologies such as solar cells,18 light-emitting diodes,19 photodetectors,20 and lasers.21 Typically represented by the general formula AMX3 (Fig. 1a), where A is a monovalent cation, M is a divalent metal, and X is a halide, the phase stability of the 3D perovskites are influenced by the Goldschmidt tolerance factor t = (rA + rX)/[√2(rM + rX)], where rA, rM, and rX denote the effective ionic radii.22–24 Perovskite phases typically form effectively when 0.8 < t < 1, with deviations often resulting in non-perovskite structures. Consequently, the choice of A-site cations is restricted to small sizes. Currently, only a few monovalent cations, i.e., Cs+, CH3NH3+, CH3NH2NH2+, HC(NH2)2+ and aziridinium, have been identified as capable of maintaining the typical conner-shared 3D perovskite structure.25–29 Attempts to incorporate larger cations generally lead to lower-dimensional structures that hinder charge transport in at least one direction.30–32
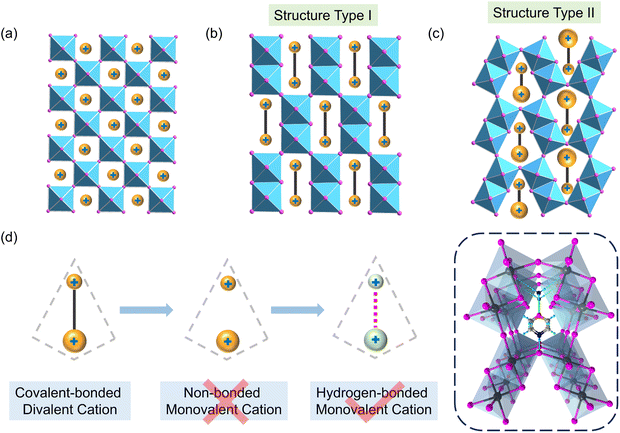 |
| Fig. 1 Octahedra connecting types of reported 3D perovskites: (a) classical AMX3; (b) type I; (c) type II. (d) Type II 3D perovskite analog with hydrogen-bonded monovalent cations reported in this work. | |
Recently, novel 3D perovskite analogs have emerged that can accommodate large diammonium cations.33–41 The fundamental building blocks consist of edge-shared [M2X10] units which further link through corner-sharing to form 3D frameworks capable of hosting divalent cations within either parallelepiped-shaped (Type I) or trigonal prism-shaped (Type II) voids (Fig. 1b and c). Synthetically, symmetric cations typically lead to type I voids39 while less symmetric ones result in type II.38,40 In essence, these 3D perovskite analogs are composed of edge-sharing octahedral dimers, with voids large enough to accommodate either two monovalent cations (A2M2X6) or one divalent cation (A′M2X6). The resulting chemical formulas, A2M2X6 or A′M2X6, effectively double the unit cell compared to traditional 3D perovskites. However, the reported structures are presently exclusively limited to A′M2X6 because the presence of significant electrostatic repulsion between two positively charged monocations within the void could make A2M2X6 energetically unfavorable and synthetically difficult. Considering the much higher abundance of monocations than dications, it is necessary to overcome this challenge to enrich the 3D perovskite family.
Herein, we employ a supramolecular synthon mechanism to address the issue caused by electrostatic repulsion of two positively charged monocations (Fig. 1d). Through carefully selecting the monocation pair, an effective hydrogen-bonding pattern is achieved between them to result in a pseudo-divalent cation and thus favor the formation of the type II framework. A synthesized model 3D perovskite analog (ammonium)(morpholinium)Pb2I6 (1) shows that the ammonium and morpholinium cations occupy the smaller and larger part of the void, respectively, based on their volumes. Structure analysis clarifies that the remote relative position of two positively charged N atoms and intermolecular N–H⋯O hydrogen bonding interaction helps to diminish the electrostatic repulsion. Compound 1 has an indirect bandgap, akin to the electronic band patterns found in AMX3 perovskites, featuring notably dispersive valence and conduction bands. And it also exhibits a good photoelectric response, thermal stability up to 250 °C and excellent long-term stability under conditions of 40% relative humidity. This work shows promise in overcoming limitations related to cation selection in 3D frameworks and may inspire the creation of new 3D perovskite materials.
Results and discussion
Synthesis
Compound 1 was synthesized using a solution-based method. In contrast to previously reported type I and type II 3D perovskite analogs, which require an excess of PbI2 to prevent the formation of other phases, 1 was synthesized with a ratio of NH4I
:
morpholine
:
PbI2 = 2
:
1
:
1. The solution was heated to over 110 °C and then gradually cooled to 90 °C, leading to the precipitation of red crystals within 12 hours (Fig. 2a). More details about crystal synthesis are provided in the ESI.† Phase purity of the sample was verified by powder X-ray diffraction (PXRD) by comparing experimental and simulated diffraction patterns (Fig. S1†).
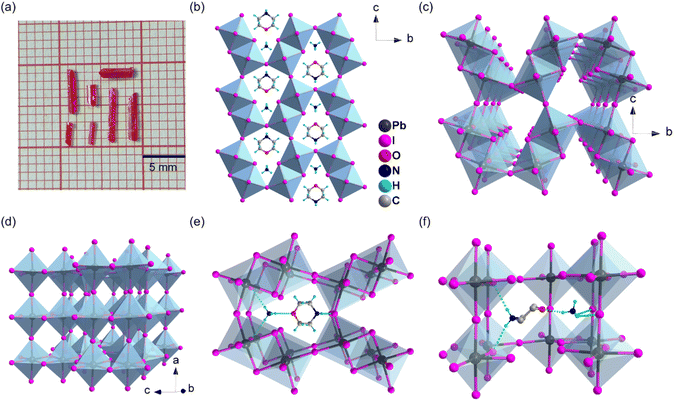 |
| Fig. 2 (a) Crystal image of 1. (b) basic structure motif of 1 viewed from a direction. (c and d) Inorganic framework showing 3D connectivity. Organic parts are omitted for clarity. (e and f) Hydrogen bonding interactions within and between the organic parts and inorganic framework. | |
Crystal structure analysis
Single-crystal diffraction analysis of 1 reveals a monoclinic structure within the P21/m space group, characterized by cell parameters of a = 6.3366(3) Å, b = 16.1613(7) Å, c = 10.1821(4) Å, α = 90°, β = 93.579(4)°, γ = 90°, and volume V = 1040.69(8) Å3 (Table S1†). The structure features inorganic dimers composed of two edge-sharing octahedra of adjacent Pb ions. Four dimers, connected via corner-sharing, constitute a trigonal prism-shaped void (Fig. 2b). Further corner-sharing of these dimers extends in bc plane to form a continuous inorganic layer and the layers are stacked along the a-axis by angle-to-angle connections to result in a 3D inorganic framework (Fig. 2c and d), as classified as type II 3D perovskite. This unique arrangement of the inorganic framework provides ample space to accommodate two monovalent cations, which are anchored to the halide ions of PbI6 octahedra through N–H⋯I hydrogen bonding interactions (Fig. 2e, f, Tables S2 and S3†). Specifically, within the trigonal prism-shaped void, the NH4+ and morpholinium cations occupy the smaller and larger volume sides, respectively, reflecting their significant volume disparity. Regarding the structure, positively charged N atoms of NH4 and morpholinium cations are strategically positioned on opposite sides of the void, thereby minimizing electrostatic repulsion. Meanwhile, the N–H⋯O hydrogen bonding interactions between the NH4+ and morpholinium cations further reduce electrostatic repulsion and enhance structural stability. This marks the first successful filling of a void in a 3D perovskite analog structure with mixed monovalent cations via intermolecular hydrogen bonds to overcome the electrostatic repulsion between positively charged species.
Given the flexibility of monocations, we aim to identify other cations that could potentially replace morpholinium to form similar 3D frameworks. We selected several cations for calculation based on their comparable volumes and shapes, as well as their potential to form hydrogen bonds with NH4+. According to Table S4,† our calculations suggest that thiomorpholinium, 4-hydroxypiperidinium, and tetrahydro-2H-pyran-4-aminium are promising candidates, with volume differences of less than 5%. However, experimental validation is further required to confirm these findings.
Because of the strong links to the electronic properties, it is important to analyze the connectivity types of the metal iodide octahedra in the structure as well as the M–X–M angles and bond distortions. To simplify the discussion, we measure the Pb–I–Pb angles by taking the two edge-shared octahedra as a whole basic unit and only consider the Pb–I–Pb angles of corner-sharing connectivity since they are the special feature in perovskites and most relevant to the charge transport and electronic band dispersion. As shown in Fig. 3, 1 has three different Pb–I–Pb angles. The largest one is 180°, which is slightly higher than that of (TMEA)Pb2Br6 (Fig. S2,† TMEA = N,N,N-trimethylethane-1,2-diaminium),35 indicating the structure is not tilted and very similar to type II structure. In the a axial direction where the octahedra are connected by corner-sharing, the Pb–I–Pb angles are 173.61°, closely to 180°. The smallest one is 146.16°, much smaller than 180°. The average Pb–I–Pb angle is calculated to be 166.59°, showing slightly smaller than that of (TMEA)Pb2Br6.
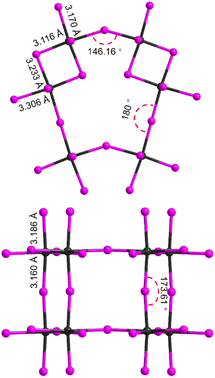 |
| Fig. 3 Summary of bond angles between inter-dimer Pb–I–Pb bonds and bond lengths within each octahedron. | |
We also assess the distortion levels of individual octahedra by using the distortion index (Δd) and bond angle variance (σ2), which are defined based on the variations in Pb–I bond lengths and Pb–I–Pb bond angles as shown in eqn (1) and (2):
| 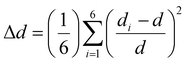 | (1) |
| 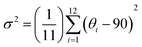 | (2) |
where
d and
di are the average and individual bond distance, respectively, and
θi is the individual angle. For
1, the two octahedra forming the dimer exhibit similar levels of distortion with Δ
d and
σ2 of 3.58 × 10
−4 and 12.48, respectively. The values are notably lower than those typically reported for 3D edge-shared HPs,
35,41 suggesting a minimal distortion close resemblance to an ideal structural model.
Electronic band structure
Density functional theory (DFT) calculations were conducted to analyze the electronic band structures and dimensional properties of 1. As depicted in Fig. 4a, the valence band maximum (VBM) appears at the A point while the conduction band minimum (CBM) lies at the Y2 point. The calculated band structure reveals an indirect feature with a bandgap of 1.86 eV, which exceeds that of corner-sharing MAPbI3 (1.5 eV) and is comparable to 3D lead iodide hybrid perovskite analogs with similar edge-sharing dimers (e.g., 1.93 eV of (M2pda)Pb2I6 (M2pda = N,N′-dimethylpropane-1,3-diaminium)).36 Compound 1 exhibits relatively flat valence bands and dispersive conduction bands. This is because the octahedra are connected in a corner-sharing mode along a direction while a combination of corner-sharing and edge-sharing is observed in the other two directions. Consequently, the Γ-Y2 direction in the Brillouin zone, corresponding to a direction in real space, exhibits the most energy dispersion for conduction bands. This contrasts sharply with other crystallographic directions where octahedra share edges, resulting in significantly less dispersive electronic bands. This is also evident from the calculated effective masses (Table S5†). The effective mass for both electrons and holes along a direction is significantly smaller than in the other two directions, indicating that charge transport is greatly restricted in the edge-sharing plane.35,41 These results align with our understanding that the corner-shared motif generally results in greater band dispersions compared to the edge-shared one, followed by the face-shared motif.42–44
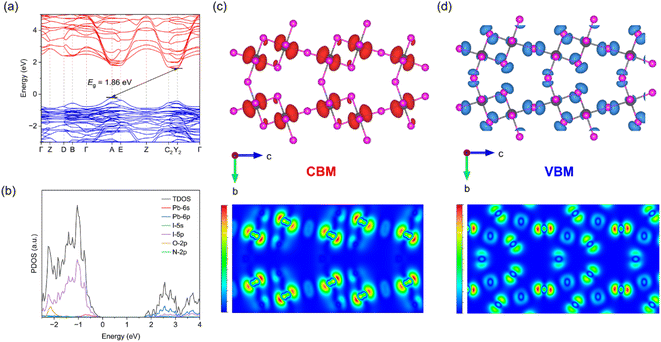 |
| Fig. 4 DFT calculations of the electronic structural features of 1: (a) band structures and (b) projected density of states (DOS); calculated partial charge densities (PCD) and 2D maps within the (100) plane for (c) VBM and (d) CBM. | |
Density of states data indicates that the VBM is formed by overlapping Pb-6s and I-5p orbitals while the CBM results from overlapping Pb-6p orbitals, highlighting that the energy band and semiconductor performance are predominantly governed by the inorganic components, with negligible contributions from organic cations (Fig. 4b). Additionally, partial charge density (PCD) distributions of the CBM and VBM were computed to deepen the understanding of the electronic properties. Analysis of the charge density associated with the VBM and CBM in the bc plane also reveals that the VBM is primarily contributed by Pb and I whereas Pb dominates in the CBM (Fig. 4c and d). Contour plots of the PCD for the CBM and VBM within the bc plane demonstrate directional differences along the b and c axes, indicating anisotropic electronic characteristics. Nevertheless, the orbitals contributing to the VBM and CBM maintain continuity across all three crystallographic axes (Fig. S3†). Owing to the 3D connectivity of the band edge-determining [PbI6] octahedra, 1 showcases a 3D electronic nature, which aligns with the structural connectivity of its inorganic framework.
Optical properties
Ultraviolet-visible diffuse-reflectance spectra of 1 were recorded and shown in Fig. 5a. There is significant absorption in the ultraviolet region. The edge of the band extends into the visible light region near 600 nm. Based on the Kubelka–Munk equation,45 the optical bandgap value is estimated to be 2.10 eV (Fig. 5a inset), which is slightly smaller than the bandgap of common 2D lead iodide perovskite semiconductors42 and is comparable to 3D lead iodide perovskite (M2pda)Pb2I6.36 A steady-state photoluminescence (PL) spectrum of the powder sample of compound 1 was recorded with 460 nm excitation. At room temperature, 1 exhibits weak emission, similar to many 3D lead perovskite analogs.35,41 The PL spectrum reveals a relatively narrow peak at 590 nm with a full width at half maximum (FWHM) of approximately 40 nm (Fig. S4a†). This contrasts with previously reported results for (NMPA)Pb2Br6 (NMPA = N,N′-dimethylpropane-1,3-diaminium) and (DMEA)Pb2Br6 (DMEA = N,N-dimethylethane-1,2-diaminium), which display broader emission.35 And the extracted PL lifetime by fitting the PL decay curves demonstrate a short lifetime of 0.74 ns (Fig. S4b†). Combining with the fact that the emission peak is near the band edge, the PL emission of 1 is likely to be excitonic. However, additional experimental evidence, such as temperature-dependent and power-dependent PL measurements, would be helpful.
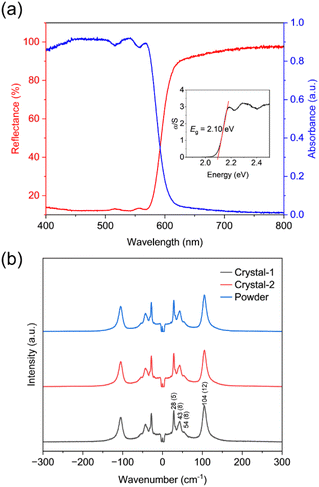 |
| Fig. 5 (a) UV-visible diffuse-reflectance spectra and (b) low-frequency Raman spectra of 1. The full-width at half-maximum (FWHM) indicated in parentheses. | |
Raman spectroscopy of 1 was performed at room temperature to explore the dynamic characteristics (Fig. 5b). It exhibits obvious peaks at 28, 43, and 104 cm−1, similar to those observed in conventional 3D AMX3 structures. Notably, peaks around 105 cm−1 are assigned to the symmetric and asymmetric stretching of [PbI6] octahedra while those below 85 cm−1 correspond to octahedral bending modes.41,46 The FWHM of these peaks are relatively narrow,47–49 indicating the absence of disorder at room temperature, which was in agreement with X-ray diffraction result. The Fourier transform infrared (FT-IR) spectroscopy was conducted to investigate the interaction between the organic molecules and the inorganic framework. The signals around 3570, 3500 and 3130 cm−1, attributed to N–H vibration of 1, shift to higher wavenumbers compared to that of the precursor cations, indicating the presence of weak hydrogen bonding interactions between the organic molecular cations and the [PbI6] octahedral frameworks (Fig. S5†). And these signals are sharp than the observed broad peak in NH4I precursor, which may indicate an order of NH4+ in the lattice. Theses observation aligns with the structural analysis described above.
Optoelectronic response
Based on the promising optical and electronic properties of 1, we conducted an initial evaluation of its photoresponse characteristics. Silver electrodes were utilized with the direction oriented along the a-axis, where all the octahedra are interconnected by corner-sharing. When subjected to ambient light at a wavelength of 565 nm, the device exhibited a distinct photoresponse, even under low power conditions of 1.6 μW cm−2 (Fig. S6a†). The on-off photoresponse increased with the power of the light, and at a light power of 62.9 μW cm−2, the device demonstrated a pronounced photoresponse with a photocurrent that was 10 times higher than the dark current. Furthermore, stability and durability tests under pulsed light revealed that the device maintained excellent photoelectric performance even after more than 103 cycles (Fig. S6b†). These findings indicate significant potential for photodetection applications at room temperature.
Structural phase transition and stability
In the context of known 3D perovskites or analogs, many exhibit structural phase transitions.33,36 However, in the case of 1, differential scanning calorimetry measurements indicate no phase transition within the measured temperature range (Fig. S7a†). The temperature-dependent dielectric measurements show no anomalies during heating and cooling, which aligns with the DSC results, further confirming that there is no structural phase transition (Fig. S7b†). Thermogravimetric analysis (TGA) evaluated the thermal stability of 1, demonstrating a good stability up to approximately 250 °C (Fig. S8†). This stability, while slightly lower than 3D perovskites containing dications, is attributed to the lighter NH4+ and weak N–H⋯O interactions. Furthermore, after exposure to 40% relative humidity for three months, the powders of 1 maintained a consistent PXRD pattern with fresh one, highlighting its good long-term stability (Fig. S9†).
Conclusions
In summary, we successfully synthesized a 3D perovskite analogue, (ammonium)(morpholinium)Pb2I6, by strategically replacing one dication with two hydrogen-bonded monocations in the voids. This innovative approach effectively mitigates issues stemming from electrostatic repulsion between two positively charged monocations. The fundamental 3D inorganic framework adopts a type II structure with minimal structural distortion, accommodating ammonium and morpholinium cations in regions of varying volume based on their respective sizes. Detailed structural analysis reveals that the spatial arrangement of the two positively charged nitrogen atoms and the N–H⋯O hydrogen bonding interactions play a crucial role in reducing electrostatic repulsion. Density functional theory calculations indicate that 1 exhibits an indirect bandgap, similar to the electronic band structures observed in AMX3 perovskites, with relatively dispersive valence and conduction bands. The synthesis of such a 3D perovskite holds promise for alleviating limitations in cation selection and has the potential to catalyze the developments of new 3D perovskite materials and related fields.
Data availability
The data supporting this article have been included as part of the ESI.†
Author contributions
Wei Wang: conceptualization, data curation, formal analysis, investigation, methodology, validation and writing original draft; Cheng-Dong Liu: visualization and formal analysis; Chang-Chun Fan: investigation; Wen Zhang: conceptualization, data curation, funding acquisition, resources, supervision, software and writing – review & editing.
Conflicts of interest
The authors declare no competing financial interest.
Acknowledgements
This work was financially supported by the National Natural Science Foundation of China (21991144 and 22475040). We thank the Big Data Computing Center of Southeast University for providing facility support for the calculations.
References
- C. C. Fan, X. B. Han, B. D. Liang, C. Shi, L. P. Miao, C. Y. Chai, C. D. Liu, Q. Ye and W. Zhang, Chiral Rashba Ferroelectrics for Circularly Polarized Light Detection, Adv. Mater., 2022, 34, e2204119 CrossRef.
- C. D. Liu, C. C. Fan, B. D. Liang, C. Y. Chai, C. Q. Jing, X. B. Han and W. Zhang, Spectrally Selective Polarization-Sensitive Photodetection Based on a 1D Lead-Free Hybrid Perovskite Ferroelectric, ACS Mater. Lett., 2023, 5, 1974–1981 CrossRef CAS.
- P. Chen, C. Cheng, X. Yang, T. T. Sha, X. Zou, F. Zhang, W. Jiang, Y. Xu, X. Cao, Y. M. You and Z. Luo, Wireless Deep Brain Stimulation by Ultrasound-Responsive Molecular Piezoelectric Nanogenerators, ACS Nano, 2023, 17, 25625–25637 CrossRef CAS.
- T. M. Guo, F. F. Gao, Y. J. Gong, Z. G. Li, F. Wei, W. Li and X. H. Bu, Chiral Two-Dimensional Hybrid Organic-Inorganic Perovskites for Piezoelectric Ultrasound Detection, J. Am. Chem. Soc., 2023, 145, 22475–22482 CrossRef CAS.
- W. Wang, C.-D. Liu, X.-B. Han, C.-Q. Jing, C.-Y. Chai, C.-C. Fan, M.-L. Jin, J.-M. Zhang and W. Zhang, Photoluminescence Switching and Non-Volatile Memory in Hybrid Metal-Halide Phase-Change Materials, ACS Mater. Lett., 2024, 6, 203–211 CrossRef CAS.
- J. Huang, Y. Yuan, Y. Shao and Y. Yan, Understanding the physical properties of hybrid perovskites for photovoltaic applications, Nat. Rev. Mater., 2017, 2, 19 Search PubMed.
- J. V. Passarelli, D. J. Fairfield, N. A. Sather, M. P. Hendricks, H. Sai, C. L. Stern and S. I. Stupp, Enhanced Out-of-Plane Conductivity and Photovoltaic Performance in n = 1 Layered Perovskites through Organic Cation Design, J. Am. Chem. Soc., 2018, 140, 7313–7323 CrossRef CAS.
- W. Wang, C. D. Liu, C. C. Fan, X. B. Fu, C. Q. Jing, M. L. Jin, Y. M. You and W. Zhang, Rational Design of 2D Metal Halide Perovskites with Low Congruent Melting Temperature and Large Melt-Processable Window, J. Am. Chem. Soc., 2024, 146, 9272–9284 CrossRef CAS.
- C. D. Liu, C. C. Fan, B. D. Liang and W. Zhang, A polar two-dimensional lead-free hybrid perovskite for self-powered polarization-sensitive photodetection, Inorg. Chem. Front., 2024, 11, 4611–4618 RSC.
- B.-D. Liang, C.-C. Fan, C.-D. Liu, T.-Y. Ju, C.-Y. Chai, X.-B. Han and W. Zhang, Large in-plane anisotropic 2D perovskites toward highly linear polarized light responses, Inorg. Chem. Front., 2023, 10, 5035–5043 RSC.
- C. D. Liu, C. C. Fan, B. D. Liang, W. Wang, M. L. Jin, C. Q. Jing, J. M. Zhang and W. Zhang, Quantum well thickness control of a hybrid perovskite to achieve a tunable anisotropic photoresponse, J. Mater. Chem. C, 2024, 12, 12734–12738 RSC.
- M. A. Green, Y. Jiang, A. M. Soufiani and A. Ho-Baillie, Optical Properties of Photovoltaic Organic–Inorganic Lead Halide Perovskites, J. Phys. Chem. Lett., 2015, 6, 4774–4785 CrossRef CAS.
- J. Kang and L.-W. Wang, High Defect Tolerance in Lead Halide Perovskite CsPbBr3, J. Phys. Chem. Lett., 2017, 8, 489–493 CrossRef CAS.
- S. D. Stranks, G. E. Eperon, G. Grancini, C. Menelaou, M. J. P. Alcocer, T. Leijtens, L. M. Herz, A. Petrozza and H. J. Snaith, Electron-Hole Diffusion Lengths Exceeding 1 Micrometer in an Organometal Trihalide Perovskite Absorber, Science, 2013, 342, 341–344 CrossRef CAS.
- D. Shi, V. Adinolfi, R. Comin, M. Yuan, E. Alarousu, A. Buin, Y. Chen, S. Hoogland, A. Rothenberger, K. Katsiev, Y. Losovyj, X. Zhang, P. A. Dowben, O. F. Mohammed, E. H. Sargent and O. M. Bakr, Low trap-state density and long carrier diffusion in organolead trihalide perovskite single crystals, Science, 2015, 347, 519–522 CrossRef CAS.
- G. Long, Y. Zhou, M. Zhang, R. Sabatini, A. Rasmita, L. Huang, G. Lakhwani and W. Gao, Theoretical Prediction of Chiral 3D Hybrid Organic-Inorganic Perovskites, Adv. Mater., 2019, 31, e1807628 CrossRef.
- Z. Saki, M. M. Byranvand, N. Taghavinia, M. Kedia and M. Saliba, Solution-processed perovskite thin-films: the journey from lab- to large-scale solar cells, Energy Environ. Sci., 2021, 14, 5690–5722 RSC.
- H. J. Zhang, Z. J. Shi, L. G. Hu, Y. Y. Tang, Z. Y. Qin, W. Q. Liao, Z. S. Wang, J. J. Qin, X. G. Li, H. L. Wang, M. Gusain, F. C. Liu, Y. Y. Pan, M. S. Xu, J. Wang, R. Liu, C. F. Zhang, R. G. Xiong, W. E. I. Sha and Y. Q. Zhan, Highly Efficient 1D/3D Ferroelectric Perovskite Solar Cell, Adv. Funct. Mater., 2021, 31, 2100205 CrossRef CAS.
- A. Fakharuddin, M. K. Gangishetty, M. Abdi-Jalebi, S.-H. Chin, A. R. bin Mohd Yusoff, D. N. Congreve, W. Tress, F. Deschler, M. Vasilopoulou and H. J. Bolink, Perovskite light-emitting diodes, Nat. Electron., 2022, 5, 203–216 CrossRef CAS.
- R. Dong, Y. Fang, J. Chae, J. Dai, Z. Xiao, Q. Dong, Y. Yuan, A. Centrone, X. C. Zeng and J. Huang, High-Gain and Low-Driving-Voltage Photodetectors Based on Organolead Triiodide Perovskites, Adv. Mater., 2015, 27, 1912–1918 CrossRef CAS.
- H. Zhu, Y. Fu, F. Meng, X. Wu, Z. Gong, Q. Ding, M. V. Gustafsson, M. T. Trinh, S. Jin and X. Y. Zhu, Lead halide perovskite nanowire lasers with low lasing thresholds and high quality factors, Nat. Mater., 2015, 14, 636–642 CrossRef CAS.
- G. Kieslich, S. Sun and A. K. Cheetham, An extended Tolerance Factor approach for organic–inorganic perovskites, Chem. Sci., 2015, 6, 3430–3433 RSC.
- G. Kieslich, S. Sun and A. K. Cheetham, Solid-state principles applied to organic–inorganic perovskites: new tricks for an old dog, Chem. Sci., 2014, 5, 4712–4715 RSC.
- V. M. Goldschmidt, Die Gesetze der Krystallochemie, Naturwissenschaften, 1926, 14, 477–485 CrossRef CAS.
- C. C. Stoumpos and M. G. Kanatzidis, The Renaissance of Halide Perovskites and Their Evolution as Emerging Semiconductors, Acc. Chem. Res., 2015, 48, 2791–2802 CrossRef CAS.
- M. a. Mączka, M. Ptak, A. Gągor, D. Stefańska, J. K. Zaręba and A. Sieradzki, Methylhydrazinium Lead Bromide: Noncentrosymmetric Three-Dimensional Perovskite with Exceptionally Large Framework Distortion and Green Photoluminescence, Chem. Mater., 2020, 32, 1667–1673 CrossRef.
- M. Mączka, A. Gagor, J. K. Zaręba, D. Stefanska, M. Drozd, S. Balciunas, M. Šimėnas, J. Banys and A. Sieradzki, Three-Dimensional Perovskite Methylhydrazinium Lead Chloride with Two Polar Phases and Unusual Second-Harmonic Generation Bistability above Room Temperature, Chem. Mater., 2020, 32, 4072–4082 CrossRef.
- M. Mączka, M. Ptak, A. Gągor, J. K. Zaręba, X. Liang, S. Balčiūnas, O. A. Semenikhin, O. I. Kucheriv, I. y. A. Gural’skiy, S. Shova, A. Walsh, J. r. Banys and M. Šimėnas, Phase Transitions, Dielectric Response, and Nonlinear Optical Properties of Aziridinium Lead Halide Perovskites, Chem. Mater., 2023, 35, 9725–9738 CrossRef.
- H. R. Petrosova, O. I. Kucheriv, S. Shova and I. A. Gural'skiy, Aziridinium cation templating 3D lead halide hybrid perovskites, Chem. Commun., 2022, 58, 5745–5748 RSC.
- B. Saparov and D. B. Mitzi, Organic-Inorganic Perovskites: Structural Versatility for Functional Materials Design, Chem. Rev., 2016, 116, 4558–4596 CrossRef CAS.
- C. Katan, N. Mercier and J. Even, Quantum and Dielectric Confinement Effects in Lower-Dimensional Hybrid Perovskite Semiconductors, Chem. Rev., 2019, 119, 3140–3192 CrossRef CAS.
- X. Li, J. M. Hoffman and M. G. Kanatzidis, The 2D Halide Perovskite Rulebook: How the Spacer Influences Everything from the Structure to Optoelectronic Device Efficiency, Chem. Rev., 2021, 121, 2230–2291 CrossRef CAS.
- C. C. Fan, C. D. Liu, B. D. Liang, T. Y. Ju, W. Wang, M. L. Jin, C. Y. Chai and W. Zhang, Chiral three-dimensional organic-inorganic lead iodide hybrid semiconductors, Chem. Sci., 2024, 15, 11374–11381 RSC.
- J. Bai, H. Wang, J. Ma, Y. Zhao, H. Lu, Y. Zhang, S. Gull, T. Qiao, W. Qin, Y. Chen, L. Jiang, G. Long and Y. Wu, Wafer-Scale Patterning Integration of Chiral 3D Perovskite Single Crystals toward High-Performance Full-Stokes Polarimeter, J. Am. Chem. Soc., 2024, 146, 18771–18780 CrossRef CAS.
- X. T. Li, M. Kepenekian, L. D. Li, H. Dong, C. C. Stoumpos, R. Seshadri, C. Katan, P. J. Guo, J. Even and M. G. Kanatzidis, Tolerance Factor for Stabilizing 3D Hybrid Halide Perovskitoids Using Linear Diammonium Cations, J. Am. Chem. Soc., 2022, 144, 3902–3912 CrossRef CAS.
- C. C. Fan, B. D. Liang, C. D. Liu, C. Y. Chai, X. B. Han and W. Zhang, Stable organic lead iodides with three-dimensional crystallographic and electronic structures showing high photoresponse, Inorg. Chem. Front., 2022, 9, 6404–6411 RSC.
- P. F. Fu, S. L. Hu, J. Tang and Z. W. Xiao, Material exploration via designing spatial arrangement of octahedral units: a case study of lead halide perovskites, Front. Optoelectron., 2021, 14, 252–259 CrossRef.
- H. Y. Zhang, X. J. Song, H. Cheng, Y. L. Zeng, Y. Zhang, P. F. Li, W. Q. Liao and R. G. Xiong, A Three-Dimensional Lead Halide Perovskite-Related Ferroelectric, J. Am. Chem. Soc., 2020, 142, 4604–4608 CrossRef CAS.
- D. Umeyama, L. Leppert, B. A. Connor, M. A. Manumpil, J. B. Neaton and H. I. Karunadasa, Expanded Analogs of Three-Dimensional Lead-Halide Hybrid Perovskites, Angew. Chem., Int. Ed., 2020, 59, 19087–19094 CrossRef CAS.
- Y. Y. Tang, Y. H. Liu, H. Peng, B. B. Deng, T. T. Cheng and Y. T. Hu, Three-Dimensional Lead Bromide Hybrid Ferroelectric Realized by Lattice Expansion, J. Am. Chem. Soc., 2020, 142, 19698–19704 CrossRef CAS.
- X. T. Li, Y. H. He, M. Kepenekian, P. J. Guo, W. J. Ke, J. Even, C. Katan, C. C. Stoumpos, R. D. Schaller and M. G. Kanatzidis, Three-Dimensional Lead Iodide Perovskiteoid Hybrids with High X-ray Photoresponse, J. Am. Chem. Soc., 2020, 142, 6625–6637 CrossRef CAS.
- W. Wang, C. D. Liu, C. C. Fan and W. Zhang, Reversible Glass-Crystal Transition in a New Type of 2D Metal Halide Perovskites, Adv. Funct. Mater., 2024, 2407143 CrossRef.
- J. M. Hoffman, X. Che, S. Sidhik, X. Li, I. Hadar, J. C. Blancon, H. Yamaguchi, M. Kepenekian, C. Katan, J. Even, C. C. Stoumpos, A. D. Mohite and M. G. Kanatzidis, From 2D to 1D Electronic Dimensionality in Halide Perovskites with Stepped and Flat Layers Using Propylammonium as a Spacer, J. Am. Chem. Soc., 2019, 141, 10661–10676 CrossRef CAS.
- L. Mao, P. Guo, M. Kepenekian, I. Hadar, C. Katan, J. Even, R. D. Schaller, C. C. Stoumpos and M. G. Kanatzidis, Structural Diversity in White-Light-Emitting Hybrid Lead Bromide Perovskites, J. Am. Chem. Soc., 2018, 140, 13078–13088 CrossRef CAS.
- T. Eickhoff, P. Grosse and W. Theiss, Diffuse reflectance spectroscopy of powders, Vib. Spectrosc., 1990, 1, 229–233 CrossRef.
- C. Quarti, G. Grancini, E. Mosconi, P. Bruno, J. M. Ball, M. M. Lee, H. J. Snaith, A. Petrozza and F. D. Angelis, The Raman Spectrum of the CH3NH3PbI3 Hybrid Perovskite: Interplay of Theory and Experiment, J. Phys. Chem. Lett., 2014, 5, 279–284 CrossRef CAS.
- M. Mączka, J. A. Zienkiewicz and M. Ptak, Comparative Studies of Phonon Properties of Three-Dimensional Hybrid Organic–Inorganic Perovskites Comprising Methylhydrazinium, Methylammonium, and Formamidinium Cations, J. Phys. Chem. C, 2022, 126, 4048–4056 CrossRef.
- M. Mączka, M. Ptak, K. Fedoruk, D. Stefańska, A. Gągor, J. K. Zaręba and A. Sieradzki, The lattice symmetrization worked, but with a plot twist: effects of methylhydrazinium doping of MAPbI3 on phase transitions, cation dynamics and photoluminescence, J. Mater. Chem. C, 2024, 12, 1396–1405 RSC.
- K. Nakada, Y. Matsumoto, Y. Shimoi, K. Yamada and Y. Furukawa, Temperature-Dependent Evolution of Raman Spectra of Methylammonium Lead Halide Perovskites, CH3NH3PbX3 (X = I, Br), Molecules, 2019, 24, 626 CrossRef.
Footnote |
† Electronic supplementary information (ESI) available: Experimental details, Fig. S1–S9 and Tables S1–S5. CCDC 2387432. For ESI and crystallographic data in CIF or other electronic format see DOI: https://doi.org/10.1039/d4sc05585c |
|
This journal is © The Royal Society of Chemistry 2024 |
Click here to see how this site uses Cookies. View our privacy policy here.