Adjustable oil adhesion on superamphiphobic copper surfaces for controlled oil droplet transport†
Received
25th September 2023
, Accepted 22nd November 2023
First published on 22nd November 2023
Abstract
The desirability of superamphiphobic surfaces, which possess both superhydrophobic and superoleophobic properties, for practical applications stems from their enhanced adaptability in oil-contaminated environments when compared to superhydrophobic surfaces. However, achieving superamphiphobic surfaces with adjustable oil adhesion through a simple, cost-effective, and reproducible fabrication process has proven challenging for researchers. This study presents an approach that combines laser etching with a chemical method to obtain superamphiphobic copper surfaces which demonstrated UV and corrosion resistance, as well as adjustable oil adhesion. The modified copper surface shows superamphiphobic properties, indicated by contact angles greater than 150° for water, colza oil, n-hexadecane, and crude oil. Significantly, the presence of concave-down micro/nano reentrant structures is found to be crucial in generating superamphiphobic properties. Additionally, the surface exhibits outstanding resistance to strong acids, strong alkalis, and saturated sodium chloride solutions. Even following a 48-hour exposure to UV irradiation, the contact angles for water and oil remain above 150°, demonstrating sustained UV resistance of the surface. Additionally, when in contact with water and crude oil, the surface exhibits outstanding anti-fouling and self-cleaning capabilities. Notably, the dimensions of the laser-etched grooves on the modified copper surface are adjusted, enabling customizable oil adhesion and controlled oil droplet transportation.
1. Introduction
Copper is widely utilized in industries such as aerospace, railways, shipbuilding, and automobiles due to its excellent ductility, conductivity, and thermal properties. However, exposure to high humidity, elevated temperatures, or oil-contaminated environments can lead to pollution and corrosion, significantly impacting its longevity.1 An approach to address this challenge involves imparting special wetting properties to copper surfaces, a promising area that has garnered continuous attention from researchers exploring functional metals with specialized wettability.2,3 The potential applications of such modifications include self-cleaning,4 anti-ice,5 anti-corrosion,6 oil–water separation,7 anti-fouling,8 and more. However, superhydrophobic surfaces (SHBSs) are vulnerable to oil and organic matter contamination.9,10 Similarly, problems associated with lubricant depletion and replenishment are encountered with slippery liquid-infused porous surfaces (SLIPSs).11,12 These issues seriously impede its application in practice. Compared to the first two, superamphiphobic surfaces (SAPSs) are not only unaffected by lubricant loss, but also repel a wider range of fluids than SHBSs, which is a natural advantage from this point of view. Therefore, SAPSs may have a wider range of potential applications than SHBSs and SLIPSs, including in crude oil transfer, fluid power systems, anti-fouling and anti-crawling materials, etc.13
There are three primary challenges when designing SAPSs for the shift from laboratory creation to practical use. Designing SAPSs is a challenging and intricate task due to the similarity in surface tension between oil and commonly used low-energy materials. The texture structure of the surface is essential for the design of SAPSs.14,15 In addition to relying on a combination of rough structure and low surface energy chemistry,16,17 the design of SAPSs requires the introduction of structures possessing reentrant curvature,18–22 such as overhang,23 mushroom-like,24,25 inverted trapezoidal16 and nano-hoodoo structures.26
The reentrant structure creates air pockets within the surface microstructure, facilitating the formation of a three-phase interface of oil droplets and contributing to the surface's oil-repellent properties. Furthermore, the Laplace pressure generated by the reentrant micro/nano structures counteracts the gravitational force of the water/oil droplets on the solid–liquid–gas three-phase contact surfaces, resulting in an upward synergistic force that minimizes the solid–liquid contact area. This effectively prevents oil droplets from infiltrating the texture structure, and maintaining the entire system in the Cassie–Baxter state. This understanding paves the way for achieving superamphiphobicity on hydrophilic surfaces.
Secondly, achieving both superamphiphobicity and durability poses a significant challenge, as the fine reentrant texture structure, essential for reducing the solid–liquid contact area, is more susceptible to wear under mechanical loading. Thus, maintaining long-term superamphiphobicity becomes a complex task. Previous strategies aimed at enhancing the mechanical durability of special wettable surfaces have included the development of multi-scale wear-resistant structures, self-similar structures, incorporation of self-repairing properties into materials, and the use of organic/inorganic adhesives to strengthen the substrate–coating adhesion.27–29 While these approaches have temporarily improved surface durability, a fundamental and permanent solution remains elusive. Notably, in 2000, Wang et al. proposed a novel concept for constructing robust SHBSs using a dual-length-scale approach. By fabricating SHBSs with inverted pyramidal armor structures on silicon substrates via photolithography, they demonstrated that the pyramidal microstructures on the surface engendered protection for the embedded fluorinated silica nanostructures, marking a significant advancement in the pursuit of durable SHBSs.30
Additionally, the scalability of manufacturing and high reproducibility of reentrant micro/nano structures on metal surfaces present competing factors. Although SAP copper surfaces have garnered significant attention in recent years, the preparation of metallic surfaces with excellent superamphiphobicity still remains complex, expensive, and difficult to reproduce. For instance, in 2012, Zhu et al.31 used a two-step solution immersion method to acquire reentrant structures of CuO micro flowers positioned on nanorods. Later, in 2018, Wen et al.1 utilized a three-step solution immersion method to obtain SAP copper surfaces featuring CuO/Ag reentrant micro/nano structures. More recently, in 2021, Zhu et al.32 achieved superamphiphobic sheets with CuO micron flowers and nano pins through molding, oxidation and fluoride modifications. Although the fabrication of SAP copper surfaces on a large scale has been facilitated, the reproduction of the desired superamphiphobicity, due to the relatively random nature of the produced reentrant structures, may necessitate multiple attempts in practical implementation. Chu et al.33 introduced the use of a femtosecond laser Bessel beam to induce controlled adhesion on a polytetrafluoroethylene surface, while Chao and colleagues34 utilized nano-laser writing (LDW) to produce microcone arrays on the surface of copper. Subsequent application of a chemical solution-based technique led to the formation of long hooked nano-grass structures on the microcones. The LDW technique enabled adjustable manipulation of the grid spacing, providing customizable oil adhesion properties. Comparative analysis of the superoleophobic properties and applications of superamphiphobic copper and aluminum surfaces in recent years has been conducted, and detailed information can be found in Table S1.†
Currently, there is a lack of comprehensive research on controllable preparation techniques for SAPSs, making consistent preparation challenging due to the absence of specific control parameters. Laser marking offers a degree of controllability and reproducibility to certain special wettable metal surfaces. The micron armor structure demonstrates exceptional resistance to both vertical pressure and shear forces. Drawing inspiration from the concept of microarmor, this study explores the design of a down-concave reentrant hierarchical micro/nano structure on copper surfaces to improve superamphiphobicity and stability at both micro and nanometer scales. Liquids with varying surface tensions remain spherical on the surface and can be easily rolled off. The attained superamphiphobicity also heightens the material's corrosion resistance, resulting in a more pronounced corrosion potential compared to unaltered and superhydrophobic copper sheets. More importantly, the copper sheet is laser-etched with a varying pattern to create a concave structure of different sizes, enabling adjustable oil adhesion for controlled oil droplet transport.
2. Experimental section
2.1. Materials
All chemicals and solvents were used without further purification. Sodium hydroxide (NaOH, 95%), ammonium persulfate ((NH4)2S2O8, AR, 98.5%) and n-hexadecane (AR, 98%, 27.5 mN m−1) were purchased from McLean Biochemistry Co. 1H,1H,2H,2H-Perfluorodecanethiol (PFDT, 98%) was purchased from Sane Chemical Technology Shanghai Co. Silver nitrate (AR, 99.8%) and rhodamine (95%) were obtained from Aladdin Holdings Group Co., Ltd. Glycerol (AR, ≥99.0%, 64 mN m−1), glycol (AR, ≥99.5%, 48.4 mN m−1) and methylene blue (AR) were obtained from China National Pharmaceutical Group Corporation. Colza oil (∼33 mN m−1), pure milk, tea, coffee, crude oil and copper flakes were purchased from local markets.
2.2. Preparation of concave-down micro structures by laser labeling
The copper sheets underwent an ultrasonic cleaning process three times with ethanol and deionized water, respectively. Subsequently, the laser marking machine (Jw-F) was used to etch square concave microstructures of various sizes on the cleaned copper sheets. The length and width of the grooves measure between 0.01 and 0.1 millimeters. The markings were made on the copper sheets measuring 1.5 cm in length and 1.5 cm width, with a thickness of 0.02 mm, in order to obtain the desired concave microstructures. The laser scanning path was precisely controlled using Auto Drawing software. Notably, the size of the recesses created during the drawing process ranged from 0.01 to 0.1 mm, with a 0.01 mm spacing between recesses, and the etching process was carried out 10 times. For specific setup parameters, please refer to Table S2.†
2.3. Fabrication of CuO lamellar and flower-like structures
The copper sheets containing grooves were immersed in 20 mL of HCl solution (1 M) for 20 s to eliminate the oxide layer. Afterward, they were dried and subsequently submerged in 20 mL aqueous solution consisting of NaOH (1 mol L−1) and (NH4)2S2O8 (0.05 mol L−1). This immersion process lasted for 30 min, conducted in a water bath set at 60 °C. Following oxidation, the copper sheets were removed from the solution, thoroughly rinsed with water, and finally dried at 60 °C.
2.4. Fabrication of CuO/Ag micro/nano structures
The modified copper sheets were immersed in 20 mL AgNO3 solution (1 g L−1) for a duration of 30 min, carried out at room temperature. Subsequently, they were dried at 60 °C for 30 min. To eliminate any traces of residual reagents, the dried copper sheets underwent a thorough washing procedure with deionized water, followed by a drying process at room temperature. This meticulous procedure resulted in the creation of copper sheets possessing a hierarchical structure of CuO/Ag.
2.5. Fluoridation
Copper sheets featuring concave-down micro/nano structures were immersed in an ethanol solution of PFDT with a volume fraction of 5/1000 for a duration of 30 min, conducted at room temperature. After that, the copper sheets were cleaned with ethanol and dried at room temperature to obtain super-duplexed copper surfaces (F-CuO/Ag-0.01–0.1). For comparison, superhydrophobic copper surfaces (F-CuO) were obtained by directly immersing copper sheets with micrometer grooves of CuO lamellar structures into an ethanol solution of PFDT for 30 min prior to immersion in AgNO3 solution.
2.6. Characterization
The surface morphology was characterized using field emission scanning electron microscopy (FESEM, Zeiss Sigma 500). Elemental distribution on the superamphiphobic copper surface was determined using energy dispersive spectroscopy (EDS, JSM-5600LV). The crystal structures of Cu, CuO and Ag were analyzed by X-ray diffraction (XRD, D8 Advance) with a scan rate of 0.5° min−1 and 2θ from 5° to 80°. Fourier transform infrared spectroscopy (FTIR, Nicolet iS50) was employed to analyze the changes in chemical composition. The surface chemical structure was characterized using X-ray photoelectron spectroscopy (XPS, Escalab 250Xi; Thermo Scientific). The water/oil contact and slide angle (5 μL droplet volume) on the copper surface was measured using a JC2000D goniometer (Zhong Chen Digital Equipment Co. Ltd, Shanghai, China). To evaluate the corrosion protection function, polarization measurements were conducted in a 3.5 wt% NaCl aqueous solution at room temperature via a three-electrode system. In this system, the sample to be tested served as the working electrode, a platinum sheet as the counter electrode, and a saturated calomel electrode as the reference electrode. The exposed area of the working electrode was 1.5 cm2.
3. Results and discussion
3.1. Fabrication and characterization of superamphiphobic copper surfaces
The preparation process of SAPSs is shown in Fig. 1. The micrometer groove structure was first obtained by etching an array of grooves (G) on the cleaned copper sheet surface using a laser marking machine. The size of the grooves (10 to 100 μm) was adjusted by designing the patterning parameters using Auto CAD drawing software, followed by alkali-assisted oxidation, displacement and fluorination reactions using the solution immersion method. SEM characterization of the samples obtained during the preparation of the SAP surface shows a gradual increase in roughness when comparing Fig. 2a–d and g, h.
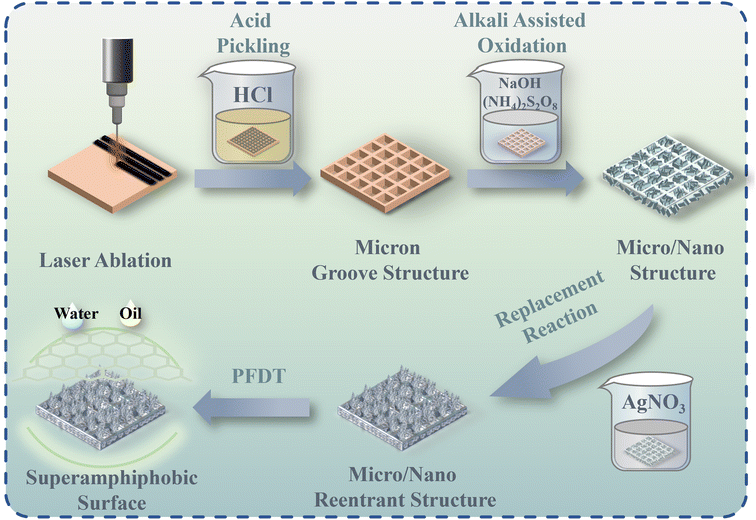 |
| Fig. 1 Preparation process of superamphiphobic copper sheets with concave-down reentrant micro/nano structures. | |
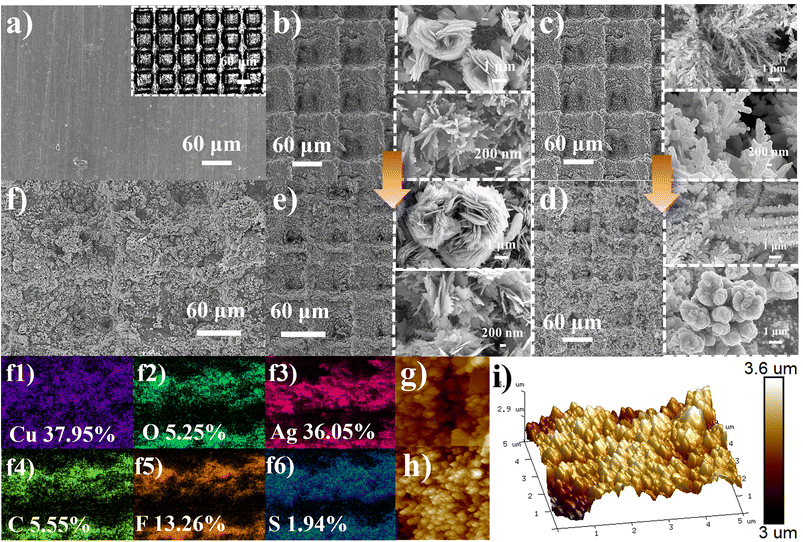 |
| Fig. 2 (a) SEM image of the pristine copper sheet. The upper right corner is a light microscope photograph after laser marking a 60 μm notch on a copper sheet; (b) SEM image after alkali-assisted oxidation reaction, with magnifications of 10k and 20k times in the upper right and lower right corners, respectively; (c) SEM image after the substitution reaction, with magnifications of 10k and 20k times in the upper right and lower right corners, respectively; (d) F-CuO/Ag-0.06, upper-right and lower-right corners are magnifications of 10k and 20k times, respectively; (e) SEM image of F-CuO, upper-right and lower-right corners are magnifications of 10k and 20k times, respectively; (f) EDS image of F-CuO/Ag-0.06; (g) and (h) AFM 2D height images of F-CuO-0.06 and F-CuO/Ag-0.06; (i) AFM 3D height of F-CuO/Ag-0.06 images. | |
After alkali-assisted oxidation, lamellar and flower-like CuO structures grew both inside and outside the grooves of the copper sheets (Fig. 2b). Following the replacement reaction with AgNO3 solution, some dendritic and coral-like clusters of Ag nanoparticles grew on the CuO (Fig. 2c). As a result of the modification by PFDT, the lamellar structure of CuO was thickened, and the dendritic and coral-like Ag nanoparticle agglomerates were transformed into multiscale micro/nano reentrant structures. Eventually, the grooves were uniformly and densely covered with micro/nano structures both inside and outside (Fig. 2d). For comparison, the grooved CuO and ungrooved CuO and CuO/Ag structured surfaces were also modified with PFDT to obtain F-CuO-0.06 (Fig. 2e), F-CuO and F-CuO/Ag surfaces, respectively. The EDS images of the superamphiphobic copper surfaces show that the elements Cu, O, Ag, C, F and S are uniformly distributed on the surfaces (Fig. 2f). From the 3D AFM image (Fig. 2i), it can be observed more intuitively that the micro/nano protrusions generated by fluorination increase the surface roughness.
To determine the chemical composition of the modified samples, the FTIR, XRD and XPS plots of the samples were analyzed. The FTIR maps of the F-CuO and F-CuO/Ag surfaces revealed the generation of three new absorption peaks at 1527, 1209 and 1151 cm−1, attributed to the stretching vibrations of the –CF3, –CF2– and C–S groups, respectively (Fig. 3a). The XRD patterns represented the crystal structures of Cu, CuO and Ag (Fig. 3b). In the pristine copper sheet's XRD spectrum, the crystal planes (111), (200) and (220) were consistent with those of Cu. After oxidation, two new crystal planes (−111) and (111) emerged, corresponding to the crystal planes of CuO, indicating the formation of oxidized lamellar and floral structures as CuO. Furthermore, the appearance of three new crystal planes (111), (200) and (220) in the substitution reaction corroborated the crystal planes of Ag, suggesting the presence of dendritic and coral-like structures formed by silver nanoparticle agglomerates. The successful generation of CuO and Ag micro-nanoparticles was also evident from the fine spectra of Cu 2p, C 1s and Ag 3d (Fig. 3d and e). The XPS full spectrum (Fig. 3c) further confirmed the presence of CuO and Ag, as well as the successful grafting of PFDT. Taken together, these results provide compelling evidence for the successful modification of the F-CuO/Ag superamphiphobic copper surface.
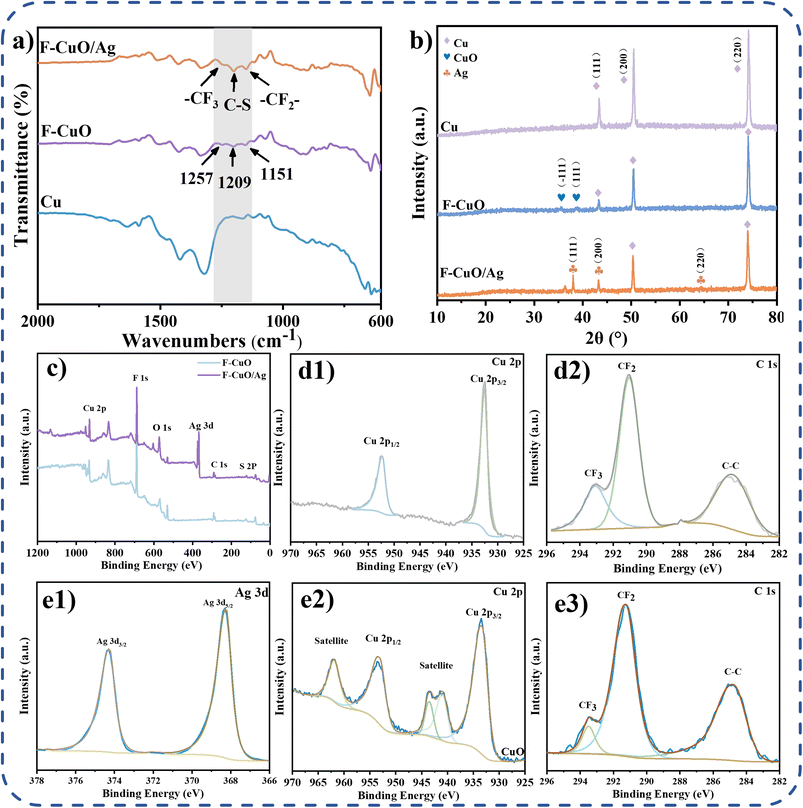 |
| Fig. 3 (a) FTIR profiles of Cu, F-CuO and F-CuO/Ag surfaces; (b) XRD profiles of Cu, F-CuO and F-CuO/Ag surfaces; (c) XPS full-spectrum profiles of F-CuO and F-CuO/Ag surfaces; (d) split-peak plot of F-CuO; (e) split-peak plot of F-CuO/Ag. | |
3.2. Surface wettability of the modified copper surfaces
The contact angle measurements to water, ethanediol, glycerol and colza oil were carried out for the F-CuO without grooves, F-CuO/Ag and F-CuO/Ag-0.01–0.1 samples, respectively (Fig. 4). The comparison reveals that F-CuO is only highly hydrophobic (HHB) and highly oleophobic (HOB), F-CuO/Ag is SHB and highly oleophobic (HOB), and F-CuO/Ag-0.01–0.1 is SAP.
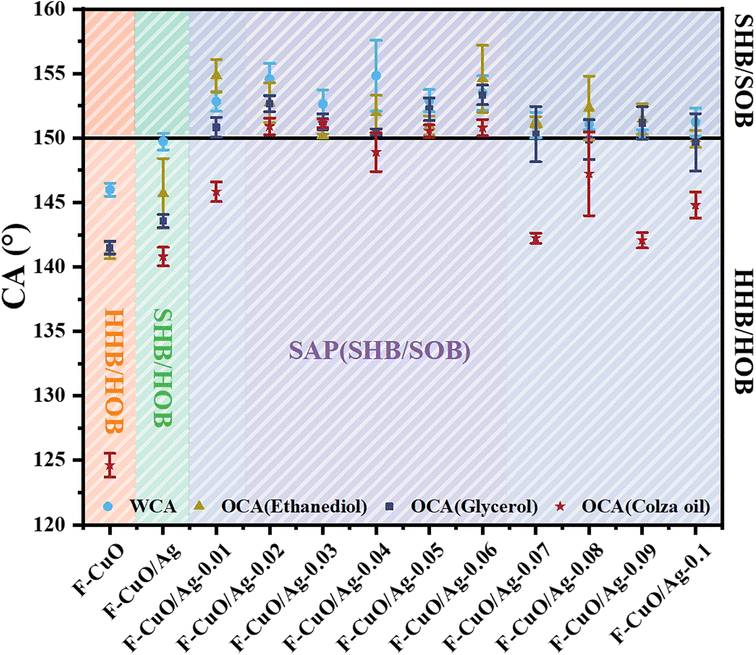 |
| Fig. 4 Contact angles of F-CuO, F-CuO/Ag and F-CuO/Ag-0.01–0.1 against water, ethanediol, glycerol and colza oil. | |
Comparison of the F-CuO and F-CuO/Ag surfaces shows that both ammonia-base etching and substitution reactions increase the surface roughness on the nanometer scale, respectively, and the fluorination reaction reduces the surface chemical energy, enabling the copper surface to produce superhydrophobicity and high oleophobicity. Comparison of F-CuO/Ag and F-CuO/Ag-0.1–0.01 surfaces shows that the roughness provided by laser etching on the micrometer scale plays an important role in producing superoleophobicity. In addition, comparing the SAP copper surfaces with different groove sizes, it can be found that the samples with groove sizes of 20–60 μm have the lowest surface energy, and the contact angles are all greater than 150° for colza oil, while the other samples were only superoleophobic to ethanediol and glycerol, with an OCAcolza oil of about 140°. This indicates that the solid–liquid contact area size can be changed by simply adjusting the etching pattern. The surface energy of the sample is lowest within a certain solid–liquid contact range, when the superamphiphobicity is optimal. Therefore, laser etching imparts adjustable oil adhesion to copper surfaces.
The Wenzel and Cassie–Baxter models are used to explain the effect of roughness on the apparent contact angle of a liquid on a rough surface, respectively. The Wenzel model demonstrates the wetting state of the liquid penetrating within the rough surface, and the Cassie model demonstrates the presence of air between the solid surface and the liquid, forming a solid–liquid–gas three-phase composite interface. When water/oil droplets are located on the SAP copper surface, the wetting behavior is described by the Cassie–Baxter equation.35
cos θr = fs cos θs + fg cos θgwhere |
fs is the solid contact area fraction and
fg is the gas contact area fraction (
fs +
fg = 1). Then, the Cassie–Baxter equation can be transformed as follows:
cos θr = −1 + fs(cos θs + 1) |
where
θs is Young's contact angle,
θr is the apparent contact angle, and
fs is the solid contact area fraction. The surface roughness coefficient
rf (
rf > 1) of the wetted area is introduced to account for localized surfaces, where
f is the fraction of the projected area of the solid surface wetted by water (
fs = 1 +
f).
SEM and AFM showed that rf on the copper surface can indeed be increased by alkali-assisted oxidation and displacement reactions. The fluorination reaction produced a high oleophobic sample with OCAcolza oil = 140.8 ± 0.7°. Laser labeling further reduces the solid–liquid contact area and raises the rf to a higher level, and the sample produces superoleophobicity,36,37 OCAcolza oil = 151.3 ± 0.3°.
It has been shown that structures with reentrant curvature favor very high θr and can establish stable composite interfaces with various liquids, including organic liquids such as cetane.38,39 For example, overhang structures, mushroom-like structures, inverted trapezoidal structures, etc. are crucial for the formation of composite solid–liquid–gas interfaces with oil droplets as well as when the oil droplets are placed on such reentrant structures. The Laplace pressure (ΔP) generated by the reentrant structure on the three-phase contact surface is able to balance the gravitational force of the water/oil droplet at the solid–liquid–gas three-phase contact point, generating an upward combined force (Fn).40
ΔP = P − P0 = −γ cos(θ + α)/R − h tan α |
where
γ is the surface tension of the liquid,
θ is Young's contact angle,
α is the tilt angle,
R is the half-width between the bottom edges of two neighboring sloping walls, and
P is the pressure on the liquid side of the curved moon face. In addition, Yong
et al.16 argued that when the local geometric texture angle (
ψ) is satisfied: 90° >
θ >
ψ. The oil droplets are able to generate an upward
Fn at the three-phase contact point.
On the one hand, the fluorination treatment produces multiscale micro/nanostructures with low surface energy and high roughness, increasing θr. On the other hand, this reentrant topography provides the ψ needed to generate superamphiphobicity. On the surface with reentrant micro/nano topography, Fig. 5 shows that the ψ values of the surface are less than 90°. The F-CuO/Ag-0.06 sample was selected for superamphiphobicity testing (Fig. 6). This structure results in a system with Fn upwards, minimizing the solid–liquid contact area. Thus, organic liquids such as n-hexadecane and crude oil can be effectively prevented from penetrating into the textured structure (Fig. 5a), thus maintaining the whole system in Cassie–Baxter state.
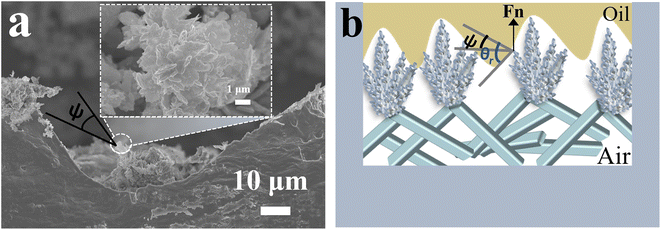 |
| Fig. 5 (a) Cross-sectional SEM image of F-CuO/Ag-0.06, (b) schematic structure of F-CuO/Ag-0.06. | |
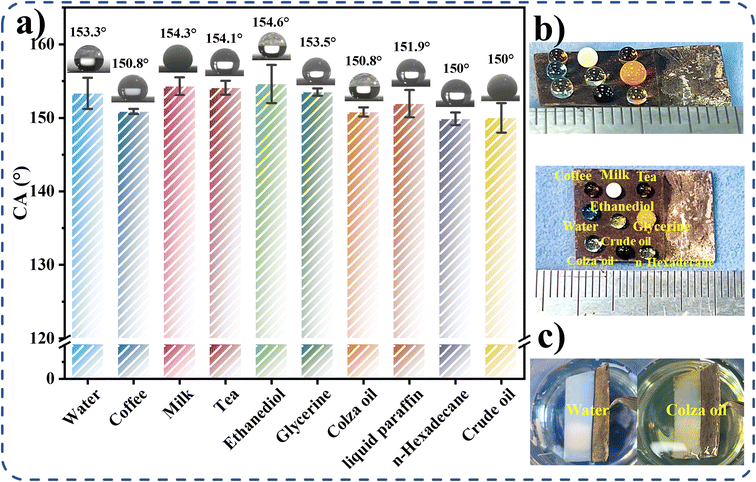 |
| Fig. 6 (a) Contact angle magnitudes and optical photographs of F-CuO/Ag-0.06 for ten liquids; (b) optical photographs of nine liquids that remain spherical on the F-CuO/Ag-0.06 surface; (c) silver mirror phenomenon produced by the F-CuO/Ag-0.06 surface in water and colza oil, respectively. | |
The contact angles of water, edible oil, n-hexadecane, and crude oil on the F-CuO/Ag-0.06 sample, as indicated in Fig. 6a, are 153.3 ± 2.1°, 150.8 ± 0.6°, 149.9 ± 0.9°, and 150 ± 2°, respectively. Fig. 6b shows the modified copper sheet allowing n-hexadecane with very low surface tension and highly adherent crude oil to stand on its surface in a spherical shape. Fig. 6c shows the silver mirror phenomenon produced by the F-CuO/Ag-0.06 surface in water and canola oil, illustrating the presence of an air layer between the rough textured structure and the liquid, which acts as a barrier impeding contact at the solid–liquid interface. As a result, the prepared F-CuO/Ag-0.06 surface provides the desired surface texture, can keep the surface unwetted in contact with water/oil, and has ultra-low surface tension (γ < 25.7 mN m−1) and excellent superamphiphobicity.
3.3. Stability and corrosion resistance of the superamphiphobic copper sheet
There is a cushion of air in the reentrant micro/nano textured structure that insulates the substrate from liquids when the surface comes into contact with it. Therefore, the SAP surface has good corrosion resistance in addition to superhydrophobicity and superoleophobicity. In order to demonstrate the chemical stability, UV resistance and corrosion resistance of the F-CuO/Ag-0.06 surface some simple studies were carried out.
Aqueous solutions with pH = 1–14 were configured to test the wettability of SAP copper surfaces to droplets of different pH values. Fig. 7a shows that the contact angles of the F-CuO/Ag-0.06 surfaces to the aqueous droplets of pH = 1–14 are all greater than 150°. Immersion of the F-CuO/Ag-0.06 surface into hydrochloric acid solution at pH = 1, sodium hydroxide solution at pH = 14, and saturated sodium chloride solution resulted in the appearance of silver mirrors, indicating that the surface has good resistance to acid, alkali, and salt solutions (Fig. 7b). To determine the long-term stability of the superamphiphobic surface outdoors, the surface was irradiated under a UV lamp with a wavelength of 365 nm and a power of 10 W for 48 hours, and the contact angles to water and colza oil were measured every six hours. Fig. 7c shows that after 48 hours of irradiation, the contact angle between water and oil was still greater than 150°, indicating the excellent UV resistance of the layer. When the modified copper sheet is exposed to UV light for 7 days, water and oil droplets still roll very easily.
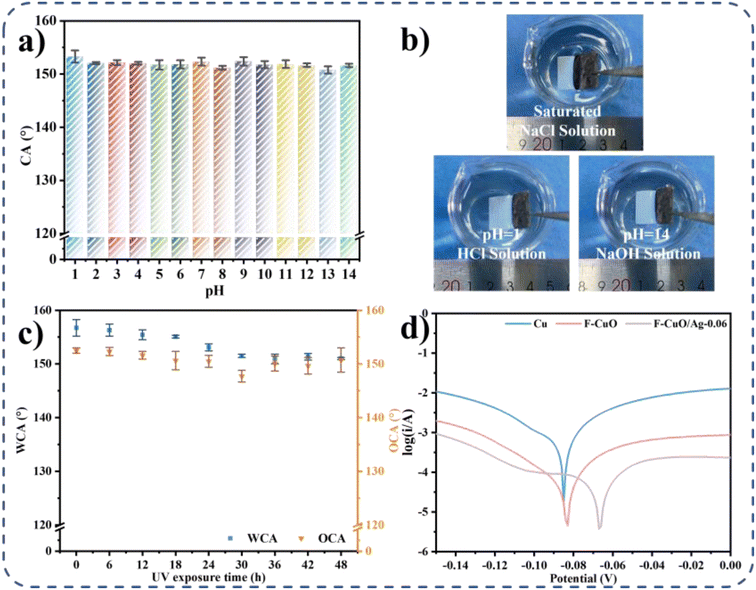 |
| Fig. 7 (a) Contact angle of the F-CuO/Ag-0.06 surface to water at pH = 1–14; (b) silver mirror phenomenon of the F-CuO/Ag-0.06 surface immersed in hydrochloric acid solution at pH = 1, sodium hydroxide solution at pH = 14, and saturated sodium chloride solution; (c) contact angle of the F-CuO/Ag-0.06 surface to water and edible oil after UV irradiation from 0 to 48 hours contact angle; (d) kinetic potential polarization curves of the unmodified Cu surface, F-CuO surface and F-CuO/Ag-0.06 surface. | |
F-CuO/Ag-0.06 surfaces with an area of 1.5 cm2 were immersed in a 3.5 wt% NaCl solution, and the corrosion resistance of unmodified copper sheets, F-CuO superhydrophobic copper sheets, and F-CuO/Ag-0.06 superamphiphobic copper sheets was measured by kinetic potential polarization. Fig. 7d and Table 1 show that the corrosion voltage of superamphiphobic copper sheet is the smallest and the resistance is the largest, indicating high and stronger corrosion protection. This is attributed to the fact that the re-entrant micro/nano-textured structure of the superamphiphobic copper surface traps more air, resulting in a larger solid-air contact area, and so is able to exhibit a more aggressive corrosion potential and better corrosion resistance than superhydrophobic and normal copper sheets.
Table 1 Corrosion characteristics of the Cu, F-CuO and F-CuO/Ag-0.06 surfaces
Samples |
i
corr (A) |
E
corr (V) |
R
corr (Ω) |
Cu |
7.858 × 10−3 |
−0.085 |
8.8 |
F-CuO |
4.416 × 10−4 |
−0.083 |
89 |
F-CuO/Ag-0.06 |
2.390 × 10−4 |
−0.067 |
130.7 |
3.4. Adhesion analysis of superamphiphobic copper surfaces
Waxing and flow blockages in the petroleum industry cause serious safety issues and economic losses. SAP surfaces with anti-adhesion properties may be a potential strategy to solve these problems. Fig. 8a shows the pinning and rebounding behaviors of the droplets (volume of 5 μL) as they are released from a height of 2 mm toward the SAP surface. To further confirm the low interfacial adhesion of the low surface tension liquid on the F-CuO/Ag-0.06 surface, a goniometer was used to record the dynamic contact behavior of the droplets. Fig. 8b shows that as water, glycerol, ethanediol, and colza oil moved downward, contacted, pressed, and separated upward from the coating surface, the four liquids did not have any residual traces on the surface, reflecting the extremely low liquid–solid interfacial adhesion and excellent superamphiphobicity.
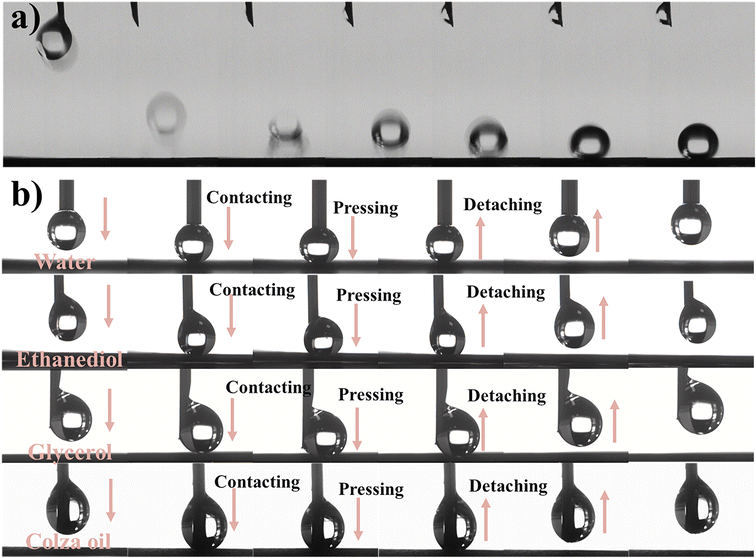 |
| Fig. 8 (a) Pinning and rebounding behavior of water droplets; (b) dynamic liquid-coating interface contact behavior on SAP. | |
3.5. Anti-fouling and self-cleaning properties of superamphiphobic copper surfaces
Fig. 9a and Movies S1–S4† demonstrate the exceptional oil and stain resistance of the F-CuO/Ag-0.06 surface. The superamphiphobic copper sheet was immersed in water stained with methylene blue and crude oil, both of which had high adhesion, for 10 seconds. After the removal of the sheet, the surface remained clean. This confirms the surface's outstanding antifouling capability and low adhesion.
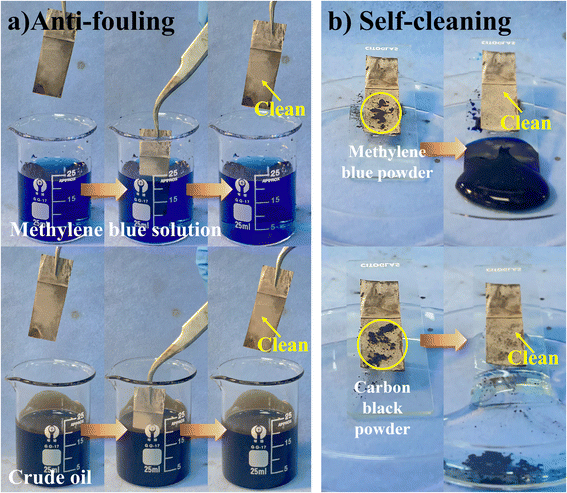 |
| Fig. 9 (a) Antifouling experiments of the F-CuO/Ag-0.06 surface against water and crude oil; (b) self-cleaning experiments of the F-CuO/Ag-0.06 surface against methyl blue powder and carbon black powder. | |
To test the self-cleaning ability of the prepared superamphiphobic surfaces, the modified coatings were tested for self-cleaning using hydrophilic methyl blue powder and hydrophobic carbon black powder as contaminants (Fig. 9b). As can be observed from Fig. 9b and Movies S1 and S2,† water droplets take away the methyl blue powder when rolling from the F-CuO/Ag-0.06 surface, and colza oil, when reaching the sample surface, quickly scratches from the surface and then takes away the carbon black powder. In the end, both resulted in clean surfaces with no droplets or contaminants remaining.
3.6. Controlled transport of oil droplets
A SAPS with adjustable oil adhesion can serve as a switch to control the movement and stoppage of oil droplets on the surface (Fig. 10a). As shown in Fig. 10b and Movies S5–S8,† we successfully achieved the transport of glycerol (Movie S5†), colza oil (Movie S6†), n-hexadecane (Movie S7†), and crude oil (Movie S8†) on SAPS with low adhesion (sample F-CuO/Ag-0.54), medium adhesion (sample F-CuO/Ag-0.18), and high adhesion (sample F-CuO/Ag-0.06). To achieve this controllable transport of oil droplets, it only requires simple adjustment of the laser-etched patterns (i.e., groove size) and the tilt angle of the modified copper plate. This enables spontaneous movement and stoppage of droplets with different viscosities on the surface. In order to study the relationship between groove size and the adhesion of the modified copper surface, colza oil was used as the test liquid, and the adhesion of the surface was tested using a surface tensiometer. As shown in Fig. 10c, as the groove size decreases, the maximum adhesion force (Fmax) tends to increase. The adhesion force variation curves in Fig. 10d demonstrate the change from low to high adhesion for samples with G = 0.54, 0.18, and 0.06. From the sliding angle data shown in Fig. 10e, it can also be observed that surfaces with high, medium, and low adhesive forces exhibit an increasing trend in the sliding angle. Therefore, by adjusting the tilt angle of the copper plate, different types of oil droplets can be transported and collected spontaneously.
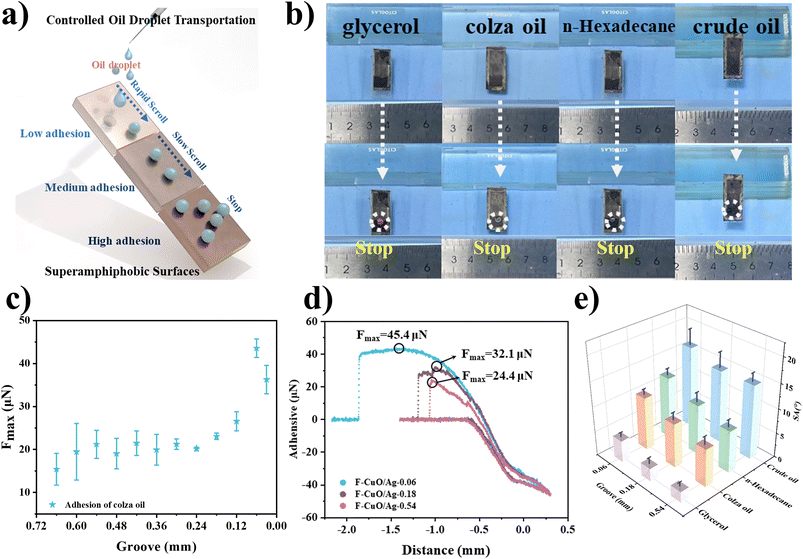 |
| Fig. 10 (a) Schematic diagram of a superamphiphobic surface with adjustable oil adhesion properties controlling droplet transportation; (b) optical photographs of the controlled transportation of glycerol, colza oil, n-hexadecane, and crude oil on the modified copper surface; (c) the maximum adhesion force of colza oil on the modified copper surface with different groove sizes; (d) adhesion force variation curve of superamphiphobic surfaces with low, medium, and high adhesion forces. (e) Sliding angle (SA) of superamphiphobic copper sheets with low, medium and high adhesion forces. | |
4. Conclusions
In summary, we demonstrate a process-simple, parametrically controllable, and highly reproducible way to create superamphiphobic copper surfaces by a combination of laser etching and chemical solution immersion. The advantage of laser etching is the ability to tune the repellency to different types of oils by simply changing the etching parameters. The exceptional superamphiphobicity is attributed to the concave-down reentrant micro/nano structure, which creates an upward synergistic force at the three-phase contact point. This force minimizes the solid–liquid contact area, effectively trapping air and enhancing superamphiphobicity. The modified copper surface exhibits a surface tension of less than 25.7 mN m−1, with CA values exceeding 150° for low surface tension liquids such as n-hexadecane and crude oil. Moreover, immersion of the modified surfaces in strong acid, alkali and salt solutions produced a silver mirror reaction, while the CA with water and colza oil remained greater than 150° even after 48 hours of UV irradiation, demonstrating excellent acid and alkali resistance and UV resistance. Additional tests, including water droplet bounce and dynamic liquid contact behavior, confirmed the surfaces' low adhesion properties, while self-cleaning and anti-fouling tests verified their self-cleaning effect. Furthermore, kinetic potential polarization measurements revealed improved corrosion resistance of the superamphiphobic copper surfaces compared to unmodified and superhydrophobic copper sheets. In addition, by simply changing the pattern, it is possible to modulate the oil adhesion to the SAP copper surface, enabling the transport manipulation of crude oil, n-hexadecane, colza oil and glycerol. The modified SAP copper sheets have important applications in aircraft, automobiles, ships, crude oil transfer, fluid power systems, oil resistance, anti-crawling materials, anti-fouling, and so on.
Conflicts of interest
There are no conflicts to declare.
Acknowledgements
This work is supported by the National Natural Science Foundation of China (No. 51735013).
References
- Q. Wen, F. Guo, Y. Peng and Z. Guo, Colloids Surf., A, 2018, 539, 11–17 CrossRef CAS.
- J. Wei, B. Li, L. Jing, N. Tian, X. Zhao and J. Zhang, Chem. Eng. J., 2020, 390, 124562 CrossRef CAS.
- V. S. Saji, J. Magnesium Alloys, 2021, 9, 748–778 CrossRef CAS.
- P. Ragesh, V. Anand Ganesh, S. V. Nair and A. S. Nair, J. Mater. Chem. A, 2014, 2, 14773–14797 RSC.
- S. Farhadi, M. Farzaneh and S. A. Kulinich, Appl. Surf. Sci., 2011, 257, 6264–6269 CrossRef CAS.
- Y. Li, T. Hu, B. Li, J. Wei and J. Zhang, Adv. Mater. Interfaces, 2019, 6, 1901255 CrossRef CAS.
- F. Guo, Q. Wen, Y. Peng and Z. Guo, J. Colloid Interface Sci., 2017, 494, 54–63 CrossRef CAS PubMed.
- L. Schmuser, N. Encinas, M. Paven, D. J. Graham, D. G. Castner, D. Vollmer, H. J. Butt and T. Weidner, Biointerphases, 2016, 11, 031007 CrossRef PubMed.
- J. Wang, H. Wang, Y. Wang, P. Gao, F. Wang, X. Men, Z. Zhang and Y. Lu, Chem. Eng. J., 2021, 420, 129806 CrossRef CAS.
- X.-J. Guo, C.-H. Xue, S.-T. Jia and J.-Z. Ma, Chem. Eng. J., 2017, 320, 330–341 CrossRef CAS.
- J. Yang, H. Song, H. Ji and B. Chen, J. Adhes. Sci. Technol., 2014, 28, 1949–1957 CrossRef CAS.
- M. J. Hoque, S. Sett, X. Yan, D. Liu, K. F. Rabbi, H. Qiu, M. Qureshi, G. Barac, L. Bolton and N. Miljkovic, ACS Appl. Mater. Interfaces, 2022, 14, 4598–4611 CrossRef CAS PubMed.
- D. Wang, X. Wang, X. Liu and F. Zhou, J. Phys. Chem. C, 2010, 114, 9938–9944 CrossRef CAS.
- K. Tsujii, T. Yamamoto, T. Onda and S. Shibuichi, Angew. Chem., Int. Ed., 1997, 36, 1011–1012 CrossRef CAS.
- A. Tuteja, W. Choi, M. Ma, J. M. Mabry, S. A. Mazzella, G. C. Rutledge, G. H. McKinley and R. E. Cohen, Science, 2007, 318, 1618–1622 CrossRef CAS PubMed.
- J. Yong, F. Chen, Q. Yang, J. Huo and X. Hou, Chem. Soc. Rev., 2017, 46, 4168–4217 RSC.
- A. Shome, A. Das, A. Borbora, M. Dhar and U. Manna, Chem. Soc. Rev., 2022, 51, 5452–5497 RSC.
- L. Chen, Z. Guo and W. Liu, J. Mater. Chem. A, 2017, 5, 14480–14507 RSC.
- Z. Chu and S. Seeger, Chem. Soc. Rev., 2014, 43, 2784–2798 RSC.
- X. Deng, L. Mammen, H. J. Butt and D. Vollmer, Science, 2012, 335, 67–70 CrossRef CAS PubMed.
- A. Dhyani, J. Wang, A. K. Halvey, B. Macdonald, G. Mehta and A. Tuteja, Science, 2021, 373(6552), eaba5010 CrossRef CAS PubMed.
- X. Gou and Z. Guo, Adv. Colloid Interface Sci., 2019, 269, 87–121 CrossRef CAS PubMed.
- H.-J. Choi, S. Choo, J.-H. Shin, K.-I. Kim and H. Lee, J. Phys. Chem. C, 2013, 117, 24354–24359 CrossRef CAS.
- S. M. Kang and J. S. Choi, Small, 2020, 16, 1904612 CrossRef CAS PubMed.
- S. Y. Lee, Y. Rahmawan and S. Yang, ACS Appl. Mater. Interfaces, 2015, 7, 24197–24203 CrossRef CAS PubMed.
- S. Dong, X. Zhang, Q. Li, C. Liu, T. Ye, J. Liu, H. Xu, X. Zhang, J. Liu, C. Jiang, L. Xue, S. Yang and X. Xiao, Small, 2020, 16, 2000779 CrossRef CAS PubMed.
- Y. Zhang, Z. Xiao, C. Liu and X. Yu, Soft Matter, 2019, 15, 7374–7380 RSC.
- Y. Qing, S. Shi, C. Lv and Q. Zheng, Adv. Funct. Mater., 2020, 30, 1910665 CrossRef CAS.
- S. Wang, B. Fan, C. Lv, X. Jia and T. Wang, J. Alloys Compd., 2020, 831, 154741 CrossRef CAS.
- D. Wang, Q. Sun, M. J. Hokkanen, C. Zhang, F. Y. Lin, Q. Liu, S. P. Zhu, T. Zhou, Q. Chang, B. He, Q. Zhou, L. Chen, Z. Wang, R. H. A. Ras and X. Deng, Nature, 2020, 582, 55–59 CrossRef CAS PubMed.
- X. Zhu, Z. Zhang, X. Xu, X. Men, J. Yang, X. Zhou and Q. Xue, J. Colloid Interface Sci., 2012, 367, 443–449 CrossRef CAS PubMed.
- J. Zhu and K. Liao, ACS Appl. Mater. Interfaces, 2021, 13, 37830–37839 CrossRef CAS PubMed.
- D. Chu, S. C. Singh, J. Yong, Z. Zhan, X. Sun, J. A. Duan and C. Guo, Adv. Mater. Interfaces, 2019, 6, 1900550 CrossRef.
- J. Chao, J. Feng, F. Chen, B. Wang, Y. Tian and D. Zhang, Colloids Surf., A, 2021, 610, 125708 CrossRef CAS.
- A. B. D. Cassie and S. Baxter, Trans. Faraday Soc., 1944, 40, 546–551 RSC.
- L. Chen, Z. Guo and W. Liu, ACS Appl. Mater. Interfaces, 2016, 8, 27188–27198 CrossRef CAS PubMed.
- K. Wang, X. Liu, Y. Tan, W. Zhang, S. Zhang, J. Li and A. Huang, Chem. Eng. J., 2019, 359, 626–640 CrossRef CAS.
- S. Masoud Emarati and M. Mozammel, Chem. Eng. J., 2020, 387, 124046 CrossRef CAS.
- S. Martin, P. S. Brown and B. Bhushan, Adv. Colloid Interface Sci., 2017, 241, 1–23 CrossRef CAS PubMed.
- L. Liu, Y. Pan, B. Bhushan, F. Li and X. Zhao, Chem. Eng. J., 2020, 391, 123523 CrossRef CAS.
|
This journal is © The Royal Society of Chemistry 2024 |
Click here to see how this site uses Cookies. View our privacy policy here.