DOI:
10.1039/D3SD00297G
(Paper)
Sens. Diagn., 2024,
3, 295-300
Artificial light-harvesting nanoparticles based on a tripodal fluorescence sensor mediated by multiple luminescence mechanisms†
Received
1st November 2023
, Accepted 15th December 2023
First published on 22nd December 2023
Abstract
It is highly desirable to precisely control the luminescence properties of organic molecules through the combination of multiple fluorescence mechanisms. In this work, we designed and synthesized a tripodal sensor molecule G, which contains three tetraphenylethylene (TPE) groups and three Schiff base groups. The G molecules can form nanoparticles in H2O/THF mixed solvent (fw = 90%) through the reprecipitation method. Moreover, by co-precipitation with an energy acceptor dye NDI, efficient artificial light harvesting nanoparticles (G–NDI NPs) could be obtained. As a result, a combination of three fluorescence mechanisms was introduced into this binary system: excited-state intramolecular proton-transfer (ESIPT) based on Schiff base groups, aggregation-induced emission (AIE) based on TPE groups, and Förster resonance energy transfer (FRET) between the donor and acceptor. At a relatively high donor/acceptor ratio (200/1), the antenna effect can reach 11.4 and the energy transfer efficiency can reach 22.0%. Furthermore, these assembled NPs were successfully used in the detection of Fe3+ and Cu2+ ions and showed good probing abilities with large Stokes shifts and red fluorescence quenching phenomena. This study will further inspire the development of multi-color luminescent materials by controlling multiple mechanisms and show great potentials in chemical sensing, bioimaging, and photoluminescent devices.
Introduction
Natural photosynthesis is vital for all living things on the Earth. The initial process of photosynthesis is the harvesting of sunlight through a large number of antenna chromophores.1,2 The absorbed energy was stepwise transferred in chlorophyll and eventually reaches the reaction center. Inspired by nature, many artificial light-harvesting systems (LHSs)3–9 have been reported in recent years for different applications, such as photocatalysis,10–23 bio-imaging,24–26 information encryption27–29 and light-emitting devices.30–33 These systems are often nanoparticles (NPs) dispersed in aqueous media to meet the requirement of green production. Strategies for preparing such NPs generally include self-assembly,34–37 micro-emulsion,38–43 reprecipitation44,45etc. For example, Wang and co-workers constructed artificial light-harvesting NPs based on the self-assembly of water-soluble pillar[6]arene.46 Yang and co-workers pioneered the fabrication of light-harvesting NPs through a micro-emulsion method.47 Acharyya and co-workers reported light-harvesting NPs with cascade energy transfer built in 90% water-acetone solution.48 However, the organic donor fluorophores used in these NPs often lack further functionality, such as sensing capabilities, hindering their application in chemical/biosensing fields. Therefore, it is highly desirable to create functional light-harvesting NPs based on multi-functional donor molecules in an efficient manner for chemical sensing.
To enable the NPs to be highly emissive, aggregation-induce emission (AIE)49–53 fluorophores are reasonably chosen owing to their high luminescence efficiency in a confined micro-environment. Actually, light-harvesting NPs based on the AIE fluorophore have received considerable attention recently.8 To avoid self-absorption and achieve large Stokes shift in organic fluorescent molecules, the excited-state intramolecular proton-transfer (ESIPT) mechanism is often adopted.54–56 ESIPT is a process in which excited molecules with intramolecular hydrogen bonds relax their energy through tautomerization by proton transfer. The integration of AIE and ESIPT in one organic molecule can be realized straightforwardly by molecular structure modification, affording highly fluorescent NPs with a large Stokes shift. By introducing proper acceptor dyes into the NPs, Förster resonance energy transfer (FRET) could be further occured.57–59 Based on the idea of this AIE–ESIPT–FRET triple fluorescence mechanism, we previously reported a spirocyclic bridged double tetraphenylethylene (TPE) derivative to achieve the construction of a LHS and the recognition of metal ions.60
Considering the outstanding performance of tripodal molecules in chemical sensing,61–63 we designed and synthesized a tritopic monomer (G) based on our previous experience, which contains a triethylamine tripod with a TPE group at each end (Scheme 1). The tripod and TPE are connected by Schiff-base units. On this basis, strong fluorescent NPs with a large Stokes shift could be obtained by reprecipitation in aqueous media due to the AIE–ESIPT dual mechanism of G. In addition, by introducing acceptor (A) molecules (NDI) into nanoparticles to achieve FRET of G (donor, D), an artificial light harvesting system based on the ESIPT–AIE–FRET triple mechanism can be constructed and exhibit good antenna effects. Furthermore, due to the metal-coordination ability of Schiff-base groups, it is worth noting that both G and light-harvesting G–NDI nanoparticles exhibit good detection capabilities for Fe3+ and Cu2+.
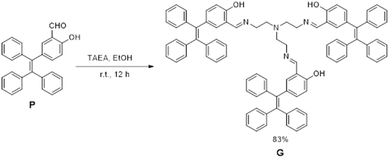 |
| Scheme 1 Synthesis of compound G. | |
Experimental
Materials and methods
Chemicals were purchased from commercial suppliers. Yields were given as isolated yields. 1H/13C NMR spectroscopy was performed on Bruker AVANCE III spectrometers (300 MHz/400 MHz). High-resolution electrospray ionization mass spectra (HR-ESI-MS) were recorded on an Agilent Technologies 6540 UHD Accurate-Mass. DLS were measured on a Zetasizer Nano ZS ZEN3600. TEM investigations were carried out on a JEM-2100 instrument. UV-vis spectra were carried out on a Perkin Elmer Lambda 35 UV-vis spectrometer. Fluorescence was performed on an Agilent Cary Eclipse spectrofluorometer. The fluorescence lifetimes were determined using time correlated single photon counting (TCSPC) on a FLS980 instrument with a pulsed xenon lamp. Analysis of fluorescence decay curves were subjected to fit a bi-exponential decay. The instrument response function (IRF) measures the scattering of laser excitation from non-fluorescent control samples to measure the fastest possible response of the detectors. The absolute fluorescence quantum yields were measured on a FLS980 instrument with an integrating sphere.
Synthesis of G
The imine group-containing compound G is simply synthesized by the condensation of an aldehyde group-containing the TPE derivative P60 and tris(2-aminoethyl)amine (TAEA) (Scheme 1). The final compound G was fully characterized by 1H/13C NMR and high-resolution ESI-MS (Fig. S15–S17, ESI†). To a 150 mL flask, P (1.00 g, 2.66 mmol), TAEA (0.13 g, 0.89 mmol) and anhydrous ethanol (70 mL) were added. The mixture was stirred at room temperature for 12 hours. After that, the obtained mixture was filtered and the solid was washed with ethanol thoroughly. The orange residue was further purified by recrystallization using dichloromethane and hexane to give the yellow solid product G (0.90 g, 83%). 1H NMR (300 MHz, CDCl3): δ (ppm) = 13.35 (s, 3H, Ar–OH), 7.87 (s, 3H, –CH
N–), 7.08–6.99 (m, 45H, Ar–H), 6.90 (d, J = 8.4 Hz, 3H, Ar–H), 6.83 (s, 3H, Ar–H), 6.59 (d, J = 8.7 Hz, 3H, Ar–H), 3.47(br s, 6H, –CH2–), 2.82 (br s, 6H, –CH2–). 13C NMR (100 MHz, CDCl3): δ (ppm) = 165.94, 160.15, 143.95, 143.91, 143.74, 140.43, 140.03, 135.71, 134.36, 134.20, 131.55, 131.44, 131.42, 127.98, 127.84, 127.77, 126.66, 126.52, 126.45, 118.11, 116.54, 58.58, 56.03. HR-ESI-MS: m/z calcd for C87H72N4O3 [M + H]+ = 1221.5677, found = 1221.5620.
Preparation of NP dispersion
Nanoparticles of G are prepared by a reprecipitation method in a mixed solvent of good and poor solvents. To a tetrahydrofuran (THF) solution of G (5.00 × 10−4 M, 0.4 mL), deionized H2O (3.6 mL) was added. The test concentration of G was kept at 50 μM. After ultrasonication at room temperature for 10 min, stable and dispersed G NPs in aqueous media were obtained. The nanoparticles of G–NDI with different D/A ratios were prepared similarly by a co-reprecipitation method.
Chemical sensing towards Fe3+ and Cu2+
Metal cation solutions (5.00 × 10−4 M) were prepared in H2O. Nitrate anion was used as the counter anion for all metal salts. 0.4 mL of G (5.00 × 10−4 M) in THF and 0.4 mL of metal cation solution were transferred into a 4 mL volumetric flask using a pipette, and then 3.2 mL of H2O was added (fw = 90%, [G] = 5.00 × 10−5 M, [Mn+] = 5.00 × 10−5 M). The test solution was ultrasonicated for 10 min. Afterwards, fluorescence testing was performed. Sensing experiments using G–NDI as a probe were carried out in a similar manner.
Results and discussion
Photophysical properties of G
The absorption and emission properties of G were first studied (Fig. S1, ESI†). The UV-vis absorption spectrum of G in mixed THF/H2O (v/v = 1/9) solvent indicates the absorption maximum is at 325 nm. Interestingly, the fluorescence spectrum of G shows a dual emission with a weak peak at 389 nm and an intensified peak at 550 nm, which is due to the ESIPT mechanism. The weak peak at 389 nm was ascribed to the enol form of G (Scheme S1, ESI†). However, the excited G would undergo ESIPT and transform to the ketone form, resulting in a large red-shift emission at 550 nm. In the meantime, the Stokes shift of G was increased from 64 nm to 225 nm, which greatly avoids the self-absorption of the material.
Besides ESIPT, the AIE mechanism of G was further investigated. THF is a good solvent for G, so G exhibits a very weak emission in pure THF (Fig. 1a and c). Upon the addition of poor solvent H2O into the solution, G's fluorescence was remarkably increased, especially for samples with a H2O fraction (fw, v/v) more than 70%. The increasing trend of fluorescence intensity with fw is shown in Fig. 1b. This should be attributed to the restriction of intramolecular movement of TPEs in G, which greatly reduced the non-radiation pathway. Although G samples with fw > 90% exhibit a brighter fluorescence, it's not stable under this condition. Therefore, this work selects fw = 90% as the condition to conduct all tests. The good ESIPT–AIE dual fluorescence mechanism of G provides a perfect prerequisite for the construction of light harvesting systems.
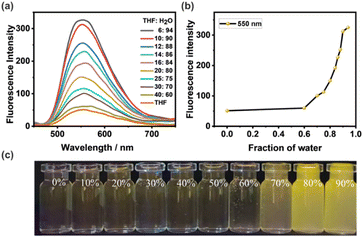 |
| Fig. 1 (a) Fluorescence spectra of compound G (5.00 × 10−5 M) in THF/H2O mixtures with different H2O fractions, λex = 325 nm. (b) Plot of fluorescence intensity of compound G at 550 nm versus H2O fractions. (c) Photographs of G in THF/H2O mixed solvent under UV irradiation at 365 nm. | |
Morphological characterization
The morphology of the reprecipitated NPs of G was studied by dynamic light scattering (DLS), transmission electron microscopy (TEM) and the Tyndall effect. The DLS data of G showed an average hydrodynamic diameter of 263 nm (Fig. 2a), while the TEM image exhibited a regular spherical shape (Fig. 2c). The sample also shows a clear Tyndall effect, further confirming the existence of abundant NPs in aqueous solution (Fig. 2a, inset). Since G showed remarkable AIE emission in aqueous media, it should be a suitable candidate as the donor to fabricate man-made light harvesting NPs. The hydrophobic dye NDI was used as the acceptor due to the good overlap between the absorption of NDI and the emission of G (Fig. 3a). Notably, G has no absorption after 450 nm, ensuring the feasibility of efficient FRET. Since the tripodal structure of G will provide many hydrophobic microenvironmental domains in NPs, hydrophobic NDI can be easily trapped into NPs through co-reprecipitation. The NDI-loaded NPs were further characterized by DLS, TEM and the Tyndall effect (Fig. 2b and d), also indicative of a well-defined spherical morphology with an average hydrodynamic diameter of 279 nm.
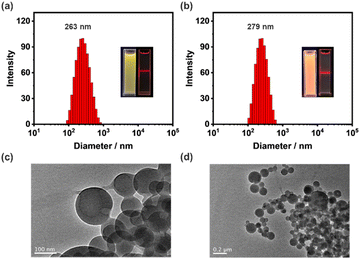 |
| Fig. 2 DLS data of (a) G NPs and (b) G–NDI NPs. Insets: fluorescence photographs (left) of each sample and their corresponding Tyndall effects (right). TEM images of (c) G NPs and (d) G–NDI NPs. [G] = 5.00 × 10−5 M, [NDI] = 2.50 × 10−7 M, respectively. | |
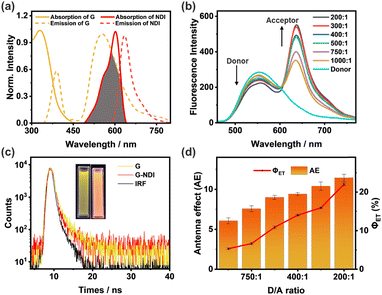 |
| Fig. 3 (a) Normalized absorbance spectra (solid curves) of G (yellow trace) and NDI (red trace), and their normalized fluorescence spectra (dashed curves). (b) Fluorescence spectra of G in the presence of different concentrations of NDI ([G] = 5.00 × 10−5 M, λex = 325 nm). (c) Fluorescence decay profiles of G and G–NDI, inset: fluorescence images of G (left) and G–NDI (right), [G] = 5.00 × 10−5 M, [NDI] = 2.50 × 10−7 M. (d) ΦET and AE at different G/NDI ratios. | |
Construction of an artificial light-harvesting system
With the NDI-loaded nanoparticles in hand, the energy transfer process was thoroughly studied. As shown in Fig. 3b, when NDI was gradually titrated into the NPs, the emission of G (donor, D) at 550 nm decreased obviously when excited at 325 nm. In the meantime, the emission of NDI (acceptor, A) at 640 nm increased significantly. Furthermore, the fluorescence color changed from yellow to red (Fig. 3c, inset). These observations indicate that FRET indeed occurs from G to the encapsulated NDI molecule. The absolute fluorescence quantum yield increased from 2.76% to 5.64%, further indicating the efficient energy transfer process (Fig. S3, ESI†). In addition, this energy transfer process was further evidenced by the significant decrease in fluorescence lifetime (τ) of G upon the encapsulation of NDI (Fig. 3c). These fluorescence decay profiles were subjected to double exponential decay analysis. The fluorescence lifetimes of G determined at 550 nm are τ1 = 0.76 ns and τ2 = 3.55 ns (Table S1, ESI†). After co-assembly with 0.5% NDI, the fluorescence lifetimes of G decreased to τ1 = 0.52 ns and τ2 = 2.67 ns, suggesting the successful fabrication of an artificial light harvesting system.
In order to quantitatively evaluate the efficiency of these light harvesting NPs with different D/A ratios, the energy transfer efficiency (ΦET) and antenna effect (AE) were calculated according to the literature method.18 Herein, ΦET is the ratio of the energy absorbed by NDI to the total excitation energy of G. The AE is the fluorescence amplification of NDI in the G–NDI dual-component NPs, compared to direct excitation itself. As shown in Fig. 3d, both ΦET and AE increase with the increase of NDI. When G/NDI reaches 200/1, the ΦET was calculated to be 22.0% (Fig. S4 and Table S3, ESI†), and the AE was 11.4 (Fig. S5 and Table S4, ESI†), indicating that the obtained G–NDI NPs played a good role as light-harvesting systems in aqueous media.
Fluorescence sensing for Fe3+ and Cu2+
Since the Schiff base group has the ability to bind metal ions,64,65 we tried to use G nanoparticles and G–NDI binary nanoparticles to construct a two-color mode probe for sensing metal ions. Firstly, we investigated the fluorescence spectra of G NPs upon the addition of different cations (including Li+, Na+, K+, Cs+, Mg2+, Ba2+, Ca2+, Fe3+, Cu2+, Al3+, Cd2+, Hg2+, Zn2+, NH4+, and Ag+, [Mn+] = 5.00 × 10−5 M) in a mixed THF/H2O solvent (fw = 90%). As shown in Fig. 4a, the fluorescence of G was quenched in the presence of Fe3+ or Cu2+, while other cations had no significant effect on the fluorescence of G. Subsequently, the G–NDI system was further used to probe Fe3+ or Cu2+ with red emission quenching (Fig. 4b). Because Fe3+ or Cu2+ can quench the emission of G, the FRET processes between G and NDI were also interrupted. The fluorescence quenching effects of both G and G–NDI could also be directly observed from the fluorescence photo changes under UV irradiation (Fig. 4c). The G NPs displayed a yellow fluorescence quenching, and the G–NDI NPs showed a red fluorescence quenching. Therefore, fluorescence detectors based on dual-color mode for both Fe3+ and Cu2+ were obtained.
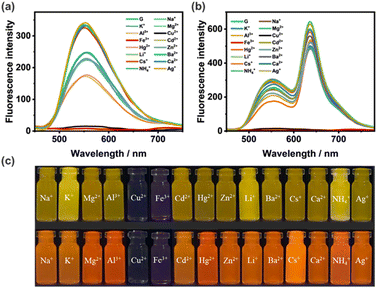 |
| Fig. 4 (a) Fluorescence spectra of G NPs (5.00 × 10−5 M, fw = 90%) interacting with different metal ions. (b) Fluorescence spectra of G–NDI NPs ([G] = 5.00 × 10−5 M, G/NDI = 200/1, fw = 90%) interacting with different metal ions. [Mn+] = 5.00 × 10−5 M, λex = 325 nm. (c) Photograph of compound G (upper) and G–NDI (down) interacting with metal ions under a 365 nm UV lamp. | |
It is worth noting that both iron or copper play important biological roles in the human body.66–69 For example, iron is involved in many metabolic processes and copper is crucial for the normal operation of the immune system. However, excessive iron or copper intake is harmful to human health. Therefore, monitoring iron or copper ions in the water environment is significantly important. Subsequently, more detailed testing on probing Fe3+ and Cu2+ was carried out. The binding behaviors of G with Fe3+ and Cu2+ was further evidenced by 1H NMR (Fig. S6 and S7, ESI†). Upon the titration of Fe3+ or Cu2+, the proton signal (Ha) from the hydroxyl group of G gradually disappeared, indicative of the coordination between metal and oxygen atoms. Notably, for Fe3+ and Cu2+ sensing, there is a dramatic difference in color change under natural light: Fe3+caused a light red change, while Cu2+ showed no obvious color change (Fig. S8, ESI†). By selecting cations and anions as potential interfering coexisting ions, anti-interference experiments were conducted, indicating that G exhibits high selectivity and specific recognition for Fe3+ and Cu2+ ions (Fig. S9 and S10, ESI†). In the titration experiments of Fe3+ or Cu2+, the fluorescence intensity of G gradually decreased at 550 nm, which showed a good linear relationship with the concentration of Fe3+ or Cu2+ (Fig. S11 and S12, ESI†). In addition, the fluorescence limit of detection (LOD) of G were calculated as 4.35 × 10−7 M for Fe3+ and 5.10 × 10−7 M for Cu2+, which are comparable to those reported in the literature.70–73 Furthermore, the Job's plot for G with Fe3+ and Cu2+ were depicted. The Job's plot of G and Cu2+ showed a stoichiometry of 1
:
1 (Fig. S13, ESI†). The Job's plot of G and Fe3+ showed a stoichiometry of 2
:
1 (Fig. S14, ESI†). Therefore, possible binding models were proposed as shown in Fig. S13c and S14c (ESI†).
In order to get further insight and evaluate the potential application of the probe G for detecting Fe3+ or Cu2+ in environmental water, water samples were collected from tap water, river water, and tea water, which were filtered for further testing. For either Cu2+ or Fe3+, the fluorescence intensity of the probe G showed a significant fluorescence quenching at 550 nm. The recoveries were calculated in the range of 93–103% in tap water, 100–110% in river water, and 91–99% in tea water, for Cu2+ (Table S5†). The recoveries were calculated in the range 97–108% in tap water, 92–106% in river water, and 99–109% in tea water, for Fe3+ (Table S6†). Therefore, the high selectivity and specificity of the probe G indicates that it could be used for monitoring Fe3+ or Cu2+ in a water environment.
Conclusions
In summary, we designed and synthesized a tripodal sensor G, which contains both TPE groups and Schiff base groups, ensuring the ESIPT and AIE mechanisms during the luminescence process. The G molecules can form nanoparticles in THF/H2O mixed solvent (fw = 90%) through the reprecipitation method. The nanoparticles were thoroughly characterized by the Tyndall effect, DLS, and TEM. By co-precipitation with an acceptor dye NDI, efficient artificial light harvesting nanoparticles could be prepared. At a high donor/acceptor ratio (D/A = 200/1), the antenna effect can reach 11.4 and the energy transfer efficiency can reach 22.0%. Moreover, both G nanoparticles and G–NDI light-harvesting nanoparticles show good Fe3+ and Cu2+ sensing abilities with dual-color fluorescence quenching phenomena. This work demonstrates a simple and green approach for constructing artificial light-harvesting system with ion-sensing ability, which may have great potential in the field of chemical probing and bioimaging.
Author contributions
Zhiying Wu: investigation, methodology, data curation, validation and writing – original draft. Qiaona Zhang: data curation and investigation. Dengli Chen: investigation. Tangxin Xiao: conceptualization, writing – original draft, preparation, project administration and supervision.
Conflicts of interest
The authors declare that they have no competing interests.
Acknowledgements
The authors are grateful for the financial support from the National Natural Science Foundation of China (21702020). D. C. acknowledges the Postgraduate Research & Practice Innovation Program of Jiangsu Province (grant no. KYCX23_3109).
References
- R. J. Sension, Nature, 2007, 446, 740–741 CrossRef CAS PubMed.
- D. Gust and T. A. Moore, Science, 1989, 244, 35–41 CrossRef CAS PubMed.
- Z. Wu, H. Qian, X. Li, T. Xiao and L. Wang, Chin. Chem. Lett., 2024, 35, 108829 CrossRef CAS.
- X. M. Chen, X. Chen, X. F. Hou, S. Zhang, D. Chen and Q. Li, Nanoscale Adv., 2023, 5, 1830–1852 RSC.
- Y. Han, X. Zhang, Z. Ge, Z. Gao, R. Liao and F. Wang, Nat. Commun., 2022, 13, 3546 CrossRef CAS PubMed.
- X. H. Wang, X. Y. Lou, T. Lu, C. Wang, J. Tang, F. Liu, Y. Wang and Y. W. Yang, ACS Appl. Mater. Interfaces, 2021, 13, 4593–4604 CrossRef CAS PubMed.
- K. Wang, K. Velmurugan, B. Li and X. Y. Hu, Chem. Commun., 2021, 57, 13641–13654 RSC.
- Y.-X. Hu, W.-J. Li, P.-P. Jia, X.-Q. Wang, L. Xu and H.-B. Yang, Adv. Opt. Mater., 2020, 8, 2000265 CrossRef CAS.
- J. Otsuki, J. Mater. Chem. A, 2018, 6, 6710–6753 RSC.
- T. Xiao, D. Chen, H. Qian, Y. Shen, L. Zhang, Z.-Y. Li and X.-Q. Sun, Dyes Pigm., 2023, 210, 110958 CrossRef CAS.
- Y. Wang, C. Q. Ma, X. L. Li, R. Z. Dong, H. Liu, R. Z. Wang, S. S. Yu and L. B. Xing, J. Mater. Chem. A, 2023, 11, 2627–2633 RSC.
- K. Wang, Y. Shen, P. Jeyakkumar, Y. Zhang, L. Chu, R. Zhang and X.-Y. Hu, Curr. Opin. Green Sustainable Chem., 2023, 41, 100823 CrossRef CAS.
- G. Sun, M. Li, L. Cai, D. Wang, Y. Cui, Y. Hu, T. Sun, J. Zhu and Y. Tang, J. Colloid Interface Sci., 2023, 641, 803–811 CrossRef CAS PubMed.
- Y. Qin, Q.-H. Ling, Y.-T. Wang, Y.-X. Hu, L. Hu, X. Zhao, D. Wang, H.-B. Yang, L. Xu and B. Z. Tang, Angew. Chem., Int. Ed., 2023, 62, e202308210 CrossRef CAS PubMed.
- J. Yu, H. Wang and Y. Liu, Adv. Opt. Mater., 2022, 10, 2201761 CrossRef CAS.
- Y. Li, C. Xia, R. Tian, L. Zhao, J. Hou, J. Wang, Q. Luo, J. Xu, L. Wang, C. Hou, B. Yang, H. Sun and J. Liu, ACS Nano, 2022, 16, 8012–8021 CrossRef CAS PubMed.
- D. Zhang, W. Yu, S. Li, Y. Xia, X. Li, Y. Li and T. Yi, J. Am. Chem. Soc., 2021, 143, 1313–1317 CrossRef CAS PubMed.
- M. Hao, G. Sun, M. Zuo, Z. Xu, Y. Chen, X. Y. Hu and L. Wang, Angew. Chem., Int. Ed., 2020, 59, 10095–10100 CrossRef CAS PubMed.
- Z. Zhang, Z. Zhao, Y. Hou, H. Wang, X. Li, G. He and M. Zhang, Angew. Chem., Int. Ed., 2019, 58, 8862–8866 CrossRef CAS PubMed.
- Q. Liu, M. Zuo, K. Wang and X.-Y. Hu, Chem. Commun., 2023, 59, 13707–13710 RSC.
- P. Jia, Y. Hu, Z. Zeng, Y. Wang, B. Song, Y. Jiang, H. Sun, M. Wang, W. Qiu and L. Xu, Chin. Chem. Lett., 2023, 34, 107511 CrossRef CAS.
- K. Wang, X. Tian, J. H. Jordan, K. Velmurugan, L. Wang and X.-Y. Hu, Chin. Chem. Lett., 2022, 33, 89–96 CrossRef CAS.
- G. Sun, M. Zuo, W. Qian, J. Jiao, X.-Y. Hu and L. Wang, Green Synth. Catal., 2021, 2, 32–37 CrossRef.
- G. Sun, L. Cai, Y. Zhang, Y. Hu, J. Zhu, T. Sun and Y. Tang, Dyes Pigm., 2022, 205, 110577 CrossRef CAS.
- M. Huo, X. Y. Dai and Y. Liu, Adv. Sci., 2022, 9, e2201523 CrossRef PubMed.
- X. M. Chen, Q. Cao, H. K. Bisoyi, M. Wang, H. Yang and Q. Li, Angew. Chem., Int. Ed., 2020, 59, 10493–10497 CrossRef CAS PubMed.
- T. Xiao, L. Zhang, D. Chen, Q. Zhang, Q. Wang, Z.-Y. Li and X.-Q. Sun, Org. Chem. Front., 2023, 10, 3245–3251 RSC.
- K. Diao, D. J. Whitaker, Z. Huang, H. Qian, D. Ren, L. Zhang, Z.-Y. Li, X.-Q. Sun, T. Xiao and L. Wang, Chem. Commun., 2022, 58, 2343–2346 RSC.
- T. Xiao, X. Wei, H. Wu, K. Diao, Z.-Y. Li and X.-Q. Sun, Dyes Pigm., 2021, 188, 109161 CrossRef CAS.
- T. Xiao, H. Qian, X. Li, Z. Wu, Z.-Y. Li and X.-Q. Sun, Dyes Pigm., 2023, 215, 111289 CrossRef CAS.
- H. Qian, T. Xiao, R. B. P. Elmes and L. Wang, Chin. Chem. Lett., 2023, 34, 108185 CrossRef CAS.
- Z. Lian, J. He, L. Liu, Y. Fan, X. Chen and H. Jiang, Nat. Commun., 2023, 14, 2752 CrossRef CAS PubMed.
- Q. Song, S. Goia, J. Yang, S. C. L. Hall, M. Staniforth, V. G. Stavros and S. Perrier, J. Am. Chem. Soc., 2021, 143, 382–389 CrossRef CAS PubMed.
- T. Xiao, L. Tang, D. Ren, K. Diao, Z.-Y. Li and X.-Q. Sun, Chem. – Eur. J., 2023, 29, e202203463 CrossRef CAS PubMed.
- X. Li, Z. Wu, Q. Wang, Z.-Y. Li, X.-Q. Sun and T. Xiao, ChemPlusChem, 2023, 88, e202300431 CrossRef CAS PubMed.
- T. Xiao, D. Ren, K. Diao, J. Wang, Z. Y. Li, X. Q. Sun and L. Wang, Chem. – Asian J., 2022, 17, e202200386 CrossRef CAS PubMed.
- T. Xiao, H. Qian, Y. Shen, C. Wei, D. Ren, L. Zhang, Z. Y. Li, L. Wang and X. Q. Sun, Mater. Today Chem., 2022, 24, 100833 CrossRef CAS.
- D. Ren, L. Tang, Z. Wu, Q. Zhang, T. Xiao, R. B. P. Elmes and L. Wang, Chin. Chem. Lett., 2023, 34, 108617 CrossRef CAS.
- T. Xiao, L. Zhang, H. Wu, H. Qian, D. Ren, Z.-Y. Li and X.-Q. Sun, Chem. Commun., 2021, 57, 5782–5785 RSC.
- T. Xiao, Y. Shen, C. Bao, K. Diao, D. Ren, H. Qian and L. Zhang, RSC Adv., 2021, 11, 30041–30045 RSC.
- T. Xiao, H. Wu, G. Sun, K. Diao, X. Wei, Z.-Y. Li, X.-Q. Sun and L. Wang, Chem. Commun., 2020, 56, 12021–12024 RSC.
- Y. Zhu, L. Xu, L. Wang, H. Tang and D. Cao, Chem. Commun., 2019, 55, 5910–5913 RSC.
- C. L. Sun, H. Q. Peng, L. Y. Niu, Y. Z. Chen, L. Z. Wu, C. H. Tung and Q. Z. Yang, Chem. Commun., 2018, 54, 1117–1120 RSC.
- B.-K. An, S.-K. Kwon, S.-D. Jung and S. Y. Park, J. Am. Chem. Soc., 2002, 124, 14410–14415 CrossRef CAS PubMed.
- H.-B. Fu and J.-N. Yao, J. Am. Chem. Soc., 2001, 123, 1434–1439 CrossRef CAS.
- S. Guo, Y. Song, Y. He, X. Y. Hu and L. Wang, Angew. Chem., Int. Ed., 2018, 57, 3163–3167 CrossRef CAS PubMed.
- H. Q. Peng, J. F. Xu, Y. Z. Chen, L. Z. Wu, C. H. Tung and Q. Z. Yang, Chem. Commun., 2014, 50, 1334–1337 RSC.
- K. Acharyya, S. Bhattacharyya, H. Sepehrpour, S. Chakraborty, S. Lu, B. Shi, X. Li, P. S. Mukherjee and P. J. Stang, J. Am. Chem. Soc., 2019, 141, 14565–14569 CrossRef CAS PubMed.
- M. H. Chua, H. Zhou, Q. Zhu, B. Z. Tang and J. W. Xu, Mater. Chem. Front., 2021, 5, 659–708 RSC.
- Z. Zhao, H. Zhang, J. W. Y. Lam and B. Z. Tang, Angew. Chem., Int. Ed., 2020, 59, 9888–9907 CrossRef CAS PubMed.
- F. Würthner, Angew. Chem., Int. Ed., 2020, 59, 14192–14196 CrossRef PubMed.
- Y. Hong, J. W. Y. Lam and B. Z. Tang, Chem. Soc. Rev., 2011, 40, 5361–5388 RSC.
- J. Luo, Z. Xie, J. W. Y. Lam, L. Cheng, H. Chen, C. Qiu, H. S. Kwok, X. Zhan, Y. Liu, D. Zhu and B. Z. Tang, Chem. Commun., 2001, 1740–1741 RSC.
- A. C. Sedgwick, L. Wu, H.-H. Han, S. D. Bull, X.-P. He, T. D. James, J. L. Sessler, B. Z. Tang, H. Tian and J. Yoon, Chem. Soc. Rev., 2018, 47, 8842–8880 RSC.
- V. S. Padalkar and S. Seki, Chem. Soc. Rev., 2016, 45, 169–202 RSC.
- J. Wu, W. Liu, J. Ge, H. Zhang and P. Wang, Chem. Soc. Rev., 2011, 40, 3483–3495 RSC.
- L. Xu, Z. Wang, R. Wang, L. Wang, X. He, H. Jiang, H. Tang, D. Cao and B. Z. Tang, Angew. Chem., Int. Ed., 2020, 59, 9908–9913 CrossRef CAS PubMed.
- T. Xiao, W. Zhong, L. Zhou, L. Xu, X.-Q. Sun, R. B. P. Elmes, X.-Y. Hu and L. Wang, Chin. Chem. Lett., 2019, 30, 31–36 CrossRef CAS.
- Z. Xu, S. Peng, Y. Y. Wang, J. K. Zhang, I. Lazar Alexandra and D. S. Guo, Adv. Mater., 2016, 28, 7666–7671 CrossRef CAS PubMed.
- T. Xiao, C. Bao, L. Zhang, K. Diao, D. Ren, C. Wei, Z.-Y. Li and X.-Q. Sun, J. Mater. Chem. A, 2022, 10, 8528–8534 RSC.
- J. Samanta, M. Tang, M. Zhang, R. P. Hughes, R. J. Staples and C. Ke, J. Am. Chem. Soc., 2023, 145, 21723–21728 CrossRef CAS PubMed.
- Q. Lin, X.-W. Guan, Y.-Q. Fan, J. Wang, L. Liu, J. Liu, H. Yao, Y.-M. Zhang and T.-B. Wei, New J. Chem., 2019, 43, 2030–2036 RSC.
- B. Kuswandi, Nuriman, W. Verboom and D. N. Reinhoudt, Sensors, 2006, 6, 978–1017 CrossRef CAS.
- H. Sun, Y. Jiang, J. Nie, J. Wei, B. Miao, Y. Zhao, L. Zhang and Z. Ni, Mater. Chem. Front., 2021, 5, 347–354 RSC.
- H.-F. Xie, C.-J. Yu, Y.-L. Huang, H. Xu, Q.-L. Zhang, X.-H. Sun, X. Feng and C. Redshaw, Mater. Chem. Front., 2020, 4, 1500–1506 RSC.
- Y. Sun, P. Sun and W. Guo, Coord. Chem. Rev., 2021, 429, 213645 CrossRef CAS.
- H. J. Lee, K. J. Korshavn, A. Kochi, J. S. Derrick and M. H. Lim, Chem. Soc. Rev., 2014, 43, 6672–6682 RSC.
- T. Kobayashi and N. K. Nishizawa, Plant Sci., 2014, 224, 36–43 CrossRef CAS PubMed.
- E. Gaggelli, H. Kozlowski, D. Valensin and G. Valensin, Chem. Rev., 2006, 106, 1995–2044 CrossRef CAS PubMed.
- X. Zhu, Y. Duan, P. Li, H. Fan, T. Han and X. Huang, Anal. Methods, 2019, 11, 642–647 RSC.
- A. Gümrükçüoğlu, Y. Topaloğlu, A. Mermer, N. Demirbaş, A. Demirbaş, M. Ocak and Ü. Ocak, J. Fluoresc., 2019, 29, 921–931 CrossRef PubMed.
- J. Tang, S. Ma, D. Zhang, Y. Liu, Y. Zhao and Y. Ye, Sens. Actuators, B, 2016, 236, 109–115 CrossRef CAS.
- G. J. Park, G. R. You, Y. W. Choi and C. Kim, Sens. Actuators, B, 2016, 229, 257–271 CrossRef CAS.
|
This journal is © The Royal Society of Chemistry 2024 |
Click here to see how this site uses Cookies. View our privacy policy here.