DOI:
10.1039/D3SU00368J
(Perspective)
RSC Sustainability, 2024,
2, 1337-1349
The pathway to net zero: a chemicals perspective
Received
14th October 2023
, Accepted 18th March 2024
First published on 22nd March 2024
Abstract
The global ambition is to reach a net zero waste and emissions society by 2050. For the chemical sector to align with these ambitions, it needs to find alternative resource flows for organic chemicals and derived materials to continue to contribute to the global economy. The current linear approach of the chemical industry is incompatible with emissions reduction targets, social responsibility and sustainable growth policies, a circular economy is viewed as a solution to facilitate the transition towards a net zero society. This paper sets out the current state of play for emissions and waste management from the chemical industry in the EU and UK, a vision to reach a circular economy of chemicals to achieve the ambition of net zero by 2050, and the gaps emerging in the current ecosystem.
Sustainability spotlight
This article describes the current status and future prospects of transforming the UK's Chemical Industry into a Circular Economy. The olefins resources flow, particularly ethylene and propylene, are of overwhelming importance to the chemical industry, accounting for >70% of all organic chemical production. They can be readily converted into a wide range of products, such as polymers, chemical fibres, solvents, synthetic rubber, and high-value speciality chemicals. These intermediates subsequently feed other manufacturing and industrial sectors to produce useable end products. However, current olefin production accounts for 25% of direct CO2 emissions for the chemical sector whilst, downstream olefin conversion and use can be estimated to contribute at least 7% of the 66 Mt per year industrial CO2 emissions in the UK. Moving from the linear, fossil-based supply chain and moving to a new circular chemical economy offers a unique opportunity to (1) limit CO2 emissions and (2) high volumes of waste by recovering and re-using the material. It also (3) offers means to re-use captured industrial CO2 emissions as a feedstock to generate added-value streams. This work is directly linked to UN SDGs 8 (decent work and economic growth), 9 (industry innovation and infrastructure), 11 (sustainable cities and communities) and 12 (responsible consumption and production). However, further impacts in reducing waste to air, land and water, supporting a just transition to net zero and the interface with other sectors such as energy, food and healthcare would impact across most or all SDGs. This manuscript sets the scene for multidisciplinary research challenges to be addressed by the community within the next decade, in order to meet our sustainability commitments.
|
Methods
The information in this paper has been the result of a review of current literature, workshops conducted by the UKRI interdisciplinary Centre for Circular Economy of Chemicals and interviews with existing stakeholders across the chemical supply chain to gain the insight for gaps and industry needs.
State of play – emissions and waste
Chemical production is ubiquitous in all areas of our daily lives, the fibres in our clothes, the food and drink we consume (and the packaging it is held in), the precious metals used to connect us to the rest of the world, homecare products and fuel for our vehicles and homes. With continued global population growth (9.7 billion expected by 2050) and consumption trends, the demand for chemicals is projected to grow four-fold by 2050 unless more sustainable models are implemented into society to reduce mass consumption.1,2 Chemical production is important in ensuring continued economic prosperity. The chemical industry contributes a staggering $5.7 trillion to the global economic output and supports 120 million jobs worldwide.1 However, the current consumption and production of chemicals has a large contribution to greenhouse gas emissions, with the chemical industry responsible for 16% of direct global CO2 emissions alone.3 Greenhouse gas emissions associated with chemical production can be categorised into two key components, the first is energy consumption and second is the feedstocks associated with producing chemicals. It is particularly energy intensive to make high-value primary chemicals such as methanol, ethylene, propylene, ammonia and BTX (benzene, toluene and xylene), which account for roughly two thirds of the sector's total energy consumption and a large portion of feedstock resource.4
Olefins serves as the building blocks for producing a wide range of products including polymers, fibres, solvents and high-value speciality material. These products are subsequently fed into several manufacturing and industrial sectors to create useable final products. Short-chain olefins, ethylene and propylene, are estimated to be produced at 200 Mt a year globally, the largest-volume petrochemical produced worldwide, their synthesis is the most energy-consuming process in the chemical sector.5 The global carbon demand for organic chemicals and their derived materials currently stands at 450 Mt embedded C per year.6 Embedded carbon refers to the greenhouse gas emissions that arise from the whole production process of chemicals up to their point of use, for context, the UK reported total greenhouse gas emissions of 417 MtCO2e in 2022.7 At present, the feedstock used for organic chemicals comes largely from fossil-based resources, with an estimated 95% of all manufactured goods produced associated with petrochemical feedstock.8 The chemical sector must redesign pathways of olefin production and other primary chemicals to achieve net zero scope 1–3 emissions goals and provide a platform to assist other sectors in their pursuit of net zero targets.
The chemical sector is responsible for large volumes of waste, both from industrial waste and post-consumer waste, often ending in landfill or polluting our natural environment. The use of landfill for waste is not compatible for a healthy and sustainable planet, the avoidance of landfill usage should be prioritised, which can be achieved in a circular economy. End-of-life products from primary chemicals, single-use plastics, combustion gases, and industrial and domestic waste put increasing pressure on waste management systems. Landfill capacity will continue to diminish despite more waste being produced by an ever-growing global population. To redirect waste from landfill, the European Union (EU) has introduced the landfill directive and circular economy action plan, which entail achieving 55% municipal waste recycling rate by 2025 and 10% or less of total amount of municipal waste generated sent to landfill by 2035.9,10 Members states of the EU are obligated to report their progress on meeting agreed targets. Despite Brexit, the UK has also adopted the municipal waste targets set by the EU.11 The department for Environmental Food and Rural Affairs (Defra) are required to produce progress reports on waste targets set by the waste framework directive. Fig. 1 illustrates the split of treatment of municipal waste (MW) in the EU and the UK for 2018 (later statistics not available for the UK).
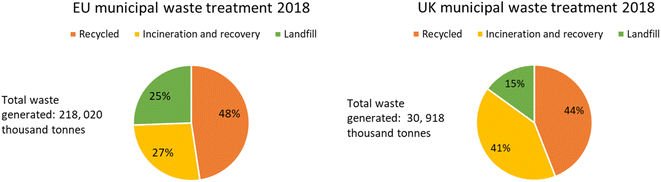 |
| Fig. 1 EU and UK municipal waste treatment statistics for 2018, original data taken and reproduced from Eurostat for EU and Defra stats on waste for UK.12,13 | |
While significant progress has been made in recycling municipal waste, a significant portion continues to be sent to landfill. Incineration and other waste-to-energy technologies are being widely adopted to reach EU and UK waste targets, however there is still some concern over the emission abatement of incineration and whether it is a viable long-term sustainable solution. To control landfill depletion, there is a pressing need to shift our focus toward a systemic priority change for products and waste, which can be underpinned by the waste hierarchy principles.14
Where we need to get to
The chemical industry's current linear production route primarily consumes fossil feedstocks to make valuable products, which are generally discarded as waste at the end of the process. This production route is one of the major contributors to many world crises, such as global warming, energy shortages, competition for natural resources, and long-lasting damage to biodiversity. It is clear a more circular, resource efficient approach is required for the chemical sector to turn from a leading cause of global crises to a potential solution.
Introducing circularity into chemical production offers several key benefits. It reduces the chemical industry's dependency on virgin petrochemical feedstocks, increase resource efficiency, and extend the lifetime of products across the supply chain, in addition to reducing carbon emissions where possible. Proposed technological changes to enable a circular economy of chemicals to operate are reviewed below, with a focus on the EU and UK.
Increasing resource efficiency
The waste hierarchy should be at the heart of any society that reduces waste to a minimum and increases resource efficiency (Fig. 2). By making materials safe and sustainable by design, products can remain in a closed loop system for longer, with the responsibility of products end-of-use distributed evenly along the supply chain and enforced through policy levers.
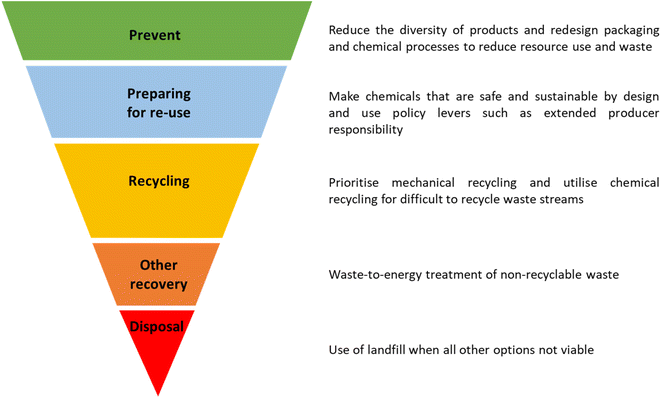 |
| Fig. 2 Applying the waste hierarchy principle to the chemical sector, reproduced from ref. 14 with permission from https://www.gov.uk/, copyright 2011.14 | |
Materials and products should be tracked throughout the supply chain to ensure policy levers can be enforced and maintain a transparent supply chain, for example via a block chain analysis system.15 The UK government are currently consulting on the appropriate method of mass balance to be utilised for chemical recycling.16 Effective use of policy legislation to unify waste collection can provide improvements to the recycling process, allow for higher rates of mechanical recycling to occur, reduce process losses and maintain higher material grades to achieve a zero-waste society. Consumers can play a key role in contributing to a zero-waste society when given the incentive to do so. An example of this is the deposit return scheme, a small deposit is paid when a shopper buys a beverage which they can receive if returned to a deposit system. The deposit return scheme has seen great success in countries such as Denmark, Germany and Lithuania, all of whom reported a high bottle return rate above 90% after adopting this scheme. The EU has set a mandate of 90% for bottle returns for its member states by 2029 under the single use plastic directive, with other EU member states set to make deposit return schemes compulsory.17 The amount of deposit for a bottle can change depending on the type of material for the container, as seen with Germany's Pfand system. Single-use containers are fixed at €0.25 and multi-use containers are set by the producer with a maximum of €0.25 deposit. Coupling societal change with technology and policy progression can ensure the principles of the waste hierarchy are maintained alongside a zero-waste society.
Reducing direct CO2 emissions
Sustainable chemicals and energy use are inherently related when considering the thermodynamics of feedstock conversion. Using CO2 utilisation as one example, CO2 is below all primary chemicals in the thermodynamic energy well, to make primary chemicals there is an inevitable energy penalty for this conversion process. There are a number of established technologies for energy production currently available and capable of supplying power using different (and thus renewable) energy sources. The renewable energy mix technologies most well established are wind, solar photovoltaics (PV) and hydroelectric power. Nuclear power is also seen as a cleaner established alternative to fossil power. Smart grid networks and interconnectors such as the North Sea link, can improve trade of renewable energy and maintain a secure supply of energy on a multinational level. Significant investment into hydrogen and hydrogen storage, such as the UK's investment into the Teesside industrial cluster, will enable hydrogen power to join the energy mix in the future, and can replace existing infrastructure currently relying on natural gas as a fuel source.
Short term effective strategies to decarbonise the chemical sector can be achieved through retrofitting current industrial processes. Some processing equipment can be electrified relatively easily, such as pumps and compressors and boilers which do not reach excessive temperatures.18 Electrifying logistical equipment on industrial sites, such as transport carrier systems, can also lay the foundation towards industrial sites reaching sustainability targets. Large-scale retrofitting such as electrification of steam crackers are currently being explored with SABIC, BASF and Linde joining to develop an electrically heated steam cracker in 2023.19 The merit of emission reduction via electrification however largely depends on the energy source employed, as this process itself is energy intensive it would require a large supply of renewable electricity.20,21 Reforming crackers to use a higher content of blue hydrogen for heating can significantly reduce the CO2 emissions, with the source of hydrogen taken from fuel gas generated in the cracker. Sources of hydrogen can move away from classic steam reforming to electrical routes via water electrolysis, which has been proven at scale.22
Replacing petrochemical feedstocks and valorising waste
The conventional production path to olefins involves the steam cracking of hydrocarbons, from fossil feedstocks. The feedstocks range from light alkanes such as ethane or propane, to complex mixtures such as naphtha found in crude oil. Steam cracking uses extreme pressure and heat, which in turn is highly energy intensive and emits large quantities of greenhouse gases, in the order of 1–2 tonne of CO2 for each tonne of olefins produced. To develop a truly circular economy, not only the energy source but also the carbon feedstock used to produce olefins and specialty chemicals needs to change. There are numerous examples of pathways being developed which can convert conventional waste streams into renewable feedstocks, and thus change the feedstocks employed to create chemicals. The three main feedstocks to displace fossil carbon comprise biomass, solid waste and captured CO2. As a case study, a set of routes for converting these alternative feedstocks into ethylene are detailed in the excerpt below. Through diversifying feedstocks and production methods, a more resilient supply chain can also be built.
A flowchart summarising some of the routes to sustainable ethylene production from biomass, solid waste and capture CO2 feedstocks at various technology readiness levels is shown in Fig. 3, with an accompanying table of abbreviations.
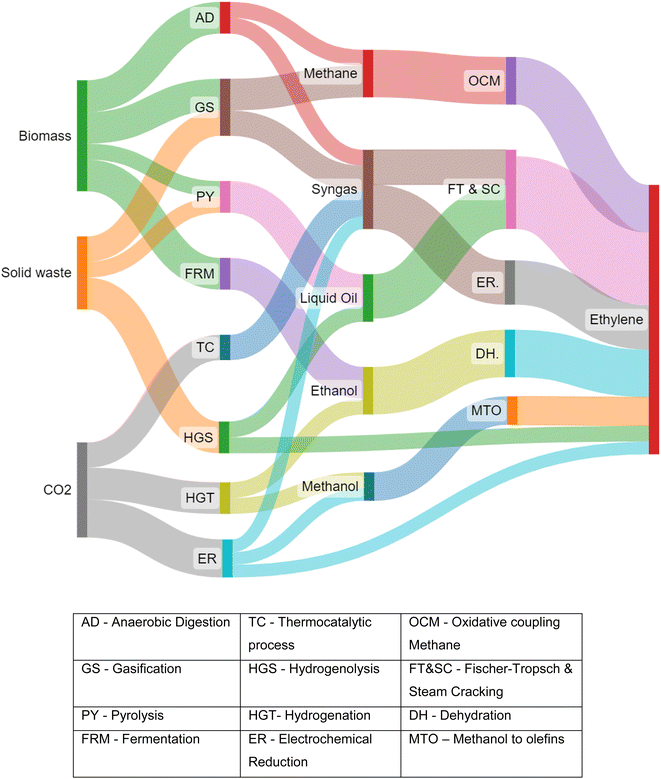 |
| Fig. 3 Flow diagram of alternative sustainable routes to ethylene with accompanying table of abbreviations. | |
Direct conversion
• Solid waste – sorted polyolefinic plastics can be depolymerised into their monomer building blocks through pyrolysis.23
• CO2 conversion – CO2 can be directly converted to olefins via catalytic electrochemical reduction.24,25
Waste feedstock to intermediates
• Biomass – Biomass can be converted into intermediates such as methane and syngas via anaerobic digestion and gasification.19,26 Other thermal cracking routes such as pyrolysis and hydrothermal liquefaction of biomass can achieve a high bio-crude yield.27 Pre-treated waste biomass can also be converted to ethanol via a fermentation process. Although the conversion of biomass feedstocks into bioethanol is currently running at a commercial level with over 5 billion litres a year produced in Europe, it is largely first-generation ethanol from beet sugar feedstock.28 Instead, biomass waste feedstocks comprise wood, food waste, low quality crops, and sewage sludge.
• Solid waste – chemical recycling technologies are being deployed to convert solid waste back into their building constituents. The most common routes involve thermal cracking via either gasification, pyrolysis or hydrothermal liquefaction (HTL). Gasification with steam, oxygen or air as a gasifying agent can produce syngas as an intermediate feedstock and is tolerant to mixed plastic waste streams.29 Pyrolysis and HTL of polyolefinic plastics, on the other hand, can produce a liquid oil alongside gases comprising short-chain alkanes and alkenes.23,30,31 Polyolefinic plastics can also be broken down into alkanes via hydrogenolysis.32,33
• CO2 conversion – electrochemical CO2 reduction through the use of electrolysers can be used to convert waste CO2 gas into syngas, and then further on to make primary chemicals and olefins.24,34,35 Thermocatalytic conversion of CO2 captured from flue gas or directly from air has also grown rapidly, with available routes to syngas, subsequently converted into olefins and other high-value chemicals.36–39 CO2 hydrogenation provides another set of routes to intermediate feedstocks such as methanol40 and ethanol.41
Intermediates to olefins
• Methane – a key pathway from methane to olefins is via oxidative coupling of methane.42,43 An indirect route is via reforming methane to syngas, then converting syngas to olefins.44
• Syngas – syngas can be converted to olefins in a number of ways. The first is via a Fischer–Tropsch synthesis to higher alkanes, followed by cracking to olefins.45 Electrochemical reduction of syngas to olefins is also feasible and currently being studied.45,46 An indirect route would be the synthesis of methanol from syngas, which can then be used to make olefins from the methanol to olefin (MTO) process.
• Methanol and ethanol – the MTO route via dimethyl ether (DME) is a well-established process for the production of olefins such as ethylene and propylene.47 The yield of ethylene from the MTO process is typically quite low for established processes,48 but recent advancements have reported higher yields for ethylene and propylene.49 Projects to convert ethanol into ethylene have also been established for some time via dehydration.50,51
A vision for a circular economy of chemicals
With these principles in mind, a vision for a circular economy of chemicals can be drawn (Fig. 4). By continually keeping products and materials in a closed loop, net zero emissions and waste targets cannot only be achieved but continually maintained indefinitely. Eventually, the need for processes such as energy from waste, shown in Fig. 4, can be phased out as more sustainable technologies mature.
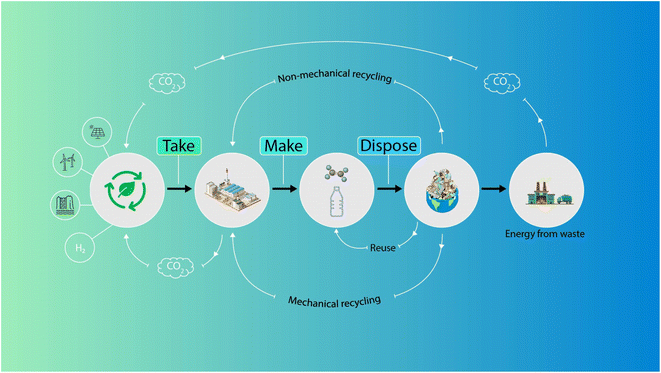 |
| Fig. 4 A vision for a circular economy in the UK and EU chemical sector. | |
Whilst the solution to effectively switch from a linear to circular approach seems a simple one, the implementation of circularity into the chemical sector comes with complexity. A whole systems approach which connects technology, industry, policy, finance and society is needed for the success of chemical circularity. The consideration of economic, societal and technological factors of a product's lifecycle need to be introduced as early as possible for circularity to succeed.
What strategies are being implemented?
The circular economy of chemicals is a focus for the United Nations Development Programme (UNDP) under Goal 12 of the Sustainable Development Goals, ‘Responsible consumption and production’. The UNDP released a strategy on their interventions for proper chemical and waste management to achieve circularity in 2022.52 The G7 alliance on resource efficiency was created to connect researchers, industry, policymakers, private enterprises and relevant stakeholders to improve global resource efficiency. The 5 year Bologna roadmap (2017) outlines priorities for G7 countries towards achieving greater resource efficiency, which the G7 countries pledged to achieve.53 A similar commitment led to the Toyama framework on material cycles in 2016, via the G7 alliance, creating a framework for countries around the world to enhance resource efficiency and promote a circular economy.54 Many countries have also been taking action to curb plastic pollution, with over 60 countries introducing bans and levies on plastic packaging and single-use items. Notably, in March 2022, the UN adopted a historic resolution to end plastic pollution, committing to create an international legally binding agreement by 2024. A binding agreement in this area would be the first of its kind globally.
France has laid out a visionary roadmap to reduce its carbon emissions, ‘France 2030’, backed by a €30 billion investment, with a clear emphasis on fostering innovation in its industrial sector.55 The roadmap includes a new target of 31% lower emissions by 2030, with measurable steps for lowering emissions across the sector.
The United States have set a number of ambitious targets towards a circular economy, with a number of strategies from the environmental protection agency and federal government including a draft national strategy to prevent plastic pollution. The recent inflation reduction act incentivises organisations to move towards a clean energy economy and brought into effect at the beginning of 2023.56
As one of the largest producers of primary chemicals in the chemical sector, the chemical industry in China is continuing to grow. In response, China has set their peak CO2 emissions by 2030 reaching 11.9 GtCO2, and a target to reach carbon neutrality by 2060.57 Their continual 5 year plans provide a detailed guidance for industrial sectors to reduce carbon emissions with the most recent 14th 5 year plan (2021–2025) focusing on circular economy goals.58
The EU has launched a chemicals strategy for sustainability as part of the European green deal.59,60 The strategy aims to promote the approach of ‘safe and sustainable by design’ towards chemicals, banning the most harmful chemicals in consumer products and to establish a ‘one substance, one assessment’ process to simplify the assessment of risks and hazards of chemicals. The chemicals strategy pushes innovation to promote the decarbonisation of the industry, whilst protecting the supply and sustainability of critical chemicals, which coincides with the European Critical Raw Materials Act.61 The European circular economy action plan sets out clear goals to achieve a wider circular economy and acts as a key pillar for the European Green Deal.9
The EU green deal and similar EU incentives have been adopted by the UK. The UK government and the UK Research and Innovation (UKRI) funding agency are supporting projects from industry and academia towards the transition to net zero, through centres such as the National Interdisciplinary Circular Economy Research (NICER) programme. Fig. 5 gives a timeline of key UK and EU strategies related to the chemical industry.
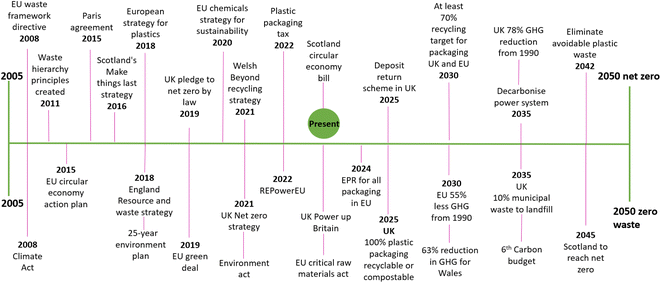 |
| Fig. 5 EU and UK policy strategies and targets towards a net zero timeline. | |
The Netherlands, an example of a nationwide circular economy strategy
National pushes towards a circular economy on all levels can be seen from the example from the Netherlands. In 2016 the government set out a programme for a circular Dutch economy by 2050 with two key objectives, the first is to halve raw material consumption by 2030, and ultimately, to realise a fully circular economy by 2050. The program outlines various strategies and actions to transition the Netherlands towards a circular economy, with three underlying principles identified to accomplishing circularity:
(1) Increase production process efficiency to reduce raw material usage.
(2) When raw materials are needed, use sustainable renewable raw materials to reduce the dependence on fossil fuel resources.
(3) Develop production processes and new products that are circular in nature.
To complement the strategy, a detailed timeline is given to forecast how the three goals will be achieved and eventually achieve a waste-free economy by 2050 on the government website. In 2017, a significant milestone was achieved with the establishment of the raw materials agreement, a pact signed by government and representatives from industry to move towards renewable resources. The raw materials agreement led to the identification of five key sectors (plastics, consumer goods, manufacturing, construction and biomass/food) that can have the greatest impact on the Dutch circular economy. Roadmaps dedicated to each sector were created to describe how each sector can transition to a circular economy. A subsequent circular economy implementation programme was set up to ensure the roadmaps for the five sectors were then given measurable actions and projects from 2019–2023. Progress reports published by the Environmental Assessment Agency and annual national circular economy conferences help to monitor progress and adjust the implementation programme.
As well as leading the way with a national circular economy programme, the Netherlands have the ambition to turn Amsterdam into the first circular city. Amsterdam partnered with Circle Economy to use their City Circle Scan tool identifying areas circular business models can be applied and how the models can be implemented practically. Like the five key sectors for a Dutch circular economy, a roadmap and action agenda has been made for Amsterdam to become fully circular.
Where are the gaps emerging?
The common gaps emerging in the chemical industry provide major barriers to a circular chemical economy and are depicted in Fig. 6 and further discussed below. The gaps presented in Fig. 6 are ubiquitous across the whole supply chain, spanning technology, policy, professional training, public engagement, and finance.
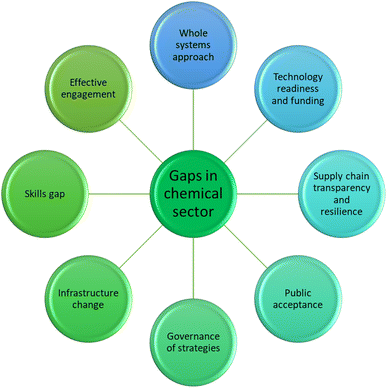 |
| Fig. 6 Common gaps emerging in the chemical industry towards a circular economy of chemicals. | |
Whole systems approach
Whilst there is no shortage of studies focused on developing low-carbon technologies for chemical manufacturing, a more holistic approach is needed to address to the environmental implications of renewable carbon technology beyond reducing emissions. When considering wider system boundaries, Steffen et al. have provided a planetary boundary framework to enable decision makers choose a suitable option for societal development.62 The planetary boundary framework incorporates regional and planetary level boundaries such as ocean acidification, land usage change, biodiversity loss and water intensity. Employing the planetary boundary framework should be practiced for both policy making decisions and strategies to ensure the well-being of society. Bardow et al. apply the planetary boundary framework to create a model for the production and end-of-life treatment of 90% of global plastics.63 Their bottom-up approach concludes that recycling rates of at least 75% with a combination of renewable carbon-based plastics have the potential to operate safely within planetary boundaries. However, it is noted the demand, suitable resource production and waste management of plastics can vary by region.
The Dasgupta review highlights the urgent need to address biodiversity loss and ecological degradation, aligning to the goals of a circular economy.64 The Dasgupta review takes the approach of embedding our economies within nature to fully account for the impact of our demand for natures resources. The recommendations from the review look to couple sustainable practices with preserving nature.
Legislation can play a role in taking a long-lasting approach, such as the Welsh governments well-being of future generations act (2015), which requires public bodies in Wales to embrace a more long-term, whole-system approach to ensure decisions that are made will have a beneficial effect on current and future generations. Approaches like this should be adopted in a wider UK and European context.
Technology readiness with funding gaps
The NASA technology readiness level (TRL) system is widely adopted and adapted across different sectors to assess technology maturity before deployment.65Fig. 7 is a schematic example of TRLs for the chemical sector with conversion technologies for ethylene production. Fundamental technologies lie between TRL levels 1–3, with university research and government funding bodies typically fitting into this category. TRL levels 4–6 are described as emerging technologies, with small and medium enterprises (SMEs), mature institutional projects, and government funding projects operate between these levels. TRL levels 7–9 are described as established technologies, which are often dominated by the private sector. Despite technologies showing promise towards sustainable ethylene production, as shown in Fig. 3, the majority of the technologies are at the emerging stage. It should be noted that some of the technologies categorised as emerging technologies, are at higher TRL levels to produce other chemicals and precursors for olefins. For example, hydrogenation of CO2 to methanol has been industrialised in China, Iceland, and Norway66 and many Fischer–Tropsch processes from syngas are currently used to make fuels at a commercial scale. Retrofitting of traditional crackers, such as electrified crackers and hydrogen-fuelled crackers are also still only at pilot scale.
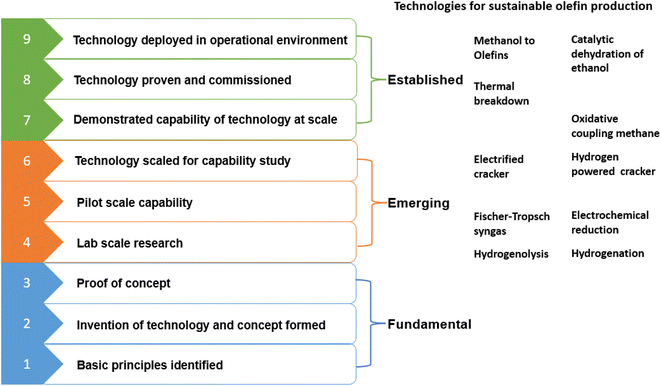 |
| Fig. 7 Technology readiness levels (TRLs) adapted for the chemical sector with highlighted technologies for sustainable olefin production. | |
The current ethylene production capacity globally from 2021 was 214 million metric tons,67 based off an average increase of global capacity (calculated as 5% from 2018–2021), production capacity could reach 332 million metric tons by 2030. The scale of production of sustainable ethylene is far from matching the demand for ethylene production currently or with future forecasting. A common issue with the increase in production for sustainable ethylene lies with the energy intensity and associated cost of emerging technologies in comparison to steam cracking of natural gas and crude oil. Electrification of reforming processes will rely on a constant steady flow of renewable energy. The availability of renewable energy is known to fluctuate over the course of a year, current steam reformers require 24/7 power for operation. Long term renewable energy storage capacity will be required to deal with intermittency in renewable energy generation for chemical production. A combination of renewable energy when there is sufficient sources and use of fossil-derived power when renewable energy sources fluctuate could be a viable path forward until capacity reaches demand for renewable power.
The level of investment and risk greatly increases from an emerging technology (i.e. low level TRL) to an established technology (i.e. high TRL level), particularly for the chemical industry, which is a capital-intensive heavy industry. The difficulty for a company to receive the investment required to move from TRL 4–6 into an established TRL presents a large gap in the market, referred to as the ‘valley of death’, which often prevents SME's from succeeding.68 Accenture conducted financial research on the capital cost to achieve the EU Green Deal's net zero strategy through technology process routes of eight key chemicals; ammonia, ethylene, propylene, soda ash, nitric acid, carbon black, caprolactum and fluorochemicals.69 Their analysis shows that €1 trillion investment is required to cover the modification of existing facilities for renewable chemical production of the 8 chemicals mentioned. The majority of the investment is associated with the standstill costs associated with loss of production due to retrofitting of facilities and transformation of other chemicals to achieve net zero by 2050. The EU chemical industry is currently facing an investment gap of €12 billion a year to fund net-zero initiatives required.
Small and medium enterprises make up 96% of European chemical companies.70 Many SMEs are willing to move towards more sustainable practices, but often do not have the resources for performing and obtaining detailed analytics, such as life-cycle assessment (LCA) or techno-economic analysis (TEA), to inform them of how to successfully transition to a circular economy.
Whilst there are a number of promising technologies that exist for feedstock conversion and end of life treatment, there are few mature technologies producing primary chemicals such as ethylene at the scale required to produce sustainable chemicals. Emerging technologies typically take ten years or longer to reach commercial level, with varying timescales for feedback of investment.
Not only does the lack of funding slow the uptake of new technologies, risk aversion and payback time are often cited as barriers for emerging technologies to move to commercial operation.
Supply chain transparency and resilience
For supply chains to be transparent, there needs to be an effective way to track chemical use and cooperation between all stakeholder and parties across the whole supply chain, with a willingness to share information at all stages along the supply chain. The advancement in digital tracking methods, such as block chain analysis and mass balance methods, are being integrated with chemical supply chains for individuals in the industry. However, there is currently no effective system in place to ensure the chain of custody is maintained over a chemical's lifetime in the chemical sector. Companies will need to comply to honest and open data sharing, as resistance and reluctance to data sharing will inevitably hinder the ability to create transparent supply chains.
Sharing the critical raw materials needed for renewable feedstocks and renewable power technologies is of particular importance towards creating global circular supply chains. Supply chains of critical raw materials are global, with a small number of countries commanding a large share of upstream extraction processes and wider global competition for midstream processes. Recent geopolitical conflicts have seen supply chains severely affected causing shortfalls, for example the ongoing Russia–Ukraine war has highlighted the overreliance on Russia to meet global energy demands and reliance on both nations for food and key minerals. To ensure resilience of supply chains, future supply chains need to incorporate a diverse mix of feedstocks and energy sources as the geo-political landscape remains unstable.
Public acceptance of circular economy
The ‘say-do gap’ is a phenomenon which can be related to the public and business when considering sustainable choices.71 Whilst consumers and businesses often express their intention to make sustainable decisions, studies show these promises do not always align with the action. When clear communication from business and institution is lacking, the trust in circular products will be broken, greenwashing claims for sustainable products are damning examples of the breakdown in trust from business to the public. Priorities of consumers are dependent on the current social and political situation. During the current cost of living crises in the UK and across Europe, the immediate financial concerns of everyday life understandably takes precedence over the sustainability considerations. The financial burden should not be left to the consumer, but instead be assisted and empowered to make a more sustainable choice when buying goods.
There can also be a knowledge gap between a producer and consumer, particularly when considering recycling of plastic packaging. A recent campaign led by the Waste Resources and Action Programme (WRAP) called ‘recycle now’ attempts to encourage correct household recycling across the general public and avoid the spread of ‘wishcycling’, the belief a product is recyclable despite it not being. The campaign has been successful in public behaviour change with motivational clear messaging on packaging, the recycling locator tool, a database on recyclable materials and events such as recycling week. Knowing the nuances of which type of polymer can be recycled on current packaging is confusing and often time consuming for everyone. Clearer messaging on packaging alongside campaigns such as ‘recycle now’ is needed for a greater uptake in correct recycling practices and creating higher quality waste streams for reuse and recycling.
A study by Lamb et al. explored the ‘discourses of climate delay’, where people and organisations often accept climate change, but actually legitimise inaction or inadequate efforts, often through misinformation and unsubstantiated claims.72 The common discourses are categorised into four typologies:
(1) Redirect responsibility; redirecting responsibility places the impetus for change on someone else. An example of redirecting responsibility is the common argument made to not act, the carbon emissions of one nation grossly outweighs ours, so any action we take is pointless.
(2) Push non-transformative solutions; the belief that disruptive changes are not necessary to achieve net zero targets. A poignant example of this in the chemical industry is the technology optimism in the potential of enabling technologies. Instead of focusing on the infrastructure change required to bring the enabling technologies online, the business-as-usual model is continued in the hope that enabling technologies will ultimately provide the solutions required to decarbonise the industry.
(3) Emphasise the downsides of climate policies; by presenting only the downsides of climate policies such as the economic cost, there is a risk that no action is considered a better option than the perceived implications of climate action.
(4) Surrender to climate change. When points 1–3 are pushed and accepted the consensus leads to climate change is inevitable and any action that is taken is too little too late, so why bother.
Transparent campaigning can be an effective tool to mitigate these discourses and ensure correct messaging is delivered to the public, whilst showing that a circular economy is achievable and beneficial to the global population.
Governance of strategies to meet global emission goals
As well as national strategies, industry and the private sector are setting their own net zero strategies and roadmaps. Whilst these are welcome moves towards net zero goals, there is a disconnect between creating such strategies and enforcing them. Reports from the International Energy Agency (IEA) and the Climate Change Committee (CCC) show that the chemical sector is not on track for net zero by 2050.73,74 A recent study by the net zero stocktake shows that whilst over 90% of European countries have incorporated net zero strategies through policy documentation or law enforcement, more than half of countries globally are yet to set their own goals.75
Strategies and roadmaps are often cited as lacking the detail required to action the targets they set out. One such example is the UK government's ‘Net Zero Strategy’, which was found to be unlawful in a UK high court ruling.73 The strategy did not have the legally required information required to show how climate policies would meet the carbon budgets set out in the climate change act.76
Ability of existing infrastructure to change
An effective chemical management system is vital for any future circular economy of chemicals. A recent evidence report by Fidra highlighted that one important gap in the UK is the current chemical management infrastructure.77 Fidra identified the potential impact of chemical pollutants embedded in products for chemical circularity with respect to flame retardants used in mattresses. As a bulky waste item and an estimated 6.4 million disposed of in 2020, mattresses are a priority in the resources and waste strategy to divert mattresses from landfill. However, some common flame-retardant chemicals in mattresses such as decabromodiphenyl ether (DBE-209), are difficult to reuse or recycle as chemical flame retardants are listed under the Stockholm convention. The case study found a combination of outdated rigid fire safety regulations and a lack of chemical transparency presented major barriers to effective recycling of mattresses, without significant contamination of harmful chemicals into making other products.
When considering the use of waste as a resource, effective sorting of waste should be a priority to maximise the recovery rate for waste. Inefficient and inconsistent waste collection is a key barrier often cited. In Biffa's waste net zero report (2022), the starkest problem to meet 65% municipal recycling rate by 2035 was said to be a lack of provision for separate food waste collection for household and businesses.78 A potentially valuable resource (food waste) is sent to landfill or energy recovery with the additional issue of contaminating other materials which would otherwise be fit for recycling. In kerbside collection and sorting, there can be difficulties in determining a materials initial primary use (i.e., a high-density polyethylene milk bottle vs. high density polyethylene cleaning product), leading to mixed waste streams in the recycling process. For food packaging materials and similar product streams, waste streams need to be able to meet food-grade standards. One highlight of this is the UK plastic packaging tax, which requires that a minimum level of 30% recycled plastic content must be used in future plastic packaging. If the recycled polyethylene terephthalate plastic (rPET) waste streams are not efficiently sorted, food-grade waste streams can be contaminated and unfit for use.
In some cases, technologies are available and ready to contribute towards reducing carbon emissions, but the current infrastructure cannot support this much needed change. Using renewable power generation as an example, in the UK power grid, renewable energy has increased power generation in the last decade from 2.5 GW in 2012 to 10.5 GW of power generated in 2022, a share of 35% of power generation for the UK.79 Despite a strong performance in developing renewable energy technologies to meet the fossil fuel replacement demand (currently ∼30 GW fossil power), connection to the grid is a major challenge, with reports suggesting renewable projects can wait up to ten years to connect to the grid.80 The UK government recently released their Powering up Britain strategy, which aims to secure energy security through diversification of energy sources.81 The strategy acknowledges infrastructure issues with the grid by setting up the Nationally Significant Infrastructure Project (NSIP) to increase the uptake of renewable projects to the UK grid.
Skills gaps
As the industry changes, the skills required of the workforce will also need to change for more circular practices i.e. effective resource management strategies, sustainable raw material procurement, sustainable process design. A recent study undertaken by the Royal Society of Chemistry found that 68% of professional chemists believed there was a gap between current chemical knowledge and what is required for future green jobs.82 Universities keep introducing new courses into their syllabi and creating doctoral training programmes that focus on sustainable chemistry and chemical engineering, with businesses also introducing carbon literacy programmes for their employees by working with the carbon literacy project. As part of the EU Green Deal, the EU has introduced the European sustainability competence framework (GreenComp), to act as a common source for educators, and to provide training programmes that teach sustainability at all ages and education levels.
Effective engagement
National research centres can work alongside industry, non-profit organisations, governments, and funding bodies to ensure a circular economy of chemicals is achieved. However, one common problem found with multidisciplinary groups and projects is clarity in conveying technical subject matter, when members of the project have different expertise. The individual who is communicating information assumes other individuals have similar background and depth of knowledge to understand the information, often referred to as the “curse of knowledge”. Examples should be taken from the ‘National Circular Hotspots’ as created in the Netherlands, and from the Finnish ‘National Roadmap to a Circular Economy’, to illustrate how effective engagement can drive a circular economy. The messaging is clear and simple, making it easier for many working groups to understand the role they can play in a national circular economy.
Metrics play a large role when it comes to engaging in the transition towards a circular economy within the chemical industries. To make informed funding decisions, comparable metrics are required to determine best practice and to help inform the most effective levers for sustainable change. The analytical techniques used to measure circularity can vary depending on the performance indicators, making direct comparisons for greater resource efficiency difficult. A unified assessment criteria when analysing a processes sustainability and feasibility (Life Cycle Assessment and Technoeconomic analysis) needs to be in place. The international organisation for standardisation (ISO) has released ISO 14040 for the principles and framework for life cycle assessment analysis (LCA),83 however does not go into depth on methodologies for each phase of an LCA. The ISO are currently creating a framework for standardised eco-technoeconomic analysis under ISO 14076 for commonly accepted best practices for process systems at all scale.84 Guidelines for LCA for Carbon Capture and Utilisation by Muller and Katelhon et al.,85 go into greater detail to try to unify LCA studies with respect to CCU. Guidelines such as Muller and Katelhon et al. should be applied to other low-carbon technologies.
Simplified metrics can help reduce the difficulty of assessing a technology's suitability or comparing a range of candidate technologies to improve circularity in the chemical industry for decision makers. Simplified metrics can also help overcome the barrier of public acceptance, by giving relatable metrics and data to the general public. Consequently, if everyone understands the data on circularity, there will be a greater drive from the public and decision makers towards new and sustainable approaches.
Conclusion
The myriad of strategies, pledges and action plans depict an encouraging perspective for the chemical industry to achieve net zero, however the evidence of progress towards those goals have been found wanting. Whilst there are a number of solutions emerging for the chemical industry to transition towards net zero goals in the UK and EU, there a significant number of gaps that must be overcome to achieve net zero. The vision towards a circular economy of chemicals has been set out and the gaps to enable this vision are apparent. Whilst it is clear industry, government and the public are willing to fill the gaps presented, a unified whole systems approach from the chemical industry is still required to facilitate this change. The chemical industry must not lose sight of the significant role it can play within a circular economy, creating a safe, resource efficient society within stable planetary boundaries.
Conflicts of interest
There are no conflicts to declare.
Acknowledgements
The authors thank the UKRI Interdisciplinary Centre for Circular Chemical Economy EP/V011863/1. We thank Katie Lamb, the University of Sheffield, for valuable discussions.
References
-
Oxford Economics, The Global Chemical Industry: Catalyzing Growth and Addressing Our World's Sustainability Challenges – Oxford Economics, 2019, accessed Jun. 26, 2023, online, available: https://www.oxfordeconomics.com/resource/the-global-chemical-industry-catalyzing-growth-and-addressing-our-world-sustainability-challenges/ Search PubMed.
-
Department of Economic and Social Affairs, United Nations, World Population Prospects 2022: Summary of Results, 2022 Search PubMed.
- M. Takht Ravanchi and S. Sahebdelfar, Appl. Petrochem. Res., 2014, 4, 63–77 CrossRef CAS.
- P. G. Levi and J. M. Cullen, Environ. Sci. Technol., 2018, 52, 1725–1734 CrossRef CAS PubMed.
- G. Centi, E. A. Quadrelli and S. Perathoner, Energy Environ. Sci., 2013, 6, 1711 RSC.
-
F. Kähler, M. Carus, O. Porc and C. Vom Berg, Turning off the Tap for Fossil Carbon Future Prospects for a Global Chemical and Derived Material Sector Based on Renewable Carbon, 2021, online, available: https://www.renewable-carbon.eu/publications Search PubMed.
-
Office for National Statistics, 2022 UK greenhouse gas emissions, provisional figures, https://assets.publishing.service.gov.uk/government/uploads/system/uploads/attachment_data/file/1147372/2022_Provisional_emissions_statistics_report.pdf, accessed 26 February 2024 Search PubMed.
- G. Fiorentino, A. Zucaro and S. Ulgiati, Energy, 2019, 170, 720–729 CrossRef CAS.
-
European Commission, A new Circular Economy Action Plan, 2020, accessed: Sep. 02, 2023, online, available: https://www.un.org/sustainabledevelopment/sustainable-consumption-production/ Search PubMed.
-
European Commission, Directive (EU) 2018/of the European Parliament and of the Council of 30 May 2018 Amending Directive 1999/31/EC on the Landfill of Waste, 2018 Search PubMed.
-
Food and Rural Affairs, Department of Environment, The Waste (Miscellaneous Amendments) (EU Exit) Regulations 2019, Statute Law Database, 2019, accessed: Sep. 02, 2023, online, available: https://www.legislation.gov.uk/uksi/2019/620/regulation/1 Search PubMed.
-
Eurostat, Municipal waste by waste management operations, https://ec.europa.eu/eurostat/databrowser/view/ENV_WASMUN__custom_6659939/default/table?lang=en, accessed 26 June 2023 Search PubMed.
- UK statistics on waste, https://www.gov.uk/government/statistics/uk-waste-data/uk-statistics-on-waste#key-points, accessed 31 May 2023.
- Guidance on applying the Waste Hierarchy, 2011, accessed May 31, 2023, online, available: https://www.defra.gov.uk.
-
P. C. Sauer, G. Orzes and G. Culot, Production Planning & Control, 2022, pp. 1–16 Search PubMed.
- Plastic packaging tax – chemical recycling and adoption of a mass balance approach, https://www.gov.uk/government/consultations/plastic-packaging-tax-chemical-recycling-and-adoption-of-a-mass-balance-approach, accessed 13 October 2023.
- European Commission, Directive (EU) 2019/904 of the European parliament and of the council of 5 June 2019 on the reduction of the impact of certain plastic products on the environment, Official Journal of the European Union, 2019, accessed: Sep. 02, 2023, online, available: https://eur-lex.europa.eu/legal-content/EN/TXT/?uri=OJ:L:2019:155:TOC.
- J. P. Lange, Energy Environ. Sci., 2021, 14, 4358–4376 RSC.
- Greener chemicals: steam cracking could go electric by 2023 – News – The Chemical Engineer, https://www.thechemicalengineer.com/news/greener-chemicals-steam-cracking-could-go-electric-by-2023/, accessed 29 August 2023.
- C. Chen, Y. Lu and R. Banares-Alcantara, Appl. Energy, 2019, 243, 71–90 CrossRef CAS.
- L. S. Layritz, I. Dolganova, M. Finkbeiner, G. Luderer, A. T. Penteado, F. Ueckerdt and J.-U. Repke, Appl. Energy, 2021, 296, 117049 CrossRef CAS.
- T. Terlouw, C. Bauer, R. Mckenna and M. Mazzotti, Energy Environ. Sci., 2022, 15, 3583 RSC.
- W. Kaminsky, Fuel Communications, 2021, 8, 100023 CrossRef.
- M. Golam Kibria, J. P. Edwards, C. M. Gabardo, C.-T. Dinh, A. Seifitokaldani, D. Sinton, E. H. Sargent, M. G. Kibria, C. Dinh, A. Seifitokaldani, E. H. Sargent, J. P. Edwards, C. M. Gabardo and D. Sinton, Adv. Mater., 2019, 31, 1807166 CrossRef PubMed.
- L. Berkelaar, J. van der Linde, J. Peper, A. Rajhans, D. Tiemessen, L. van der Ham and H. van den Berg, Chem. Eng. Res. Des., 2022, 182, 194–206 CrossRef CAS.
- W. Wang and D. J. Lee, Bioresour. Technol., 2021, 323, 124626 CrossRef CAS PubMed.
- R. K. Mishra, V. Kumar, P. Kumar and K. Mohanty, Fuel, 2022, 316, 123377 CrossRef CAS.
- E. B. Sydney, L. A. J. Letti, S. G. Karp, A. C. N. Sydney, L. P. de S. Vandenberghe, J. C. de Carvalho, A. L. Woiciechowski, A. B. P. Medeiros, V. T. Soccol and C. R. Soccol, Bioresour. Technol. Rep., 2019, 7, 100234 CrossRef.
- S. M. Santos, A. C. Assis, L. Gomes, C. Nobre and P. Brito, Waste, 2022, 1, 140–165 CrossRef.
- S. H. Gebre, M. G. Sendeku and M. Bahri, ChemistryOpen, 2021, 10, 1202–1226 CrossRef CAS PubMed.
- G. C. Laredo, J. Reza and E. Meneses Ruiz, Cleaner Chemical Engineering, 2023, 5, 100094 CrossRef.
- P. A. Kots, B. C. Vance and D. G. Vlachos, React. Chem. Eng., 2021, 7, 41–54 RSC.
- L. Yao, J. King, D. Wu, J. Ma, J. Li, R. Xie, S. S. C. Chuang, T. Miyoshi and Z. Peng, Nat. Commun., 2022, 13, 885 CrossRef CAS PubMed.
- B. Siritanaratkul, P. K. Sharma, E. H. Yu, A. J. Cowan, B. Siritanaratkul, A. J. Cowan, P. K. Sharma and E. H. Yu, Adv. Mater. Interfaces, 2023, 10, 2300203 CrossRef CAS.
- A. Ozden, F. P. García de Arquer, J. E. Huang, J. Wicks, J. Sisler, R. K. Miao, C. P. O'Brien, G. Lee, X. Wang, A. H. Ip, E. H. Sargent and D. Sinton, Nat. Sustain., 2022, 5, 563–573 CrossRef.
- E. A. Quadrelli, G. Centi, J. L. Duplan and S. Perathoner, ChemSusChem, 2011, 4, 1194–1215 CrossRef CAS PubMed.
- C. Hepburn, E. Adlen, J. Beddington, E. A. Carter, S. Fuss, N. Mac Dowell, J. C. Minx, P. Smith and C. K. Williams, Nature, 2019, 575, 87–97 CrossRef CAS PubMed.
-
A. A. Kiss, E. Zondervan, R. Lakerveld, L. Özkan, A. Bernardi, J. E. A. Graciano and B. Chachuat, Production of Chemicals from Syngas: an Enviro-Economic Model-Based Investigation, 2019 Search PubMed.
-
A.-E. LanzaTech, The LanzaTech Process, 2015 Search PubMed.
- J. Gao, C. Jia and B. Liu, Catal. Sci. Technol., 2017, 7, 5602–5607 RSC.
- S. S. Ali, S. S. Ali and N. Tabassum, J. Environ. Chem. Eng., 2022, 10, 106962 CrossRef CAS.
- L. S. Layritz, I. Dolganova, M. Finkbeiner, G. Luderer, A. T. Penteado, F. Ueckerdt and J.-U. Repke, Appl. Energy, 2021, 296, 117049 CrossRef CAS.
- V. Spallina, I. C. Velarde, J. A. M. Jimenez, H. R. Godini, F. Gallucci and M. Van Sint Annaland, Energy Convers. Manag., 2017, 154, 244–261 CrossRef CAS.
- I. Amghizar, L. A. Vandewalle, K. M. Van Geem and G. B. Marin, Engineering, 2017, 3, 171–178 CrossRef CAS.
- M. Zhang, R. Chen, S. Wang, Y. Tu, X. Cui and D. Deng, EES Catal., 2023, 1, 250–254 RSC.
- D. Klüh, H. Nieminen, K. Melin, A. Laari and T. Koiranen, Front. Energy Res., 2023, 11, 1129076 CrossRef.
- M. R. Gogate, Pet. Sci. Technol., 2019, 37, 559–565 CrossRef CAS.
- P. Tian, Y. Wei, M. Ye and Z. Liu, ACS Catal., 2015, 5, 1922–1938 CrossRef CAS.
- J. Q. Chen, A. Bozzano, B. Glover, T. Fuglerud and S. Kvisle, Catal. Today, 2005, 106, 103–107 CrossRef CAS.
- D. Fan, D.-J. Dai and H.-S. Wu, Materials, 2013, 6, 101–115 CrossRef CAS PubMed.
- BASF, Ethanol to Ethylene (E2E), https://chemicals.basf.com/global/en/Catalysts/hydrogenation-specialty/products-we-offer/alumina/Ethanol-to-Ethylene-E2E.html, accessed 28 September 2023.
- Transitioning to a Circular Economy Through Chemical and Waste Management, United Nations Development Programme, https://www.undp.org/publications/transitioning-circular-economy-through-chemical-and-waste-management, accessed 12 May 2023.
-
G7 alliance for resource efficiency, 5-Year Bologna Roadmap on Resource Efficiency, 2017 Search PubMed.
-
Institute for Global Environmental Strategies and Ministry of the Environment, Toyama Framework on Material Cycles, 2016 Search PubMed.
-
Major objectives, https://www.gouvernement.fr/france-2030/objectifs, accessed 2 September 2023.
- H.R.5376 – 117th Congress (2021–2022), Inflation Reduction Act of 2022, https://www.congress.gov/bill/117th-congress/house-bill/5376, 2022.
- Y. Li, Y. Mei, T. Zhang and Y. Xie, Front. Environ. Sci., 2022, 10, 1648 Search PubMed.
- United Nations Development Programme, China's 14th five-year plan (English translation), 2021, accessed: Sep. 02, 2023, online, available: http://www.xinhuanet.com/english/2017-10/17/c_136686770.htm.
- European commission, Chemicals Strategy for Sustainability Towards a Toxic-Free Environment, 2020, online, available: https://echa.europa.eu/hot-topics/chemicals-strategy-for-sustainability.
-
European commission, European green deal, 2019, online, available: https://www.europarl.europa.eu/RegData/etudes/ATAG/2019/644205/EPRS_ATA(2019)644205_EN.pdf#:%7E:text=The%20European%20Green%20Deal%20is%20a%20programme%20outlined,just%20transition%20for%20the%20regions%20and%20workers%20affected Search PubMed.
-
I. E. and Sme. Directorate-General for Internal Market, European Critical Raw Materials Act, 2023 Search PubMed.
- W. Steffen, K. Richardson, J. Rockström, S. E. Cornell, I. Fetzer, E. M. Bennett, R. Biggs, S. R. Carpenter, W. de Vries, C. A. de Wit, C. Folke, D. Gerten, J. Heinke, G. M. Mace, L. M. Persson, V. Ramanathan, B. Reyers and S. Sörlin, Science, 2015, 347, 6223 CrossRef PubMed.
- M. Bachmann, C. Zibunas, J. Hartmann, V. Tulus, S. Suh, G. Guillén-Gosálbez and A. Bardow, Nat. Sustain., 2023, 6, 599–610 CrossRef.
-
H. M. Treasury, The Economics of Biodiversity: the Dasgupta Review Search PubMed.
-
J. C. Mankins, Technology Readiness Levels, White Paper, 1995, vol. 6, p. 1995 Search PubMed.
- R.-P. Ye, J. Ding, W. Gong, M. D. Argyle, Q. Zhong, Y. Wang, C. K. Russell, Z. Xu, A. G. Russell, Q. Li, M. Fan and Y.-G. Yao, Nat. Commun., 2019, 10, 5698 CrossRef CAS PubMed.
- Ethylene production capacity globally 2021, https://www.statista.com/statistics/1067372/global-ethylene-production-capacity/, accessed 28 September 2023.
-
Y. An and Y. Zhang, Sage Open, 2021, vol. 11, 21582440211047580 Search PubMed.
-
Accenture, The Chemical Industry's Road to Net Zero Costs and Opportunities of the EU Green Deal, 2022 Search PubMed.
- 2023 Facts and Figures of the European Chemical Industry, https://cefic.org/a-pillar-of-the-european-economy/facts-and-figures-of-the-european-chemical-industry/, accessed 26 June 2023.
-
R. A. Guzzo, H. R. Nalbantian, and L. F. Parra, A Big Data, Say-Do Approach to Climate and Culture: A Consulting Perspective, in The Oxford Handbook of Organizational Climate and Culture, Oxford Library of Psychology, 2014, p. 197 Search PubMed.
- W. F. Lamb, G. Mattioli, S. Levi, J. T. Roberts, S. Capstick, F. Creutzig, J. C. Minx, F. Müller-Hansen, T. Culhane and J. K. Steinberger, Glob. Sustain., 2020, 3, e17 CrossRef.
- C. Change Committee, Progress in reducing UK emissions – 2023 Report to Parliament, 2023, accessed: Aug. 02, 2023, online, available: https://www.theccc.org.uk/publications.
-
International Energy Agency, Tracking Clean Energy Progress 2023, IEA, Paris, 2023 Search PubMed.
-
K. Sanderson, Net-zero pledges are growing — how serious are they?, Nature, 2023, accessed: Jun. 26, 2023, online, available: https://www.nature.com/articles/d41586-023-01976-0 Search PubMed.
- R (Pantellerisco and others) v SSWP, Friends of the Earth vs. Secretary of state for Business, Energy and Industrial Strategy, vol. 1454, October, 2021, pp. 1–76, available: https://www.judiciary.uk/wp-content/uploads/2022/07/FoE-v-BEIS-judgment-180722.pdf.
-
H. Evans, J. Cloy, Managing Chemicals of Concern within a Circular Economy: The Impacts and Solutions for Chemical Flame Retardant Use in UK Mattresses, 2023, accessed: Jun. 01, 2023, online, available: https://www.fidra.org.uk/wp-content/uploads/The-Impacts-and-Solutions-for-Chemical-Flame-Retardant-Use-in-UK-Mattresses-Evidence-Review.pdf Search PubMed.
-
Biffa, The Reality Check 2022, 2022 Search PubMed.
- National Grid: Live, https://grid.iamkate.com/, accessed 2 August 2023.
- Renewables projects face 10-year wait to connect to electricity grid, Financial Times, https://www.ft.com/content/7c674f56-9028-48a3-8cbf-c1c8b10868ba, accessed 1 June 2023.
-
Great Britain and HM Government, Powering up Britain, Department for Energy Security and Net Zero, 2023 Search PubMed.
-
Royal Society of Chemistry, Green shoots part 2-Sustainability and the chemistry curriculum, 2022, online, available: https://www.rsc.org/policy-evidence-campaigns/environmental-sustainability/sustainability-reports-surveys-and-campaigns/a-sustainable-chemistry-curriculum/ Search PubMed.
- ISO 14040:2006 - Environmental management — Life cycle assessment — Principles and framework, https://www.iso.org/standard/37456.html, accessed 28 September 2023.
- ISO/WD TS 14076 – Eco-Technoeconomic Analyses: Principles, requirements and guidelines, https://www.iso.org/standard/61119.html, accessed 13 October 2023.
- L. J. Müller, A. Kätelhön, M. Bachmann, A. Zimmermann, A. Sternberg and A. Bardow, Front. Energy Res., 2020, 8, 505883 Search PubMed.
|
This journal is © The Royal Society of Chemistry 2024 |
Click here to see how this site uses Cookies. View our privacy policy here.