DOI:
10.1039/D4SU00520A
(Tutorial Review)
RSC Sustainability, 2024,
2, 3222-3234
Acyloxy, sulfate, and phosphate radicals as hydrogen atom transfer (HAT) agents for direct C(sp3)–H functionalization
Received
28th August 2024
, Accepted 21st September 2024
First published on 23rd September 2024
Abstract
Selective activation of inert and ubiquitous C(sp3)–H bonds has long been a challenging task in organic synthesis, through which chemists can directly synthesize value-added compounds from inexpensive and readily available alkane feedstocks. By means of modern photochemistry, electrochemistry, as well as traditional thermochemistry, diverse hydrogen atom transfer (HAT) protocols have been established, employing various radicals, especially oxygen-centered ones, as the HAT agents. This review focuses on three unique classes of oxygen radicals, namely acyloxy, sulfate, and phosphate radicals, which have demonstrated significant potential for achieving direct intermolecular C(sp3)–H bond functionalization via HAT pathways. By focusing on the key developments from 2014 to 2024, this review discusses the generation mechanisms, reactivity characteristics and applications of these acid-related oxygen radicals, aiming to provide researchers with insights to further advance the techniques and innovations in the future C(sp3)–H functionalization strategy development.
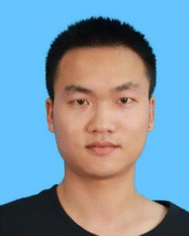 Jia-Lin Tu | Jia-Lin Tu was born in Hubei Province, China, in 1996. He obtained his degree in Medicinal Chemistry from Soochow University in 2021. In the same year, he joined the research group of Professor Wujiong Xia at Harbin Institute of Technology, focusing on the applications of iron photocatalysis in C–H activation and decarboxylation reactions, as well as photoelectrocatalysis. |
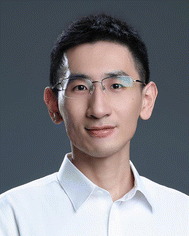 Binbin Huang | Binbin Huang obtained his bachelor's degree from Zhejiang University in 2014. Later, he acquired both master's (2016) and PhD (2021) degrees under the supervision of Prof. Wujiong Xia at Harbin Institute of Technology. After graduation, he took a position in Beijing Normal University at Zhuhai. His research interest mainly focuses on the development of sustainable organic synthetic protocols that are enabled by photo- and electro-chemical methods. |
Sustainability spotlight
In recent years, C(sp3)–H activation has emerged as a crucial area in organic synthesis, allowing for the direct utilization of unprefunctionalized alkane feedstocks to construct complex molecules. Hydrogen atom transfer (HAT), representing a sustainable approach for C–H activation under relatively mild conditions, has seen significant development in the last decades. This review highlights the use of acyloxy, sulfate, and phosphate radicals as three unique classes of oxygen-based radicals as HAT reagents for achieving direct C(sp3)–H functionalization. This research contributes to UN Sustainable Development Goals: industry, innovation, and infrastructure (SDG 9), responsible consumption and production (SDG 12), and climate action (SDG 13).
|
1. Introduction
Selective C(sp3)–H activation stands as a pivotal yet challenging domain in organic synthesis, which significantly broadens the repertoire of synthetic methodologies, enabling the direct construction of value-added compounds from readily available alkane feedstocks, thereby avoiding the tedious pre-functionalization procedures.1
Throughout the development of direct C(sp3)–H bond functionalization, intermolecular hydrogen atom transfer (HAT) processes enabled by organic photocatalysis2 (involving photosensitizers such as decatungstate,3 eosin Y and its close relatives,4 ketones,5 and metal chlorides6), electrochemical or photo-electrochemical methods,7 and traditional thermally-promoted reactions have played significant roles. Taking advantage of various reactive radicals as the HAT agents, including chlorine radical,6,7d–g,8 bromine radical,9 carbon-centered radicals,10 nitrogen-centered radicals,7b,c,11 oxygen-centered radicals,4,5,12etc., these protocols have provided diversified and more sustainable options for achieving direct C(sp3)–H functionalization. Among these, oxygen radical-mediated HAT processes have become one of the most extensively studied subjects.
Apart from the “direct HAT” mediated by eosin Y and its close relatives4 and ketones (mainly diaryl ketones and anthraquinone)5 under light irradiation, most of the oxygen radical-mediated transformations can be classified as “indirect HAT” (wherein the real HAT species are generated in situ from certain oxygen-containing precursors).2 Some representative oxygen-based precursors, as well as radicals for indirect HAT processes are highlighted in Scheme 1:
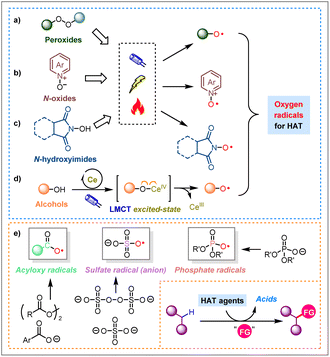 |
| Scheme 1 Representative oxygen-centered radicals as HAT reagents. (a) Peroxides as oxygen radical precursors; (b) N-oxides as oxygen radical precursors; (c) N-hydroxyimides as oxygen radical precursors; (d) alcohols as oxygen radical precursors; (e) the focus of this review: acyloxy, sulfate, and phosphate radicals as HAT agents for direct C(sp3)–H functionalization. | |
(a) Peroxides, such as tert-butyl hydroperoxide (TBHP) and di-tert-butyl peroxide (DTBP), can undergo cleavage under light irradiation, heating, or single-electron transfer (SET), to yield alkoxy radicals as HAT agents (Scheme 1a).13
(b) N-Oxides, such as pyridine N-oxide and its derivatives, are competent of generating oxygen radicals for HAT processes upon single-electron oxidation (Scheme 1b).14
(c) N-Hydroxyimides, such as N-hydroxyphthalimide (NHPI) and N-hydroxyimide (NHI), serve as highly efficient indirect HAT reagents, which are capable of generating reactive oxygen radicals upon photo-irradiation or electrochemical activation (Scheme 1c).12e,15
(d) Alcohols, can engage in photo-induced ligand-to-metal charge transfer (LMCT) mechanism with cerium salts (Scheme 1d), or be oxidized by excited-state photosensitizers to access alkoxy radicals.12b,16
Beyond the above well-established categories, this review will shed light on three classes of acid-related oxygen-centered radicals, whose roles as unique HAT agents are often overlooked: (1) acyloxy radicals (mainly aryl substituted ones), (2) sulfate radical (anion), and (3) phosphate radicals (Scheme 1e).
(1) Aryl substituted acyloxy radicals (Ar–COO˙) exhibit slow decarboxylation rates, which are nearly a thousand times slower than those of alkyl counterparts (Alk–COO˙).17 Their high stability against decarboxylation renders them ideal HAT reagents, particularly for hydrogen atom abstraction from C(sp3)–H bonds, to form aromatic carboxylic acids (Ar–COOH).18 Common generation methods for these radicals include thermal or photolytic decomposition of peroxides (e.g., benzoyl peroxide, BPO),19 and photo-/electro-chemical conversion of carboxylic acids (salts) or derivatives.20,20a,20b In 2016, a seminal publication from the Glorius group demonstrated the use of tetrabutylammonium or sodium benzoate (Bu4N+PhCOO− or Na+PhCOO−) as an HAT reagent for the selective trifluoromethylthiolation of unactivated C(sp3)–H bonds under visible-light induced photoredox catalysis.20c Later in 2017, the same group further developed a visible-light induced alkynylation of Csp2(O)–H bonds of aldehydes, wherein the aryl carboxyl radical derived from sodium 2-iodobenzoate acts as an HAT reagent.20d
(2) Sulfate radical anion (SO4˙−) can be generated from persulfates (S2O82−) through thermo- or photochemically-promoted homolytic cleavage, as well as single-electron reduction events enabled by photochemistry, electrochemistry, among others.21 It was not until recent years did the academic community conduct more in-depth explorations of utilizing sulfate radical anion as an HAT reagent in alkane C(sp3)–H functionalization.22 Notably, in 2023, Ye, Ma, and colleagues reported an innovative protocol to achieve Ritter-type amination of C(sp3)–H bonds, using sulfate radical anion as an HAT agent that is electrochemically generated from cost-effective sulfuric acid (SO42−).23
(3) It is noteworthy that phosphate radicals as HAT reagents have been observed in nature, particularly in the photolysis of DNA, where phosphate radicals generated by high-energy light can abstract hydrogen atoms from the deoxyribose moiety.24 Photo-induced single-electron oxidation of phosphates ((RO)2P(O)O−) readily generates phosphate radicals ((RO)2P(O)O˙) to serve as HAT agents for achieving direct C(sp3)–H functionalization. In 2018, the teams of Alexanian and Nicewicz,25a and Kanai and Oisaki,25b independently reported their studies on the functionalization of C(sp3)–H bonds using phosphate derivatives as HAT reagents. These strategies have further expanded the repertoire of sustainable organic synthesis.
To advance the research of HAT-based C(sp3)–H activation, this review will dissect the generation mechanisms, reaction characteristics, and potential applications of the aforementioned three types of oxygen-centered radicals in HAT processes, with a focus on the developments from 2014 to 2024. Our aim is to provide researchers with a systematic understanding and strategic toolkit, thereby propelling the development of C–H activation techniques in modern organic synthesis.
2. Acyloxy radicals as HAT reagents
2.1. Visible-light induced generation of acyloxy radicals
Visible-light photocatalysis, as an emerging strategy in organic synthesis, has garnered widespread attention from the chemical community in recent years.26 This technique utilizes visible-light as a clean and renewable energy source to excite photosensitizers under mild conditions, thereby initiating a series of synthetically useful transformations.27 Compared to reactions controlled by traditional thermodynamics or kinetics, visible-light photocatalysis offers chemists a new mode of molecule activation, enabling transformations that are elusive under conventional thermal conditions.
Trifluoromethylthiolation is an important functionalization strategy, which can significantly improve the chemical, physical, and biological properties of molecules by modifying them with trifluoromethylthio group (–SCF3), a functionality with high electronegativity and lipophilicity.28 In 2016, Glorius and colleagues disclosed a groundbreaking visible-light-promoted photoredox catalytic system for the selective trifluoromethylthiolation of unactivated C(sp3)–H bonds with Phth–SCF32 (Scheme 2).20c Employing Ir(dF(CF3)ppy)2(dtbbpy)PF6 as the photocatalyst and easily available sodium benzoate (which is equally effective to tetrabutylammonium benzoate) as the HAT catalyst, this reaction proceeds efficiently under the irradiation of 455 nm blue LEDs, exhibiting excellent selectivity in introducing –SCF3 group at the most electron-rich C(sp3)–H bond positions. Notably, high regioselectivity is also observed for the trifluoromethylthiolation of substrates containing multiple tertiary C(sp3)–H bonds. One obvious superiority of this approach is the application of alkane substrates as the limiting reagents, which greatly improves its practicability and potential in the late-stage modification of complex molecules. The proposed reaction mechanism involves the excited state photocatalyst *Ir(III) being reductively quenched by benzoate 4, generating radical Int2-A as a potent HAT agent to abstract a hydrogen atom from 1. The resulting carbon-centered radical Int2-B then reacts with the trifluoromethylthiolating reagent 2 to yield the final product 3. The simultaneously generated Phth˙ is sequentially engaged in SET with Ir(II) and protonation with benzoyl acid, for the regeneration of both ground state photocatalyst Ir(III) and benzoate anion.
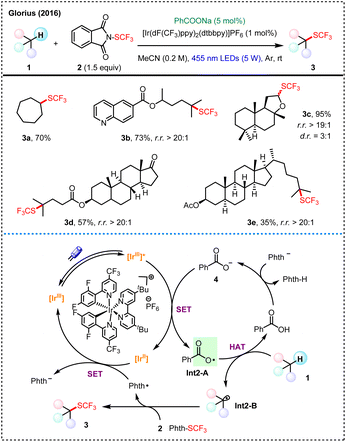 |
| Scheme 2 Selective trifluoromethylthiolation of unactivated C(sp3)–H bonds via visible-light enabled benzoyloxy radical HAT mechanism. | |
Photocatalytic Giese-type radical addition represents an important strategy for the construction of C(sp3)–C(sp3) bonds under mild conditions.29 Recently, Yoshimi and coworkers developed a photoredox HAT system for the direct alkylation of O-α-C(sp3)–H bonds using 4-tert-butylbenzoic acid as the HAT reagent, along with biphenyl (BP) as an electron donor and 9,10-dicyanoanthracene (DCA) as an electron acceptor (Scheme 3).20e Upon exposure to visible-light, a photoinduced electron transfer (PET) process between excited DCA* and BP enables efficient production of aryl acyloxy radical Int3-A, which selectively abstracts a hydrogen atom from O-containing substrate 5 (including acetals, ethers and alcohols), to generate carbon-centered radical Int3-B. The subsequent addition of Int3-B to electron-deficient alkene 6 forges a new C–C bond (Int3-C), and the following SET with DCA˙− furnishes final product 7. Notably, along with the alkylation products, the additive 4-tert-butylbenzoic acid can also be recovered in high yield after reaction.
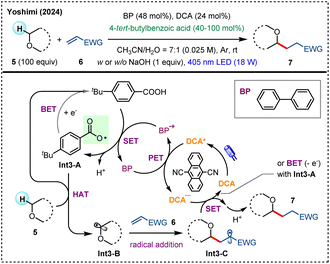 |
| Scheme 3 Visible-light induced, benzoic acid-facilitated HAT for C–C bond formation. | |
N-Heteroaromatic compounds, known for their pronounced bioactivity, have long been a focal point in pharmaceutical and agrochemical research, and the efficient construction and functionalization of these compounds are of great significance in organic synthesis.30 In 2024, Shi, Zhao, and coworkers reported a visible-light induced intermolecular HAT process with BPO as a precursor for benzoyloxy radical (Scheme 4).31 This approach avoids the application of hazardous chemicals and high temperature that are typically associated with such reactions, enabling the Minisci-type dehydrogenative coupling of N-heteroarenes 9 with inert C(sp3)–H substrates 8 in water. This reaction system is compatible with a variety of six-membered N-heterocyclic compounds such as quinolines, isoquinolines and etc., as well as C(sp3)–H compounds, including cyclic ethers, esters, aldehydes, ketones, and even silanes (Si–H). Mechanistically, the reaction initiates with the decomposition of BPO under visible-light irradiation, to generate benzoyloxy radical Int4-A that is capable of abstracting hydrogen atoms from inert C(sp3)–H bonds, resulting in the formation of key alkyl radical Int4-B. This alkyl radical then couples with TFA-protonated N-heteroarene 11 to form an N-radical cationic intermediate Int4-C, which upon further HAT and deprotonation yields the Minisci coupling product 12.
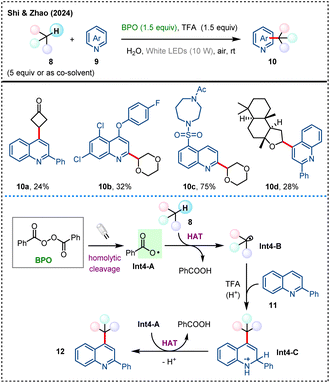 |
| Scheme 4 Visible-light-mediated dehydrogenative coupling of N-heteroarenes with inert C(sp3)–H bonds via BPO-initiated HAT. | |
2.2. Electrochemical generation of acyloxy radicals
Organic electrosynthesis has been increasingly favored in recent years due to its innate advantages that eliminates the reliance on external chemical oxidants/reductants, thereby minimizing side reactions and waste production, which is in accordance with the aim of sustainable chemistry.32 By altering the reaction components (such as electrode materials, electrolytes, solvents, etc.), and precisely adjusting the parameters (such as current and voltage), the conditions can be optimized to enhance the efficiency as well as selectivity of both electro-oxidative and electro-reductive transformations.33
Oxidative C–N bond formation under chemical oxidant-free electrochemical conditions is an attractive strategy for accessing amine derivatives.34 In 2024, the team of Qian, Sun, and Zhang utilized anodic oxidation for acyloxy radical generation from aromatic carboxylic acids 14 to achieve a three-component amidation of C(sp3)–H bonds at benzylic position (Scheme 5).35 The reaction mechanism is proposed as follows: initially, acid 14 is reduced at the cathode to form hydrogen gas and acyloxy anion Int5-A, which then migrates to the anode surface for oxidation to access oxygen-centered radical Int5-B. The following HAT process of Int5-B with substrate 13 results in the formation of benzylic radical Int5-C, which is readily oxidized at the anode to generate cation Int5-D. The next interaction with solvent MeCN and Int5-A, followed by a Mumm rearrangement yield the final product 15.
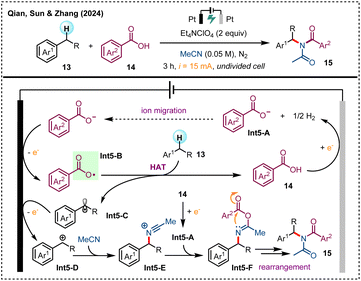 |
| Scheme 5 Electrochemical amidation of benzylic C(sp3)–H bonds via HAT with anodically generated benzoyloxy radicals. | |
2.3. Thermochemical generation of acyloxy radicals
Under heating conditions, benzoyl peroxide (BPO) can decompose to produce reactive benzoyloxy radicals via homolytic O–O bond cleavage, allowing for subsequent HAT processes with C(sp3)–H bonds.19 In the following section, some representative and significant works reported in the past decade are introduced.
Internal alkynes represent an important moiety for cyclization to access five-membered ring systems.36 In 2016, the team led by Pan and Yu disclosed a BPO-enabled oxidative radical cyclization of ynones with alkanes for the synthesis of a series of 2-alkyl-3-aryl indenones 18 (Scheme 6).37 The substrate scope includes diverse alkanes 16 which are used as reaction solvent, and diversely substituted 1,3-diaryl-2-propyn-1-ones 17. The possible mechanism for the reaction is proposed as follows: initially, BPO undergoes homolytic cleavage under heating conditions to generate benzoyloxy radical Int6-A. This radical abstracts a hydrogen atom from alkane 16, forming alkyl radical Int6-B, which then adds onto the C–C triple bond of ynone 17 to reach Int6-C. Next, the intramolecular radical addition of Int6-C results in another radical intermediate Int6-D, which further has a hydrogen atom abstracted by Int6-A to afford the target indenone product 18.
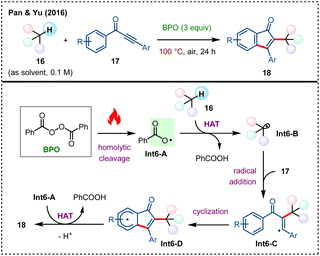 |
| Scheme 6 Metal-free radical oxidative annulation of ynones with alkanes to access indenones. | |
Microwave dielectric heating shows advantage to dramatically elevate the conversion rates of organic synthetic transformations, thereby reducing reaction times from days and hours to minutes and seconds.38 In 2022, Li, Wang, Zhou, and colleagues developed a BPO-initiated microwave-accelerated cyclization of 1,5-enynes with cycloalkanes, providing an efficient approach to exocyclic indane derivatives 21 (Scheme 7).39 Through deuterium labeling experiments, radical trapping experiments, and mass spectroscopy studies, a reasonable reaction mechanism is proposed. Under heating and microwave irradiation, BPO readily generates benzoyloxy radical Int7-A, which acts as an initiator to abstract a hydrogen atom from cycloalkane 19 to form alkyl radical Int7-B. This alkyl radical then selectively attacks the carbon–carbon double bond of the 1,5-enyne 20 to form a tertiary carbon radical Int7-C, which subsequently cyclizes with the adjacent internal alkyne moiety to afford intermediate Int7-D. Ultimately, cyclization product 21 is formed through an HAT event with cycloalkane 19, meanwhile generating another Int7-B.
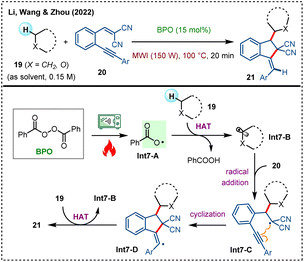 |
| Scheme 7 Microwave-accelerated synthesis of exocyclic indane derivatives via C–H activation and 1,5-enyne cyclization. | |
Cross dehydrogenative coupling (CDC) reactions for direct C(sp3)–C(sp2) bonds formation have garnered considerable research attention in recent years.40 In 2022, Zhou and Sun et al. developed a microwave-accelerated CDC reaction of quinoxalin-2(1H)-ones with alkanes under metal-free conditions (Scheme 8a).41 A wide range of simple alkanes 22 are applied as alkyl radical precursors under microwave-assisted BPO-promotion to achieve C–H alkylation of diversely substituted quinoxalin-2(1H)-ones 23 and some other nitrogen-containing heteroaromatics. Later in 2023, Liu, Zhang and colleagues reported another metal-free microwave-accelerated CDC reaction for the C5-alkylation of N-(quinolin-8-yl)amides 27 with acetone 25 or acetonitrile 26, using BPO as a benzoyloxy radical source (Scheme 8b).42
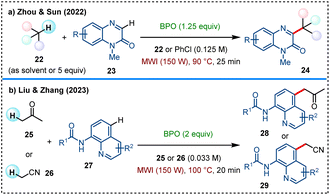 |
| Scheme 8 Microwave-accelerated CDC reactions for direct C(sp3)–C(sp2) bonds formation. | |
3. Sulfate radical anion as an HAT reagent
3.1. Visible-light induced generation of sulfate radical anion
Persulfate salts (e.g., K2S2O8, Na2S2O8, and (NH4)2S2O8), commonly serving as an oxidizing reagent in chemical reactions,21,43 begins to demonstrate unique potential in achieving direct functionalization of unactivated hydrocarbons when combined with organic photocatalysis, by forming sulfate radical anion (SO4˙−) as a powerful HAT species.22
In 2014, the MacMillan group reported an innovative photocatalytic CDC reaction for achieving α-heteroarylation of ethers (Scheme 9).44 In the mechanistic proposal, sulfate radical anion generated by SET between excited-state photocatalyst and Na2S2O8 acts as the HAT reagent, extracting hydrogen atom from O-α-C(sp3)–H of ethers 33 to form α-oxyalkyl radicals Int9-A. This radical intermediate then couples with protonated heteroarene Int9-B to build a new C–C bond (Int9-C). This method exhibits a broad substrate scope, applicable to a variety of cyclic and acyclic ethers, as well as diverse heteroarenes, including pyridines, quinolones, and their derivatives, demonstrating excellent functional group tolerance and regioselectivity.
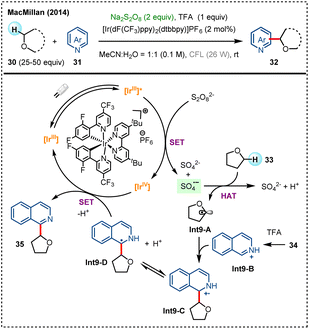 |
| Scheme 9 Photocatalytic α-heteroarylation of ethers with sulfate radical anion as an HAT agent. | |
In 2015, Ji and colleagues developed a photo-induced CDC reaction mediated by benzaldehyde, achieving α-heteroarylation of amides and ethers without the need for metal photocatalyst (Scheme 10).45 The photo-excited benzaldehyde is used to promote a unique decomposition mechanism of ammonium persulfate, generating sulfate radical anion as an powerful HAT reagent. Two possible pathways for the generation of sulfate radical anion are proposed: (a) in the absence of benzaldehyde, the formation of complex Int10-A through hydrogen bonding between (NH4)2S2O8 and amide 37, followed by a donor–acceptor interaction, produce sulfate radical anion (SO4˙−), which is primarily enabled by thermal chemistry; (b) the excited-state benzaldehyde generated under visible-light irradiation facilitates the decomposition of (NH4)2S2O8 to produce SO4˙−, a process that is part of photochemistry. Regardless of whether the sulfate radical anion is generated through thermal or photochemical pathways, it serves as an HAT agent capable of abstracting hydrogen atoms from diverse amides and ethers. Throughout the reaction process, benzaldehyde may participate in a catalytic cycle, ultimately being regenerated to sustain the catalytic process.
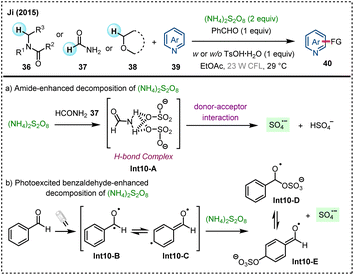 |
| Scheme 10 Photo-induced CDC for α-heteroarene functionalization of amides and ethers without metal catalysts. | |
Electron donor–acceptor (EDA) complexes are molecular aggregates of electron donors and acceptors, which upon light-irradiation can undergo photo-excitation to facilitate the following chemical processes.46 In 2015, Shah et al. developed an external photocatalyst-free CDC reaction, facilitating the activation of C(sp3)–H bonds in ethers to couple with electron-deficient N-heteroarenes under visible-light irradiation (Scheme 11a).47 In this method, N-heteroarenes 42 form EDA complexes (Int11-A) with K2S2O8, which upon visible-light excitation generates sulfate radical anion via homolytic cleavage as the HAT agent for direct C(sp3)–H functionalization of ethers 41. In 2021, Barham's group described a photocatalyst-free, visible-light-mediated direct C(sp3)–H arylation of amides (Scheme 11b).48 In this reaction, solvent-caged EDA complexes are formed between electron-rich (hetero)arenes 45 and persulfate in amides 44 (as solvent), which upon photoexcitation produces sulfate radical anion as the hydrogen abstractor for the activation of N-α-C(sp3)–H bonds.
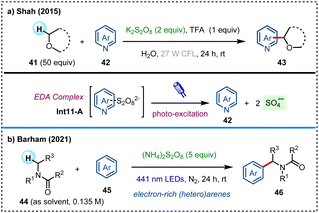 |
| Scheme 11 Visible-light-mediated EDA complex formation for C(sp3)–H bond functionalization. | |
In 2021, Li and coworkers developed a persulfate-catalyzed photocatalytic CDC reaction of N-heteroaromatics with γ-lactams/amides in water (Scheme 12).49 In the presence of catalytic K2S2O8 or (NH4)2S2O8 under air atmosphere, this protocol enables smooth amidoalkylation of various N-heteroaromatics such as quinoxalin-2(1H)-ones (48), quinolines, isoquinolines, phthalazines, and benzothiazoles in high regioselectivity. Upon visible-light irradiation, S2O82− decomposes to SO4˙−, while substrate 48 is also excited to form excited-state 48*. This excited-state molecule (48*) undergoes energy transfer with triplet oxygen to generate singlet oxygen (1O2). The sulfate radical anion (SO4˙−) then undergoes HAT with γ-lactam 50 to form a carbon-centered radical Int12-A (path a), which selectively adds onto the C3-position of 48, establishing a new C–C bond. Finally, the desired product 51 is obtained through an HAT process followed by dehydrogenation steps. Hydrogen peroxide produced during the reaction may undergo homolytic cleavage under visible-light irradiation to generate hydroxyl radical (HO˙), which may participate in the cycle of persulfate. In addition, intermediate HO2˙ may extract hydrogen from 50 to produce H2O2 (path b).
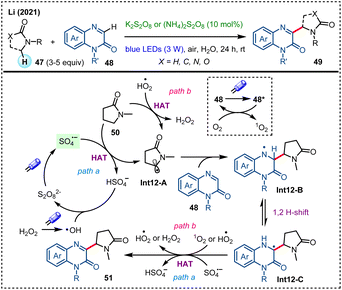 |
| Scheme 12 Visible-light-mediated regioselective amidoalkylation of N-heteroaromatics with γ-lactams/amides in aqueous medium. | |
3.2. Electrochemical generation of sulfate radical anion
Ritter-type reaction of organonitriles represents an important method for the synthesis of amide compounds.50 In 2022, Ye, Ma and their colleagues developed an innovative electrosynthetic strategy that utilizes sulfate ions (SO42−) of cost-effective sulfuric acid as a key additive to achieve Ritter-type amination of inert C(sp3)–H bonds (Scheme 13).23 Upon anodic oxidation of SO42−, sulfate radical anion SO4˙− is readily generated to serve as an HAT reagent, facilitating the direct amination of C(sp3)–H bonds of common alkanes with diverse organonitriles with excellent site selectivity.
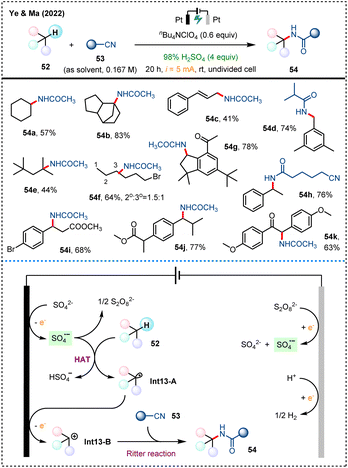 |
| Scheme 13 Electrochemical synthesis of amides via sulfate-mediated Ritter-type C(sp3)–H amination. | |
In terms of the mechanism for this electrochemical Ritter-type amination,23 the single-electron oxidation of SO42− at the anode to form sulfate radical anions (SO4˙−) serves as the key step. This radical anion is capable of abstracting a hydrogen atom from substrate 52, generating carbon radical Int13-A, which is further oxidized at the anode to form carbocation intermediates Int13-B. Subsequently, nitrile 53 attack the carbocation Int13-B, leading to the formation of the final amination product 54 through a Ritter reaction pathway. Additionally, the dimerization of sulfate radical anions forms persulfate ions (S2O82−), which are transferred to the cathode and reduced back to SO42− and SO4˙−. This method paves a convenient and flexible pathway for realizing synthetically useful transformations of C(sp3)–H bonds mediated by sulfate radical anion generated via electrochemistry.
3.3. Thermochemical generation of sulfate radical anion
In 2015, the team led by Chen and Liu developed a potassium persulfate-mediated direct C(sp3)–H trifluoromethylthiolation, utilizing silver(I) trifluoromethanethiolate (AgSCF3, 56) as the –SCF3 source (Scheme 14).51 Under relatively mild conditions at 60 °C, this reaction demonstrates a broad substrate scope, including a variety of unactivated alkanes. K2S2O8 is proposed to play a dual role in this system: on one hand, it decomposes to produce sulfate radical anion that abstracts hydrogen atoms from C(sp3)–H bonds to form key alkyl radical Int14-A; on the other hand, it is also responsible for the oxidation of AgSCF3, generating radical ˙SCF3, Ag(II)SCF3 or CF3S–SCF3 intermediates, which are key to the formation of the final trifluoromethylthiolation product 57.
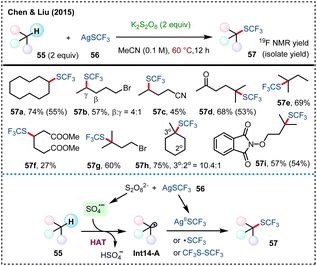 |
| Scheme 14 Direct trifluoromethylthiolation of unactivated C(sp3)–H bonds under thermal conditions. | |
The homolytic cleavage of S2O82− triggered by heating at certain temperature generates sulfate radical anion to enable HAT processes, thereby facilitating C–N and C–C bonds formation via direct C(sp3)–H functionalization. In 2015, Tang and coworkers developed an sulfate radical-mediated azidation of unactivated aliphatic C–H bonds (Scheme 15a).52 This transition-metal-free method utilizes an easily handled sulfonyl azide 59 as the azide source, showcasing practicability for scale-up reactions and late-stage azidation of natural product derivatives. In 2019, Weng and coworkers reported a hydroxyalkylation of benzothiazoles with alcohols/ethers in aqueous solution that is mediated by sulfate radicals (Scheme 15b).53 This method takes advantage of the high solubility of inorganic salts in water with simplified post-treatment process, showing good substrate applicability and functional group compatibility.
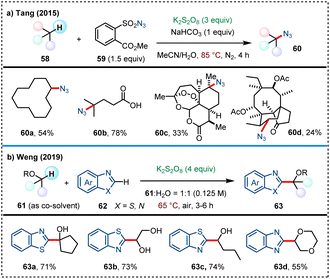 |
| Scheme 15 Sulfate radical-mediated direct C(sp3)–H azidation and heteroarylation. | |
Later in 2020, Ryu's group developed a site-selective alkenylation of alkanes in the presence of persulfate (Scheme 16).54 Using 1,2-bis(phenylsulfonyl)ethane 65 as the alkenylating reagent, this method is capable of directly modifying C(sp3)–H bonds to access diverse (E)-2-alkylvinylphenylsulfones 66, with new C(sp3)–C(sp2) bonds forged. Notably, direct alkenylation of some steric hindered C(sp3)–H bonds can also be achieved due to the compact size of sulfate radicals. This strategy offers a broad substrate scope and excellent site selectivity, providing an effective tool for complex molecule synthesis.
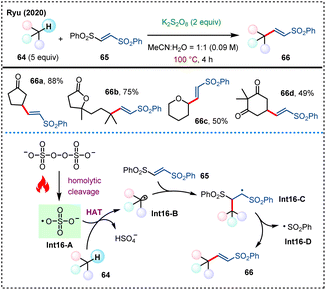 |
| Scheme 16 Compact sulfate radical-mediated C(sp3)–H alkenylation under heating conditions. | |
4. Phosphate radicals as HAT reagents
Compared with acyloxy and sulfate radicals, phosphate radicals have been relatively underexplored in the application of mediating HAT processes. The current transformations have mainly relied on visible-light photocatalytic methods.
In 2018, Alexanian, Nicewicz and their colleagues reported a general strategy combining photoredox catalysis with phosphate salts to achieve direct azidation of aliphatic C–H bonds (Scheme 17).25a Initially, the researchers hypothesized that the highly oxidizing acridinium salts (Mes–Acr+) could be used to oxidatively generate heteroatom-centered radicals with HAT ability, facilitating the functionalization of C(sp3)–H bonds with a sulfonyl group transfer reagent. A system combining K3PO4 with 1,1,1,3,3,3-hexafluoroisopropanol (HFIP) is then screened out to effectively facilitating the C–H azidation, using 68 as the azide source. The mechanism is proposed as follows: under the excitation of 455 nm LEDs, the acridinium salt photosensitizer undergoes SET with the phosphate salt, generating an oxygen-centered radical Int17-A which upon HAT with alkane 67 results in carbon-centered radical Int17-B. This radical is then trapped by the sulfonyl azide 68 to form the desired product 69, while also generating a sulfonyl radical Int17-C. Notably, using different radical acceptors, a variety of C(sp3)–H functionalizations have also been realized, including fluorination, bromination and trifluoromethylthiolation, among others. These transformations demonstrate the versatility of this photoredox catalytic system, allowing for the modular incorporation of diverse functional groups through the application of different radical trapping agents.
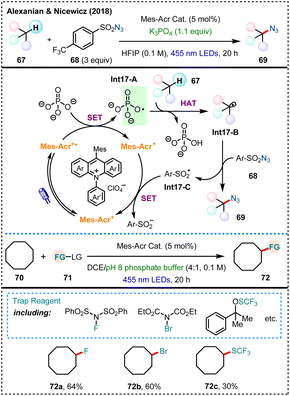 |
| Scheme 17 A general strategy for aliphatic C–H functionalization enabled by organic photoredox catalysis. | |
In the same year, the research team led by Kanai and Oisaki developed an innovative cyanation for C(sp3)–H bonds under visible-light photoredox catalysis in the presence of a phosphate catalyst (Scheme 18).25b Under the irradiation of 430 nm blue light, the phosphate salt produces a phosphorus-based oxygen radical Int18-A through single-electron oxidation, which acts as an HAT agent to efficiently activate C(sp3)–H bonds with relatively high bond dissociation energies (BDEs). The resulting radical Int18-B then react with the cyanide source 74 to form cyanation product 75. This method showcases excellent functional group compatibility, along with large potential in the late-stage modification of complex molecules, broadening the horizon for pharmaceutical synthesis.
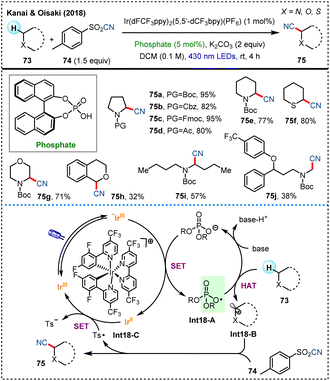 |
| Scheme 18 Phosphate-radical-mediated visible-light photoredox cyanation of C(sp3)–H bonds. | |
In 2022, the team of Deng and Wu developed a photo-induced Minisci-type CDC reaction between alkanes and heteroarenes in a metal- and external oxidant-free manner (Scheme 19a).55 The success of this transformation lies in the use of an elegantly designed stop-flow microtubing (SFMT) reactor to enhance the light penetration while stabilizing volatile and gaseous reagents and intermediates. The key additive diphenyl phosphate plays a dual role both in activating heteroarene substrates and in generating phosphate radicals to promote the HAT process triggered by photoredox catalysis. Later in 2024, Gonzalez-Gomez, Bosque, and coworkers also reported a highly selective hydroxymethylation of azaarenes with methanol, employing chlorine or phosphate radicals as the HAT reagents with a acridine photocatalyst under photo-electrochemical conditions (Scheme 19b).56 Under irradiation of 455 nm blue light, the PET process of acridinium intermediate Int19-A provides the key phosphate radical Int19-C as a selective HAT reagent. Instead of chemical oxidants, electrochemical oxidation is applied for the regeneration of the photocatalyst, thereby further improving the sustainability of this transformation.
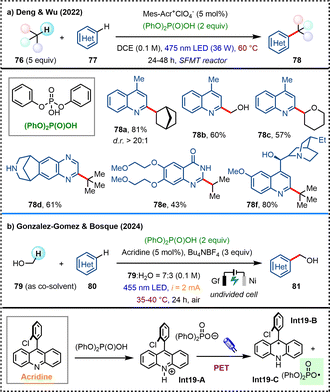 |
| Scheme 19 Phosphate radical enabled visible-light induced CDC reaction of heteroarenes with alkanes/alcohols. | |
5. Summary and outlook
This review delves into the innovative applications of acyloxy, sulfate, and phosphate radicals in intermolecular HAT reactions for achieving direct C(sp3)–H bond functionalization. Methods such as organic photocatalysis, electrosynthesis, and thermochemistry-enabled reactions have been utilized for the generation of these acid-related radicals as unique and promising categories of HAT species. Specifically, acyloxy radicals, mainly the aryl substituted ones, exhibit remarkable stability, offering high efficiency and selectivity for the hydrogen abstraction from C(sp3)–H bonds. Sulfate radical (anion), generated from easily available persulfates or sulfates through thermo-, photo- or electrochemical methods, provides a cost-effective and sustainable choice for C(sp3)–H functionalization. Phosphate radicals, as inspired by natural processes, provide a novel strategy for C(sp3)–H activation, which exhibits remarkable potential for further exploration. The generation mechanisms, reactivity characteristics and the applications in selective HAT processes of these oxygen-centered radicals are discussed, underscoring their potential to advance C–H activation methodologies in modern organic synthesis.
Looking ahead, future research may focus on establishing more sustainable reaction conditions for the facile generation of these radicals, broadening the range of substrates that these radicals can effectively engage with, as well as exploring their potential in asymmetric C(sp3)–H functionalization. As the field of C–H activation continues to evolve, these acid-related radicals are anticipated to play increasingly prominent roles, offering new strategies and tools for chemists to innovate the synthetic methodologies.
Data availability
No primary research results, software or code have been included and no new data were generated or analysed as part of this review.
Conflicts of interest
There are no conflicts to declare.
Acknowledgements
The authors are grateful for the financial support from Beijing Normal University (No. 310432105), and Harbin Institute of Technology (Shenzhen).
Notes and references
-
(a) L. Guillemard, N. Kaplaneris, L. Ackermann and M. J. Johansson, Nat. Rev. Chem, 2021, 5, 522–545 CrossRef CAS PubMed;
(b) N. Holmberg-Douglas and D. A. Nicewicz, Chem. Rev., 2022, 122, 1925–2016 CrossRef CAS PubMed;
(c) P. Bellotti, H.-M. Huang, T. Faber and F. Glorius, Chem. Rev., 2023, 123, 4237–4352 CrossRef CAS PubMed;
(d) X. Wang, J. He, Y.-N. Wang, Z. Zhao, K. Jiang, W. Yang, T. Zhang, S. Jia, K. Zhong, L. Niu and Y. Lan, Chem. Rev., 2024, 124, 10192–10280 CrossRef CAS PubMed.
-
(a) L. Capaldo and D. Ravelli, Eur. J. Org Chem., 2017, 2017, 2056–2071 CrossRef CAS PubMed;
(b) L. Capaldo, D. Ravelli and M. Fagnoni, Chem. Rev., 2022, 122, 1875–1924 CrossRef CAS PubMed;
(c) H. Cao, X. Tang, H. Tang, Y. Yuan and J. Wu, Chem Catal., 2021, 1, 523–598 CrossRef CAS;
(d) J.-L. Tu, Y. Zhu, P. Li and B. Huang, Org. Chem. Front., 2024, 11, 5278–5305 RSC.
- Selected reviews and articles:
(a) P. P. Singh, S. Sinha, P. Gahtori, S. Tivari and V. Srivastava, Org. Biomol. Chem., 2024, 22, 2523–2538 RSC;
(b) B.-C. Hong and R. R. Indurmuddam, Org. Biomol. Chem., 2024, 22, 3799–3842 RSC;
(c) I. B. Perry, T. F. Brewer, P. J. Sarver, D. M. Schultz, D. A. DiRocco and D. W. C. MacMillan, Nature, 2018, 560, 70–75 CrossRef CAS PubMed;
(d) S. Zhou, T. Liu and X. Bao, J. Catal., 2022, 415, 142–152 CrossRef CAS;
(e) Q. Wang, S. Ni, X. Wang, Y. Wang and Y. Pan, Sci. China: Chem., 2022, 65, 678–685 CrossRef CAS;
(f) Q. Liu, Y. Ding, Y. Gao, Y. Yang, L. Gao, Z. Pan and C. Xia, Org. Lett., 2022, 24, 7983–7987 CrossRef CAS PubMed;
(g) P. Li, J.-L. Tu, A.-M. Hu, L. Guo, C. Yang and W. Xia, Org. Biomol. Chem., 2024, 22, 3420–3424 RSC.
- Selected reviews and articles:
(a) D.-M. Yan, J.-R. Chen and W.-J. Xiao, Angew. Chem., Int. Ed., 2019, 58, 378–380 CrossRef CAS PubMed;
(b) J. Inoa, G. Dominici, R. Eldabagh, J. J. I. V. Foley and Y. Xing, Synthesis, 2021, 53, 2183–2191 CrossRef CAS;
(c) D. Singla, V. Luxami and K. Paul, Org. Chem. Front., 2023, 10, 237–266 RSC;
(d) N. Nagasundaram, U. Peroli, R. Venkatesh, N. Vinoth and A. Lalitha, Tetrahedron Lett., 2023, 117, 154366 CrossRef CAS;
(e) H. Cao, D. Kong, L.-C. Yang, S. Chanmungkalakul, T. Liu, J. L. Piper, Z. Peng, L. Gao, X. Liu, X. Hong and J. Wu, Nat. Synth., 2022, 1, 794–803 CrossRef;
(f) V. Srivastava, P. K. Singh and P. P. Singh, Tetrahedron Lett., 2019, 60, 1333–1336 CrossRef CAS.
- Selected reviews and articles:
(a) J. A. Dantas, J. T. M. Correia, M. W. Paixão and A. G. Corrêa, ChemPhotoChem, 2019, 3, 506–520 CrossRef CAS;
(b) D.-L. Zhu, J. Li, D. J. Young, Y. Wang and H.-X. Li, Asian J. Org. Chem., 2023, 12, e202300275 CrossRef CAS;
(c) V. Iziumchenko and V. Gevorgyan, Synlett, 2023, 34, 1289–1308 CrossRef CAS;
(d) C.-Y. Huang, J. Li, W. Liu and C.-J. Li, Chem. Sci., 2019, 10, 5018–5024 RSC;
(e) S. Zhang, S. Cao, Y.-M. Lin, L. Sha, C. Lu and L. Gong, Chin. J. Catal., 2022, 43, 564–570 CrossRef CAS;
(f) N. Ishida, Y. Masuda, Y. Imamura, K. Yamazaki and M. Murakami, J. Am. Chem. Soc., 2019, 141, 19611–19615 CrossRef CAS PubMed;
(g) J. Xu and B. Liu, Chem.–Eur. J., 2024, 30, e202400612 CrossRef CAS PubMed.
- Selected reviews and articles:
(a) F. Juliá, ChemCatChem, 2022, 14, e202200916 CrossRef;
(b) X.-Y. Yuan, C.-C. Wang and B. Yu, Chin. Chem. Lett., 2024, 35, 109517 CrossRef CAS;
(c) S. M. Treacy and T. Rovis, J. Am. Chem. Soc., 2021, 143, 2729–2735 CrossRef CAS PubMed;
(d) J.-L. Tu, A.-M. Hu, L. Guo and W. Xia, J. Am. Chem. Soc., 2023, 145, 7600–7611 CrossRef CAS PubMed;
(e) H. Liu, K. Wang, S. Ye, Q. Zhu and H. Huang, Org. Chem. Front., 2024, 11, 2027–2032 RSC;
(f) P. Li, J.-L. Tu, H. Gao, C. Shi, Y. Zhu, L. Guo, C. Yang and W. Xia, Adv. Synth. Catal., 2024, 366, 220–224 CrossRef CAS;
(g) P. Li, J.-L. Tu, A.-M. Hu, Y. Zhu, J. Yin, L. Guo, C. Yang and W. Xia, Org. Lett., 2024, 26, 6347–6352 CrossRef CAS PubMed.
- Selected reviews and articles:
(a) Z. Shen, J.-L. Tu and B. Huang, Org. Chem. Front., 2024, 11, 4024–4040 RSC;
(b) H. Li, J. Tong, Y. Zhu, C. Jiang, P. Liu and P. Sun, Green Chem., 2022, 24, 8406–8411 RSC;
(c) J. Zhao, J. Zhang, P. Fang, J. Wu, F. Wang and Z.-Q. Liu, Green Chem., 2024, 26, 507–512 RSC;
(d) P. Xu, P.-Y. Chen and H.-C. Xu, Angew. Chem., Int. Ed., 2020, 59, 14275–14280 CrossRef CAS PubMed;
(e) P.-F. Zhong, J.-L. Tu, Y. Zhao, N. Zhong, C. Yang, L. Guo and W. Xia, Nat. Commun., 2023, 14, 6530 CrossRef CAS PubMed;
(f) L. Wang, X. Huo, X. He, L. Ackermann and D. Wang, Green Chem., 2024, 26, 8315–8322 RSC;
(g) Y. Cao, C. Huang and Q. Lu, Nat. Synth., 2024, 3, 537–544 CrossRef.
- Selected reviews and articles for HAT with chlorine radical:
(a) M. Sadeghi, Adv. Synth. Catal., 2024, 366, 2898–2918 CrossRef CAS;
(b) Z. Wang, C.-X. Yan, R. Liu, X. Li, J. Dai, X. Li and D. Shi, Sci. Bull., 2024, 69, 345–353 CrossRef CAS PubMed;
(c) Y. Liu, B. Nie, N. Li, H. Liu and F. Wang, Chin. J. Catal., 2024, 58, 123–128 CrossRef CAS;
(d) S. Bonciolini, T. Noël and L. Capaldo, Eur. J. Org Chem., 2022, 2022, e202200417 CrossRef CAS;
(e) N. Xu, X. Peng, C. Luo, L. Huang, C. Wang, Z. Chen and J. Li, Adv. Synth. Catal., 2023, 365, 142–147 CrossRef CAS;
(f) V. Carré, P. Godard, R. Méreau, H.-P. J. de Rouville, G. Jonusauskas, N. McClenaghan, T. Tassaing and J.-M. Vincent, Angew. Chem., Int. Ed., 2024, 63, e202402964 CrossRef PubMed.
- Selected reviews and articles for HAT with bromine radical:
(a) B. Saxena, R. I. Patel and A. Sharma, RSC Sustainability, 2024, 2, 2169–2189 RSC;
(b) P. Jia, Q. Li, W. C. Poh, H. Jiang, H. Liu, H. Deng and J. Wu, Chem, 2020, 6, 1766–1776 CrossRef CAS;
(c) L. Huan, X. Shu, W. Zu, D. Zhong and H. Huo, Nat. Commun., 2021, 12, 3536 CrossRef CAS PubMed;
(d) Z. Ye, Y. Yu, Y.-M. Lin, Y. Chen, S. Song and L. Gong, Nat. Synth., 2023, 2, 766–777 CrossRef;
(e) Q.-L. Wang, H. Huang, G. Mao and G.-J. Deng, Org. Chem. Front., 2023, 10, 890–897 RSC;
(f) X. Zeng, F.-H. Zhang, R. Lai, X. Lin and Z. Wang, Sci. China: Chem., 2024, 67, 1589–1595 CrossRef CAS.
- Selected reviews and articles for HAT with carbon-centered radicals:
(a) S. Sarkar, K. P. S. Cheung and V. Gevorgyan, Chem. Sci., 2020, 11, 12974–12993 RSC;
(b) J.-L. Tu and B. Huang, Chem. Commun., 2024 10.1039/D4CC03383C;
(c) K. P. S. Cheung, J. Fang, K. Mukherjee, A. Mihranyan and V. Gevorgyan, Science, 2022, 378, 1207–1213 CrossRef PubMed;
(d) X.-Y. Ruan, D.-X. Wu, W.-A. Li, Z. Lin, M. Sayed, Z.-Y. Han and L.-Z. Gong, J. Am. Chem. Soc., 2024, 146, 12053–12062 CrossRef CAS PubMed;
(e) I. N.-M. Leibler, M. A. Tekle-Smith and A. G. Doyle, Nat. Commun., 2021, 12, 6950 CrossRef CAS PubMed;
(f) S. Yang, H. Hu, J.-h. Li and M. Chen, ACS Catal., 2023, 13, 15652–15662 CrossRef CAS.
- Selected reviews and articles for HAT with nitrogen-centered radicals:
(a) M. Shee and N. D. P. Singh, Chem. Soc. Rev., 2022, 51, 2255–2312 RSC;
(b) W. Xiao, X. Wang, R. Liu and J. Wu, Chin. Chem. Lett., 2021, 32, 1847–1856 CrossRef CAS;
(c) J. Wang, B. Huang, Y. Gao, C. Yang and W. Xia, J. Org. Chem., 2019, 84, 6895–6903 CrossRef CAS PubMed;
(d) M. Shee and N. D. P. Singh, Adv. Synth. Catal., 2022, 364, 2032–2039 CrossRef CAS;
(e) H. Li, C. Qiang, Y. Liao, Q. Chu, P. Liu and P. Sun, Adv. Synth. Catal., 2023, 365, 3149–3154 CrossRef CAS;
(f) M. Sneha, G. L. Thornton, L. Lewis-Borrell, A. S. H. Ryder, S. G. Espley, I. P. Clark, A. J. Cresswell, M. N. Grayson and A. J. Orr-Ewing, ACS Catal., 2023, 13, 8004–8013 CrossRef CAS PubMed;
(g) R. Mao, S. Bera, A. C. Turla and X. Hu, J. Am. Chem. Soc., 2021, 143, 14667–14675 CrossRef CAS PubMed;
(h) H. Ren, P. Zhang, J. Xu, W. Ma, D. Tu, C.-s. Lu and H. Yan, J. Am. Chem. Soc., 2023, 145, 7638–7647 CrossRef CAS PubMed.
- Selected reviews for HAT with oxygen-centered radicals:
(a) J.-J. Guo, A. Hu and Z. Zuo, Tetrahedron Lett., 2018, 59, 2103–2111 CrossRef CAS;
(b) L. Chang, Q. An, L. Duan, K. Feng and Z. Zuo, Chem. Rev., 2022, 122, 2429–2486 CrossRef CAS PubMed;
(c) A. A. M. A. El Gehani, H. A. Maashi, J. Harnedy and L. C. Morrill, Chem. Commun., 2023, 59, 3655–3664 RSC;
(d) J. Qin, H. Lei, C. Gao, Y. Zheng, Y. Zhao and W. Xia, Org. Biomol. Chem., 2024, 22, 6034–6044 RSC;
(e) Z.-X. Wu, G.-W. Hu and Y.-X. Luan, ACS Catal., 2022, 12, 11716–11733 CrossRef CAS.
- Selected articles:
(a) M. Finn, R. Friedline, N. K. Suleman, C. J. Wohl and J. M. Tanko, J. Am. Chem. Soc., 2004, 126, 7578–7584 CrossRef CAS PubMed;
(b) W.-Z. Bi, W.-J. Zhang, C.-Y. Li, L.-H. Shao, Q.-P. Liu, S.-X. Feng, Y. Geng, X.-L. Chen and L.-B. Qu, Org. Biomol. Chem., 2022, 20, 3902–3906 RSC;
(c) L.-F. Fan, R. Liu, X.-Y. Ruan, P.-S. Wang and L.-Z. Gong, Nat. Synth., 2022, 1, 946–955 CrossRef;
(d) Y. Zhang, N. A. Fitzpatrick, M. Das, I. P. Bedre, H. G. Yayla, M. S. Lall and P. Z. Musacchio, Chem Catal., 2022, 2, 292–308 CrossRef CAS.
- Selected reviews and articles:
(a) H. T. Ang, Y. Miao, D. Ravelli and J. Wu, Nat. Synth., 2024, 3, 568–575 CrossRef;
(b) M. Schlegel, S. Qian and D. A. Nicewicz, ACS Catal., 2022, 12, 10499–10505 CrossRef CAS PubMed;
(c) L. Laze, B. Quevedo-Flores, I. Bosque and J. C. Gonzalez-Gomez, Org. Lett., 2023, 25, 8541–8546 CrossRef CAS PubMed.
- Selected reviews and articles:
(a) J. E. Nutting, M. Rafiee and S. S. Stahl, Chem. Rev., 2018, 118, 4834–4885 CrossRef CAS PubMed;
(b) A. S. Budnikov, I. B. Krylov, A. V. Lastovko, B. Yu and A. O. Terent'ev, Asian J. Org. Chem., 2022, 11, e202200262 CrossRef CAS;
(c) M. A. Hoque, J. Twilton, J. Zhu, M. D. Graaf, K. C. Harper, E. Tuca, G. A. DiLabio and S. S. Stahl, J. Am. Chem. Soc., 2022, 144, 15295–15302 CrossRef CAS PubMed;
(d) C. Yang, L. A. Farmer, E. C. McFee, R. K. Jha, S. Maldonado, D. A. Pratt and C. R. J. Stephenson, Angew. Chem., Int. Ed., 2024, 63, e202315917 CrossRef CAS PubMed;
(e) Y. Liu, L. Zhang, Y. Zhang, S. Cao, X. Ban, Y. Yin, X. Zhao and Z. Jiang, J. Am. Chem. Soc., 2023, 145, 18307–18315 CrossRef CAS PubMed.
- Selected articles:
(a) X. Zhang, S. Ning, Y. Li, Y. Xiong and X. Wu, ChemCatChem, 2023, 15, e202300311 CrossRef CAS;
(b) A. Hu, J.-J. Guo, H. Pan and Z. Zuo, Science, 2018, 361, 668–672 CrossRef CAS PubMed;
(c) F. Liu, S. Ma, Z. Lu, A. Nangia, M. Duan, Y. Yu, G. Xu, Y. Mei, M. Bietti and K. N. Houk, J. Am. Chem. Soc., 2022, 144, 6802–6812 CrossRef CAS PubMed.
-
(a) H. Misawa, K. Sawabe, S. Takahara, H. Sakuragi and K. Tokumaru, Chem. Lett., 1988, 17, 357–360 CrossRef;
(b) J. Chateauneuf, J. Lusztyk and K. U. Ingold, J. Am. Chem. Soc., 1988, 110, 2886–2893 CrossRef CAS;
(c) J. W. Hilborn and J. A. Pincock, J. Am. Chem. Soc., 1991, 113, 2683–2686 CrossRef CAS;
(d) J.-L. Tu, Z. Shen and B. Huang, Adv. Synth. Catal., 2024 DOI:10.1002/adsc.202400573.
-
(a) Y. Li, J. Zhang, D. Li and Y. Chen, Org. Lett., 2018, 20, 3296–3299 CrossRef CAS PubMed;
(b) Y. Zhang, A. A. Tamijani, M. E. Taylor, B. Zhi, C. L. Haynes, S. E. Mason and R. J. Hamers, J. Am. Chem. Soc., 2019, 141, 8277–8288 CrossRef CAS PubMed.
- Selected articles:
(a) L. Wang, W. Sha, Q. Dai, X. Feng, W. Wu, H. Peng, B. Chen and J. Cheng, Org. Lett., 2014, 16, 2088–2091 CrossRef CAS PubMed;
(b) C. Pan, D. Gao, Z. Yang, C. Wu and J.-T. Yu, Org. Biomol. Chem., 2018, 16, 5752–5755 RSC;
(c) L. Zhou and H. Togo, Eur. J. Org Chem., 2019, 2019, 1627–1634 CrossRef CAS;
(d) N. Okugawa, K. Moriyama and H. Togo, J. Org. Chem., 2017, 82, 170–178 CrossRef CAS PubMed;
(e) N. Okugawa, K. Moriyama and H. Togo, Eur. J. Org Chem., 2015, 2015, 4973–4981 CrossRef CAS.
-
(a) S. Zhang, L. Li, H. Wang, Q. Li, W. Liu, K. Xu and C. Zeng, Org. Lett., 2018, 20, 252–255 CrossRef CAS PubMed;
(b) M. Yu, Y. Gao, L. Zhang, Y. Zhang, Y. Zhang, H. Yi, Z. Huang and A. Lei, Green Chem., 2022, 24, 1445–1450 RSC;
(c) S. Mukherjee, B. Maji, A. Tlahuext-Aca and F. Glorius, J. Am. Chem. Soc., 2016, 138, 16200–16203 CrossRef CAS PubMed;
(d) S. Mukherjee, R. A. Garza-Sanchez, A. Tlahuext-Aca and F. Glorius, Angew. Chem., Int. Ed., 2017, 56, 14723–14726 CrossRef CAS PubMed;
(e) M. Hirose, H. Sakaguchi, R. Hashimoto, T. Furutani, M. Yamawaki, H. Suzuki and Y. Yoshimi, Chem.–Eur. J., 2024 DOI:10.1002/chem.202402285;
(f) T. Huang, C. Liu, P.-F. Yuan, T. Wang, B. Yang, Y. Ma and Q. Liu, Green Chem., 2024, 26, 9859–9868 RSC.
-
(a) F. Minisci, A. Citterio and C. Giordano, Acc. Chem. Res., 1983, 16, 27–32 CrossRef CAS;
(b) D. A. House, Chem. Rev., 1962, 62, 185–203 CrossRef CAS;
(c) S. Mandal, T. Bera, G. Dubey, J. Saha and J. K. Laha, ACS Catal., 2018, 8, 5085–5144 CrossRef CAS;
(d) S. Saha and A. K. Bagdi, Org. Biomol. Chem., 2022, 20, 3249–3262 RSC.
- Selected reviews and articles:
(a) S. Sathyamoorthi and S. Banerjee, ChemistrySelect, 2017, 2, 10678–10688 CrossRef CAS;
(b) C. Dai, F. Meschini, J. M. R. Narayanam and C. R. J. Stephenson, J. Org. Chem., 2012, 77, 4425–4431 CrossRef CAS PubMed;
(c) Y. Xiong, Y. Zhang, L. Qi, M. Jiang, J. Zhang and T. Wang, Asian J. Org. Chem., 2020, 9, 292–295 CrossRef CAS;
(d) C. A. D. Zaragoza, G. S. G. Peagno, A. J. A. Minguine and A. G. Salles Jr, Org. Biomol. Chem., 2024, 22, 2359–2364 RSC.
- L. Zhang, Y. Fu, Y. Shen, C. Liu, M. Sun, R. Cheng, W. Zhu, X. Qian, Y. Ma and J. Ye, Nat. Commun., 2022, 13, 4138 CrossRef CAS PubMed.
-
(a) W. K. Pogozelski and T. D. Tullius, Chem. Rev., 1998, 98, 1089–1108 CrossRef CAS PubMed;
(b) A. Adhikary, D. Becker, B. J. Palmer, A. N. Heizer and M. D. Sevilla, J. Phys. Chem. B, 2012, 116, 5900–5906 CrossRef CAS PubMed.
-
(a) K. A. Margrey, W. L. Czaplyski, D. A. Nicewicz and E. J. Alexanian, J. Am. Chem. Soc., 2018, 140, 4213–4217 CrossRef CAS PubMed;
(b) T. Wakaki, K. Sakai, T. Enomoto, M. Kondo, S. Masaoka, K. Oisaki and M. Kanai, Chem.–Eur. J., 2018, 24, 8051–8055 CrossRef CAS PubMed.
-
(a) F. Strieth-Kalthoff, M. J. James, M. Teders, L. Pitzer and F. Glorius, Chem. Soc. Rev., 2018, 47, 7190–7202 RSC;
(b) V. Srivastava, P. K. Singh and P. P. Singh, J. Photochem. Photobiol., C, 2022, 50, 100488 CrossRef CAS;
(c) K. Teegardin, J. I. Day, J. Chan and J. Weaver, Org. Process Res. Dev., 2016, 20, 1156–1163 CrossRef CAS PubMed;
(d) J.-L. Tu and B. Huang, Org. Biomol. Chem., 2024, 22, 6650–6664 RSC;
(e) A. Y. Chan, I. B. Perry, N. B. Bissonnette, B. F. Buksh, G. A. Edwards, L. I. Frye, O. L. Garry, M. N. Lavagnino, B. X. Li, Y. Liang, E. Mao, A. Millet, J. V. Oakley, N. L. Reed, H. A. Sakai, C. P. Seath and D. W. C. MacMillan, Chem. Rev., 2022, 122, 1485–1542 CrossRef CAS PubMed.
- Selected articles:
(a) J.-L. Tu, W. Tang, S.-H. He, M. Su and F. Liu, Sci. China: Chem., 2022, 65, 1330–1337 CrossRef CAS;
(b) X.-K. Qi, M.-J. Zheng, C. Yang, Y. Zhao, L. Guo and W. Xia, J. Am. Chem. Soc., 2023, 145, 16630–16641 CrossRef CAS PubMed;
(c) B. Huang, Y. Li, C. Yang and W. Xia, Green Chem., 2020, 22, 2804–2809 RSC;
(d) X.-K. He, L.-Q. Lu, B.-R. Yuan, J.-L. Luo, Y. Cheng and W.-J. Xiao, J. Am. Chem. Soc., 2024, 146, 18892–18898 CrossRef CAS PubMed;
(e) X. Sun, Y. Liu, Y. Yin, X. Ban, X. Zhao and Z. Jiang, Nat. Chem., 2024, 16, 1169–1176 CrossRef CAS PubMed;
(f) B. Huang, X. Tang, J. Yuan, M. Zhang, Z. Luo, J. Wang and C. Lu, Org. Biomol. Chem., 2024, 22, 6198–6204 RSC;
(g) X. Lei, Y. Wang, S. Ma and P. Jiao, J. Org. Chem., 2024, 89, 7148–7155 CrossRef CAS PubMed.
-
(a) K. Zhang, X. H. Xu and F. L. Qing, Chin. J. Org. Chem., 2015, 35, 556–569 CrossRef CAS;
(b) J.-H. Lin, Y.-L. Ji and J.-C. Xiao, Curr. Org. Chem., 2015, 19, 1541–1553 CrossRef CAS;
(c) F. Li, J.-W. Song, X. Han and C.-P. Zhang, Synthesis, 2024 DOI:10.1055/a-2335-8627.
- Selected reviews and articles:
(a) A. L. G. Kanegusuku and J. L. Roizen, Angew. Chem., Int. Ed., 2021, 60, 21116–21149 CrossRef PubMed;
(b) M. A. Ashley, C. Yamauchi, J. C. K. Chu, S. Otsuka, H. Yorimitsu and T. Rovis, Angew. Chem., Int. Ed., 2019, 58, 4002–4006 CrossRef CAS PubMed;
(c) J. Kuzmin, J. Röckl, N. Schwarz, J. Djossou, G. Ahumada, M. Ahlquist and H. Lundberg, Angew. Chem., Int. Ed., 2023, 62, e202304272 CrossRef CAS PubMed;
(d) J. Wang, Q. Xie, G. Gao, H. Li, W. Lu, X. Cai, X. Chen and B. Huang, Org. Chem. Front., 2023, 10, 4394–4399 RSC;
(e) G. Han, J. You, J. Choi and E. J. Kang, Org. Lett., 2024, 26, 2414–2419 CrossRef CAS PubMed.
- Selected reviews and articles:
(a) M. M. Heravi and V. Zadsirjan, RSC Adv., 2020, 10, 44247–44311 RSC;
(b) S. Hu, Y. Li, W. Kan, T. Ding, H. Gu, T. Zhang, Z. Yi and Y. Chen, Med. Chem. Res., 2022, 31, 936–948 CrossRef CAS;
(c) A. C. Sun, R. C. McAtee, E. J. McClain and C. R. J. Stephenson, Synthesis, 2019, 51, 1063–1072 CrossRef CAS PubMed;
(d) W. Jia, Y. Jian, B. Huang, C. Yang and W. Xia, Synlett, 2018, 29, 1881–1886 CrossRef CAS;
(e) Y. Jiang, K. Xu and C. Zeng, Chem. Rev., 2018, 118, 4485–4540 CrossRef CAS PubMed;
(f) J. Wang, Q. Xie, G. Gao, G. Wei, X. Wei, X. Chen, D. Zhang, H. Li and B. Huang, Org. Chem. Front., 2024, 11, 4522–4528 RSC;
(g) A. Das, S. Gouthaman and K. R. J. Thomas, Green Chem., 2024, 26, 1223–1280 RSC;
(h) J. Wang, G. Chen, C. Shi, Q. Xie, G. Gao, Y. Li, H. Du, X. Cai, H. Li and B. Huang, Synlett, 2024, 35, 1551–1556 CrossRef CAS.
- J.-F. Yang, Y.-F. Liu, L.-L. Wei, K.-K. Qiao, Y.-Q. Zhao and L. Shi, J. Org. Chem., 2024, 89, 4249–4260 CrossRef CAS PubMed.
-
(a) B. A. Frontana-Uribe, R. D. Little, J. G. Ibanez, A. Palmad and R. Vasquez-Medrano, Green Chem., 2010, 12, 2099–2119 RSC;
(b) M. Yan, Y. Kawamata and P. S. Baran, Chem. Rev., 2017, 117, 13230–13319 CrossRef CAS PubMed;
(c) C. Ma, P. Fang, Z.-R. Liu, S.-S. Xu, K. Xu, X. Cheng, A. Lei, H.-C. Xu, C. Zeng and T.-S. Mei, Sci. Bull., 2021, 66, 2412–2429 CrossRef CAS PubMed;
(d) B. Huang, Z. Sun and G. Sun, eScience, 2022, 2, 243–277 CrossRef;
(e) Y. Wang, S. Dana, H. Long, Y. Xu, Y. Li, N. Kaplaneris and L. Ackermann, Chem. Rev., 2023, 123, 11269–11335 CrossRef CAS PubMed;
(f) Z. Yang, W. Shi, H. Alhumade, H. Yi and A. Lei, Nat. Synth., 2023, 2, 217–230 CrossRef.
- Selected articles:
(a) B. Huang, Y. Li, C. Yang and W. Xia, Chem. Commun., 2019, 55, 6731–6734 RSC;
(b) Z.-J. Shen, B. Huang, N. Ma, L. Yao, C. Yang, L. Guo and W. Xia, Adv. Synth. Catal., 2021, 363, 1944–1954 CrossRef CAS;
(c) M. Chen, Z.-J. Wu, J. Song and H.-C. Xu, Angew. Chem., Int. Ed., 2022, 61, e202115954 CrossRef CAS PubMed;
(d) Z.-J. Shen, C. Zhu, X. Zhang, C. Yang, M. Rueping, L. Guo and W. Xia, Angew. Chem., Int. Ed., 2023, 62, e202217244 CrossRef CAS PubMed;
(e) B. Huang, G. Chen, H. Zhang, X. Tang, J. Yuan, C. Lu and J. Wang, Org. Chem. Front., 2023, 10, 3515–3521 RSC;
(f) L. Lan, K. Xu and C.-C. Zeng, Chem. Sci., 2024, 15, 13459–13465 RSC;
(g) L. Zeng, Q. Yang, J. Wang, X. Wang, P. Wang, S. Wang, S. Lv, S. Muhammad, Y. Liu, H. Yi and A. Lei, Science, 2024, 385, 216–223 CrossRef CAS PubMed.
- Selected reviews and articles:
(a) M. D. Kärkäs, Chem. Soc. Rev., 2018, 47, 5786–5865 RSC;
(b) N. Dagar, P. P. Sen and S. R. Roy, ChemSusChem, 2021, 14, 1229–1257 CrossRef CAS PubMed;
(c) Y. Adeli, K. Huang, Y. Liang, Y. Jiang, J. Liu, S. Song, C.-C. Zeng and N. Jiao, ACS Catal., 2019, 9, 2063–2067 CrossRef CAS;
(d) B. Huang, C. Yang, J. Zhou and W. Xia, Chem. Commun., 2020, 56, 5010–5013 RSC;
(e) C. Huang, Z.-Y. Li, J. Song and H.-C. Xu, Angew. Chem., Int. Ed., 2021, 60, 11237–11241 CrossRef CAS PubMed;
(f) J. Hu, R. Ma, J. Hu, X. Liu, X. Liu, H. He, H. Yi and A. Lei, Green Chem., 2024, 26, 4684–4690 RSC;
(g) P. Jiang, C. Liang, T. He, R. Liu, X. Meng, Y. Zheng and S. Huang, Eur. J. Org Chem., 2024, 27, e202400469 CrossRef CAS.
- P. Qian, D. Zhu, X. Wang, Q. Sun and S. Zhang, J. Org. Chem., 2024, 89, 6395–6404 CrossRef CAS PubMed.
- Selected reviews and articles:
(a) A. A. Festa, P. V. Raspertov and L. G. Voskressensky, Adv. Synth. Catal., 2022, 364, 466–486 CrossRef CAS;
(b) J. Wen, W. Shi, F. Zhang, D. Liu, S. Tang, H. Wang, X.-M. Lin and A. Lei, Org. Lett., 2017, 19, 3131–3134 CrossRef CAS PubMed;
(c) Z.-W. Hou, Z.-Y. Mao and H.-C. Xu, Org. Biomol. Chem., 2021, 19, 8789–8793 RSC;
(d) M. Zhang, Z. Luo, X. Tang, L. Yu, J. Pei, J. Wang, C. Lu and B. Huang, Org. Biomol. Chem., 2023, 21, 8918–8923 RSC;
(e) S. Rajput, D. Garg and N. Jain, Adv. Synth. Catal., 2024, 366, 2072–2078 CrossRef CAS.
- C. Pan, B. Huang, W. Hu, X. Feng and J.-T. Yu, J. Org. Chem., 2016, 81, 2087–2093 CrossRef CAS PubMed.
-
(a) A. de la Hoz, Á. Díaz-Ortiz and A. Moreno, Chem. Soc. Rev., 2005, 34, 164–178 RSC;
(b) C. O. Kappe, Chem. Soc. Rev., 2008, 37, 1127–1139 RSC.
- S. Ruan, C. Zhou, L. Li, L. Wang, J. Liu and P. Li, Org. Biomol. Chem., 2022, 20, 3817–3822 RSC.
-
(a) C.-J. Li, Acc. Chem. Res., 2008, 42, 335–344 CrossRef PubMed;
(b) S. A. Girard, T. Knauber and C.-J. Li, Angew. Chem., Int. Ed., 2014, 53, 74–100 CrossRef CAS PubMed;
(c) H. Wang, X. Gao, Z. Lv, T. Abdelilah and A. Lei, Chem. Rev., 2019, 119, 6769–6787 CrossRef CAS PubMed.
- M. Wang, Z. Zhang, C. Xiong, P. Sun and C. Zhou, ChemistrySelect, 2022, 7, e202200816 CrossRef CAS.
- C. Zhou, Y. Liu, Q. Luo, Y. Zhang, J. Zhou, H. Zhang and J. Liu, RSC Adv., 2023, 13, 21231–21235 RSC.
- Selected articles:
(a) H. Sun, B. Huang, R. Lin, C. Yang and W. Xia, Beilstein J. Org. Chem., 2015, 11, 524–529 CrossRef CAS PubMed;
(b) Y. Zhao, B. Huang, C. Yang, B. Li and W. Xia, Synthesis, 2015, 47, 2731–2737 CrossRef CAS;
(c) Y. Zhao, B. Huang, C. Yang and W. Xia, Org. Lett., 2016, 18, 3326–3329 CrossRef CAS PubMed;
(d) M. Lee and M. S. Sanford, Org. Lett., 2017, 19, 572–575 CrossRef CAS PubMed;
(e) Y. Zhao, B. Huang, C. Yang, B. Li, B. Gou and W. Xia, ACS Catal., 2017, 7, 2446–2451 CrossRef CAS;
(f) G. G. D. Ortin and A. G. Salles Jr, Org. Biomol. Chem., 2022, 20, 9292–9297 RSC.
- J. Jin and D. W. C. MacMillan, Angew. Chem., Int. Ed., 2015, 54, 1565–1569 CrossRef CAS PubMed.
- Y. Zhang, K. B. Teuscher and H. Ji, Chem. Sci., 2016, 7, 2111–2118 RSC.
- Selected reviews and articles:
(a) G. E. M. Crisenza, D. Mazzarella and P. Melchiorre, J. Am. Chem. Soc., 2020, 142, 5461–5476 CrossRef CAS PubMed;
(b) Z. Yang, Y. Liu, K. Cao, X. Zhang, H. Jiang and J. Li, Beilstein J. Org. Chem., 2021, 17, 771–799 CrossRef CAS PubMed;
(c) M. Sharique, J. Majhi, R. K. Dhungana, L. M. Kammer, M. Krumb, A. Lipp, E. Romero and G. A. Molander, Chem. Sci., 2022, 13, 5701–5706 RSC;
(d) C. Shi, L. Guo, H. Gao, M. Luo, X. Zhou, C. Yang and W. Xia, Org. Lett., 2023, 25, 7661–7666 CrossRef CAS PubMed;
(e) J. Wang, G. Gao, J. Cheng, J. Li, X. Chen, X. Chen, D. Zhang, H. Li, X. Cai and B. Huang, Green Chem., 2024, 26, 5167–5172 RSC;
(f) Z. Chen, F. Xue, W. Feng, Z. Zhang, B. Wang, Y. Zhang, W. Jin, Y. Xia and C. Liu, Org. Lett., 2024, 26, 7614–7619 CrossRef CAS PubMed.
- S. Devari and B. A. Shah, Chem. Commun., 2016, 52, 1490–1493 RSC.
- J. Kaur, A. Shahin and J. P. Barham, Org. Lett., 2021, 23, 2002–2006 CrossRef CAS PubMed.
- J. Zhou, Q. Ren, N. Xu, C. Wang, S. Song, Z. Chen and J. Li, Green Chem., 2021, 23, 5753–5758 RSC.
- Selected reviews and articles:
(a) Y. Ma, C. Liu, D. Yang, Z. Fang, W. Huang, R. Cheng and J. Ye, Org. Biomol. Chem., 2024 10.1039/D4OB01210K;
(b) Q. Michaudel, D. Thevenet and P. S. Baran, J. Am. Chem. Soc., 2012, 134, 2547–2550 CrossRef CAS PubMed;
(c) G.-X. Li, C. A. Morales-Rivera, F. Gao, Y. Wang, G. He, P. Liu and G. Chen, Chem. Sci., 2017, 8, 7180–7185 RSC;
(d) B. Huang, L. Guo and W. Xia, Green Chem., 2021, 23, 2095–2103 RSC;
(e) Y. Xu, Q. Li, R. Ye, B. Xu and X. Zhou, J. Org. Chem., 2023, 88, 9518–9522 CrossRef CAS PubMed;
(f) S. Schmid, S. Wu, I. Dey, M. Domański, X. Tian and J. P. Barham, ACS Catal., 2024, 14, 9648–9654 CrossRef CAS.
- H. Wu, Z. Xiao, J. Wu, Y. Guo, J.-C. Xiao, C. Liu and Q.-Y. Chen, Angew. Chem., Int. Ed., 2015, 54, 4070–4074 CrossRef CAS PubMed.
- X. Zhang, H. Yang and P. Tang, Org. Lett., 2015, 17, 5828–5831 CrossRef CAS PubMed.
- W.-X. Xu, X.-Q. Dai and J.-Q. Weng, ACS Omega, 2019, 4, 11285–11292 CrossRef CAS PubMed.
- M. Ueda, K. Kamikawa, T. Fukuyama, Y.-T. Wang, Y.-K. Wu and I. Ryu, Angew. Chem., Int. Ed., 2021, 60, 3545–3550 CrossRef CAS PubMed.
- D.-S. Li, T. Liu, Y. Hong, C.-L. Cao, J. Wu and H.-P. Deng, ACS Catal., 2022, 12, 4473–4480 CrossRef CAS.
- B. Quevedo-Flores, I. Bosque and J. C. Gonzalez-Gomez, Org. Lett., 2024, 26, 7447–7451 CrossRef CAS PubMed.
|
This journal is © The Royal Society of Chemistry 2024 |
Click here to see how this site uses Cookies. View our privacy policy here.