Utilizing advancements in chemical sciences for decarbonization: a pathway to sustainable emission and energy reduction
First published on 18th November 2024
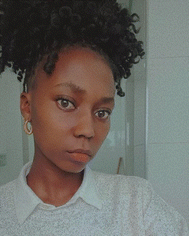 Faith Mwende Johnson | Faith Mwende Johnson was born and raised in Kenya and currently resides in Nairobi. She holds a Bachelor's degree in industrial chemistry from Jomo Kenyatta University of Agriculture and Technology. Faith's work focuses on environmental sustainability and climate change, with substantial research experience in quality assurance, food safety, and environmental analysis. Currently, she is a SHEQ assistant at Ecolab EA, ensuring compliance with safety regulations and supporting environmental management efforts. Faith is passionate about researching new ways to combat climate change and promote sustainable practices. She aims to continue her research through further studies or professional work. |
Introduction
The world is currently facing a crucial challenge in decarbonizing energy production and getting rid of greenhouse gases to counter and mitigate climate change (Shinnar, Ruel and Francesco).1 Here I explain how the chemical sciences play an important role, providing innovative solutions that can transform large scale manufacturing, energy production and agricultural processes.2 By harnessing recent advancements in chemistry, the global community can shift towards sustainable practices such as decreasing reliance on fossil fuels and hence reducing environmental impact.
Implementing green chemistry in industrial production
The traditional processes of manufacturing mostly depend on wasteful practices, use of hazardous chemicals and high-energy reactions which result in depletion of resources and considerable environmental pollution. The principles of green chemistry advocate for designing sustainable manufacturing processes which minimize the generation of waste, employ renewable feed stocks and reduce the consumption of energy.3 The chemical sciences play a vital role in the development of environmentally-friendly alternatives to traditional industrial processes across various sectors, such as material processing, agrochemicals, pharmaceuticals and polymers.4
A key aspect of green chemistry is the design of more sustainable and safer chemical reactions.5 The use of atom-efficient synthesis, solvent-free reactions and catalytic processes maximize product selectivity and yield while minimizing impact on the environment.5 An example is the use of biocatalysts such as microorganisms and enzymes which enable selective and mild transformations in ambient conditions, hence eliminating the use of hazardous reagents and reducing energy requirements.6 Bio-catalytic routes are now used in bio-based polymer manufacturing, the synthesis of pharmaceuticals and in the production of fine chemicals, which in turn offer sustainable alternatives to traditional processes which use hazardous chemicals.7
In addition, green chemistry highlights the use of bio-based materials made from agricultural residues, biomass, or waste streams and renewable feedstocks. Bio-based polymers such as polyhydroxyalkanoates (PHAs), polylactic acid (PLA) and cellulose derivatives, act as eco-friendly options to plastics and fibers based on petroleum. Bio-based polymers and monomers with increased biodegradability and reduced environmental footprint are being produced using chemical processes such as enzymatic conversion, fermentation and chemical depolymerization. Furthermore, use of bio-friendly approaches promotes efficiency of resources and can be circulatory in the manufacturing industry by integrating biochemical, chemical and thermochemical processes to make use of biomass in an array of highly valuable products such as biochemicals, biofuels and bio-plastics.8
Use of catalysis for production of clean energy
Catalysis plays a vital role in various energy conversion processes such as fuel cells,9 hydrogen production10 and carbon capture.11 Catalysts are substances which accelerate chemical reactions and foster the efficient conversion of raw materials into desired products while minimizing waste generation and energy input. For example, during the production of hydrogen using water electrolysis, catalysts enable the splitting of water molecules into oxygen and hydrogen gases. Traditional methods of electrolysis depend on energy-intensive and costly catalysts such as platinum. Catalysts based on transition metals such as cobalt, iron and nickel indicate the potential for improving efficiency and lowering costs in the production of hydrogen.12
Furthermore, catalysts are vital in fuel cell technologies. These technologies directly convert chemical energy into electrical energy without the use of combustion. Solid oxide fuel cells (SOFCs)13 and proton exchange membrane fuel cells (PEMFCs)14 depend on catalysts to carry out electrochemical reactions at electrodes, thereby facilitating the conversion of fuels into electricity. Recent advances in catalyst design aim to improve the durability and selectivity of catalysts used in fuel cells, reducing costs and improving overall performance.
Another application of catalysis that is showing potential is in carbon capture and utilization (CCU) technologies.15 CO2 can be selectively captured directly from the atmosphere or from industrial flue gases, by employing catalysts such as metal–organic frameworks or amine-functionalized materials. Successive chemical reactions enable conversion of the captured CO2 into useful products such as chemicals, fuels and building materials. For example, captured CO2 can be converted into methanol using catalysts such as copper–zinc oxide–aluminum oxide (Cu–ZnO–Al2O3).16 Such CCU processes contribute to the development of a circular carbon economy by recycling CO2 as a feedstock for various industries, mitigating the emission of greenhouse gases.17
Carbon capture and utilization (CCU)
The continuous rise of CO2 levels in the atmosphere because of anthropogenic activities poses a substantial threat to ecosystem health and climate stability. Use of carbon capture and utilization (CCU) technologies provide potential strategies for alleviating CO2 emissions from industrial processes and tackling the growing demand for carbon-neutral material and fuels.8 Chemical sciences play a vital role in developing cost effective and efficient CCU processes through process optimization, innovative material design and life cycle analysis.
One method in carbon capture involves chemical absorption.18 In this approach, CO2 is absorbed by sorbents or solvents in secondary combustion flue gases.18 Amine based solvents can be regenerated for repeated use and exhibit high CO2 absorption. These amine-based solvents include amine-functionalized porous materials and monoethanolamine (MEA). Ongoing research focuses on improving the stability, selectivity and energy efficiency of sorbents used to capture CO2 through process integration, surface modification and material synthesis. In addition, recent emerging technologies for carbon capture such as cryogenic distillation19 and membrane-based separation, provide alternative ways to capture CO2 with reduced environmental and energy footprints.
After CO2 is captured, it can be transformed into useful products by utilizing chemical processes such as hydrogenation, carbonation and electrochemical reduction. An example is that CO2 can react with alkaline materials such as magnesium silicate or calcium oxide producing stable carbonate minerals which can be used for construction or geological sequestration.20 In addition, the hydrogenation of CO2 catalyzed by transition metal catalysts gives alcohols, hydrocarbons and other chemical feedstocks providing a sustainable pathway for production of carbon neutral fuel. Also, reduction of CO2 by electrochemical processes using renewable sources of electricity makes it possible to synthesize value-added chemicals such as methane, formic acid or ethylene, with high energy efficiency and selectivity.21 Ongoing research in CCU is exploring reaction pathways, novel catalysts and strategies for process integration, to increase the environmental merits of carbon utilization technologies and economic viability.
Storage and conversion of renewable energy
Renewable sources of energy such as wind power and solar are known to offer clean and abundant alternatives to fossil fuels.22 However, their continued use poses challenges regarding supply reliability and grid stability.22 Chemical sciences play an important role by developing conversion technologies and efficient energy storage to curb these challenges.
Batteries are vital for storage and delivery of electricity from renewable sources which in turn enables management of loads and balance. The battery market has been dominated by lithium-ion batteries (LIBs) because they have a long-life cycle and a high energy density.23 However, due to safety concerns and the limited availability of lithium, research efforts have been focused on alternatives. Some potential sustainable and low-cost alternatives to LIBs being explored, include magnesium-ion batteries (MIBs),24 potassium-ion batteries (PIBs) and sodium-ion batteries (SIBs). Furthermore, advances in battery technologies such as flow batteries provide better scalability, safety and long-life cycle, paving the way for the wide use of renewable systems for energy.
Apart from batteries, chemical processes are being used for storage and conversion in fuel-based systems. For instance, hydrogen gas produced through biomass conversion or electrolysis from renewable sources, can be used as a carrier of clean energy with many applications in transportation, fuel cells and industrial processes. Chemical means of storage such as reversible chemical reactions25 and hydrogenation of organic compounds, make it possible to store hydrogen safely and efficiently for long periods. Ongoing research efforts are looking to develop advanced materials for storage of high-density hydrogen with better thermodynamics and improved kinetics. These advanced materials include chemical hydrides, metal hydrides and porous frameworks.
Technologies for conversion of solar energies such as solar thermal systems and photovoltaics utilize sunlight to produce heat or electricity. Photovoltaic devices utilize inorganic, organic and hybrid materials to harness sunlight which is then converted into electrical energy. Promising alternatives to the outdated solar cells based on silicon include perovskite solar cells which have low cost of fabrication, high efficiency and tunable optoelectronic properties. Optimization of the structure, stability and composition of perovskite materials is a crucial role played by chemical sciences for practical application of solar cells.
Conclusions
The chemical sciences provide diverse opportunities to reduce the emission of greenhouse gases from agricultural processes and large-scale manufacturing and to decarbonize the production of energy. Through technological advancements, innovative research and the adoption of sustainable practices, it is possible to build a more environmentally resilient future and eliminate climate change. It is essential for policymakers, communities, industries and scientists to collaborate to make it possible to achieve a low-carbon economy and to ensure that the planet is sustained for future generations. By making use of the transformative power of chemistry, it is possible to address the challenges of environmental stewardship and energy sustainability, making way for a more prosperous and greener world.
Conflicts of interest
AI technologies were not used in the preparation of my essay.
References
-
J. J. Soon, K. S. Low and S. T. Goh, Multi-Dimension Diode Photovoltaic (PV) Model for Different PV Cell Technologies, IEEE International Symposium on Industrial Electronics, 2014, pp. 2496–2501 DOI:10.1109/ISIE.2014.6865012.
- I. Butnar, O. Broad, B. S. Rodriguez and P. E. Dodds, The Role of Bioenergy for Global Deep Decarbonization: CO2 Removal or Low-Carbon Energy?, GCB Bioenergy, 2020, 12(3), 198–212, DOI:10.1111/gcbb.12666.
- T. L. Chen, H. Kim, S. Y. Pan, P. C. Tseng and Y. P. Lin,
et al.. “Implementation of Green Chemistry Principles in Circular Economy System towards Sustainable Development Goals: Challenges and Perspectives, Sci. Total Environ., 2020, 716(1), 136998, DOI:10.1016/j.scitotenv.2020.136998.
-
S. A. Shafiee, Investigating the Study of Green Chemistry and Its Achievements in Protecting the Environment and Preventing Pollution, 2018, 6, 1, 36–40.
- W. Abdussalam-mohammed, A. Qasem and A. O. Errayes, Green Chemistry: Principles, Applications, and Disadvantages, Chem. Methodol., 2020, 4(4), 408–423 CrossRef CAS.
- G. Antranikian, C. E. Vorgias and C. Bertoldo, Extreme Environments as a Resource for Microorganisms and Novel Biocatalysts, Adv. Biochem. Eng./Biotechnol., 2005, 96, 219–262, DOI:10.1007/b135786.
- R. de Regil and G. Sandoval, Biocatalysis for Biobased Chemicals, Biomolecules, 2013, 3(4), 812–847, DOI:10.3390/biom3040812.
- P. C. Sabapathy, S. Devaraj, K. Meixner, P. Anburajan and P. Kathirvel,
et al.. “Recent Developments in Polyhydroxyalkanoates (PHAs) Production – A Review, Bioresour. Technol., 2020, 306, 123132, DOI:10.1016/j.biortech.2020.123132.
- T. R. Ralph and M. P. Hogarth, Catalysis for Low Temperature Fuel Cells, Platinum Met. Rev., 2002, 46(3), 117–135 CrossRef CAS.
- B. Xie, R. J. Wong, T. H. Tan, M. Higham and E. K. Gibson,
et al.. “Synergistic Ultraviolet and Visible Light Photo-Activation Enables Intensified Low-Temperature Methanol Synthesis over Copper/Zinc Oxide/Alumina, Nat. Commun., 2020, 11(1) DOI:10.1038/s41467-020-15445-z.
- H. J. Kulik, S. E. Wong and S. E. Baker,
et al.. “Developing an Approach for First-Principles Catalyst Design: Application to Carbon-Capture Catalysis, Acta Crystallogr., Sect. C: Struct. Chem., 2014, 70(2), 123–131, DOI:10.1107/S2053229613027666.
- B. H. Liu, P. L. Zhou and S. Suda, Nickel- and Cobalt-Based Catalysts for Hydrogen Generation by Hydrolysis of Borohydride, J. Alloys Compd., 2006, 415(1–2), 288–293, DOI:10.1016/j.jallcom.2005.08.019.
- S. Park, R. J. Gorte and J. M. Vohs, Applications of Heterogeneous Catalysis in the Direct Oxidation of Hydrocarbons in a Solid-Oxide Fuel Cell, Appl. Catal., A, 2000, 200(1), 55–61, DOI:10.1016/S0926-860X(00)00650-5.
- S. Tepavcevic, Y. Liu, D. Zhou, B. Lai and J. Maser,
et al.. “Nanostructured Layered Cathode for Rechargeable Mg-Ion Batteries, ACS Nano, 2015, 9(8), 8194–8205, DOI:10.1021/acsnano.5b02450.
- M. A. Sabri, S. A. Jitan, D. Bahamon, L. F. Vega and G. Palmisano, Current and Future Perspectives on Catalytic-Based Integrated Carbon Capture and Utilization, Sci. Total Environ., 2021, 790, 148081, DOI:10.1016/j.scitotenv.2021.148081.
- P. Gabrielli, M. Gazzani and M. Mazzotti, The Role of Carbon Capture and Utilization, Carbon Capture and Storage, and Biomass to Enable a Net-Zero-CO2 Emissions Chemical Industry, Ind. Eng. Chem. Res., 2020, 59(15), 7033–7045, DOI:10.1021/acs.iecr.9b06579.
- S. Mona, S. K. Malyan, N. Saini, B. Deepak, A. Pugazhendhi and S. S Kumar, Towards sustainable agriculture with carbon sequestration, and greenhouse gas mitigation using algal biochar, Chemosphere, 2021, 275, 129856, DOI:10.1016/j.chemosphere.2021.129856.
- B. Roffel, B. H. L. Betlem and J. A. F. De Ruijter, First Principles Dynamic Modeling and Multivariable Control of a Cryogenic Distillation Process, Comput. Chem. Eng., 2000, 24(1), 111–123, DOI:10.1016/S0098-1354(00)00313-6.
- A. Scott, C. Oze, V. Shah, N. Yang and B. Shanks,
et al.. “Transformation of Abundant Magnesium Silicate Minerals for Enhanced CO2 Sequestration, Commun. Earth Environ., 2021, 2(1), 1–6, DOI:10.1038/s43247-021-00099-6.
- Xu Lu, D. Y. C. Leung, H. Wang, M. K. H. Leung and J. Xuan, Electrochemical Reduction of Carbon Dioxide to Formic Acid, ChemElectroChem, 2014, 1(5), 836–849, DOI:10.1002/celc.201300206.
- B. Holtom, Accepted Version. 4e, J. Nurs. Adm., 2004, 34(5), 216–227, DOI:10.1097/00005110-200405000-00005.
- D. Deng, Li-Ion Batteries: Basics, Progress, and Challenges, Energy sci. eng., 2015, 3(5), 385–418, DOI:10.1002/ese3.95.
- S. Wang, A. Lu and C. J. Zhong, Hydrogen Production from Water Electrolysis: Role of Catalysts, Nano Convergence, 2021, 8(1) DOI:10.1186/s40580-021-00254-x.
- W. H. Bernskoetter and N. Hazari, Reversible Hydrogenation of Carbon Dioxide to Formic Acid and Methanol: Lewis Acid Enhancement of Base Metal Catalysts, Acc. Chem. Res., 2017, 50(4), 1049–1058, DOI:10.1021/acs.accounts.7b00039.
- S. A. Kalogirou, S. Karellas, V. Badescu and K. Braimakis, Exergy Analysis on Solar Thermal Systems: A Better Understanding of Their Sustainability, Renew. Energy, 2016, 85, 1328–1333, DOI:10.1016/j.renene.2015.05.037.
|
This journal is © The Royal Society of Chemistry 2024 |
Click here to see how this site uses Cookies. View our privacy policy here.