DOI:
10.1039/D3TA06341K
(Paper)
J. Mater. Chem. A, 2024,
12, 1753-1763
Enhanced piezoelectricity and spectral absorption in Nd-doped bismuth titanate hierarchical microspheres for efficient piezo-photocatalytic H2 production and pollutant degradation†
Received
18th October 2023
, Accepted 8th December 2023
First published on 20th December 2023
Abstract
Hydrothermally synthesized Nd-doped bismuth titanate (Bi3.15Nd0.85Ti3O12, BIT-Nd) hierarchical microspheres with assembled nanosheets were developed for water splitting and wastewater purification utilizing the piezo-phototronic effect for the first time. Undoped Bi4Ti3O12 (BIT) microspheres were simultaneously studied for comparison to reveal the role of Nd doping. Under the combined light and ultrasound excitation, the degradation efficiency of RhB (C0 = 10 mg L−1) solution reached 97.5% in 8 minutes in the presence of the BIT-Nd microspheres, leading to an ultrahigh first-order rate constant k of 0.407 min−1, which was 4.9 times higher than that of the BIT microspheres. More impressively, superior piezo-photocatalytic activity in water splitting compared to that of other reported piezo-photocatalysts was achieved in the BIT-Nd microspheres, which showed a H2 evolution rate of 1330.3 μmol g−1 h−1 without any cocatalyst or scavenger. To understand the origins of the excellent piezo-photocatalytic performance, microstructure analysis and piezoelectric and photoelectric characterization, as well as density functional theory calculations were carried out. After Nd doping, the atomic framework of the Ti–O octahedron underwent an apparent distortion, resulting in a stronger ferroelectric polarization, and thus a larger piezoelectric potential output under pressure. Moreover, the thinner nanosheets in the BIT-Nd microspheres were more susceptible to deformation under the ultrasonic effect, which was also beneficial to generate a higher piezoelectric field that inhibited the charge carrier recombination. Additionally, the presence of Nd 4f orbitals in BIT-Nd effectively reduced the band gap and promoted the spectral absorption and production of photogenerated carriers. These merits enabled the BIT-Nd microspheres to exhibit strong piezo-photocatalytic activity.
1. Introduction
The utilization of semiconductor photocatalysts has great potential to alleviate the energy crisis and environmental pollution.1,2 In general, efficient absorption of light, rapid separation and transfer of photoexcited charge carriers, and abundant active sites contribute to high photocatalytic performance.3 However, the unsatisfactory solar energy utilization and the high recombination of photoexcited charge carriers in conventional semiconductor materials are significant issues that limit the photoelectric conversion efficiency, and result in weak and inefficient photocatalytic activities. A series of measures to improve the photocatalytic efficiency have been considered which include system innovation,4 morphology maneuvering,5 doping modification,6 and heterostructure construction.7 Typically, the piezo-phototronic effect in piezoelectric semiconductors with the coupling of the piezoelectric effect and photoelectric effect has been widely studied due to the alternating built-in electric field which is beneficial to facilitate the charge separation.8–10
In recent years, the material family showing the piezo-phototronic effect has been extended from metal oxides, e.g. ZnO11 and perovskite structure ferroelectrics, e.g. BaTiO3 (ref. 12) to layered two-dimensional (2D) materials, e.g. g-C3N4.13 Among the piezo-phototronic semiconductors, bismuth layer-structured ferroelectric Bi4Ti3O12 (BIT) is particularly attractive owing to its special electronic structure of a hybridized valence band composed of Bi 6s and O 2p orbitals that facilitate the generation of electron–hole pairs,14 and the favorable ferroelectricty with a spontaneous polarization of ∼40 μC cm−2 along the a-axis and ∼4 μC cm−2 along the c-axis.15 Moreover, the layered structure with [Bi2O2]2+ bismuth oxide layers and [Bi2Ti3O10]2− perovskite layers stacked alternately along the c-axis allows the growth of BIT crystals with a 2D shape, which is easy to be deformed under mechanical vibrations.16 Most of the studies about the piezo-photocatalytic performance of BIT nanostructures are concentrated on wastewater purification, including dye degradation. For examples, Tang et al. reported the piezo-photocatalytic activity of BIT nanosheets with different oxygen vacancy concentrations, which reached a k ∼ 0.214 min−1 for the decomposition of RhB solution (C0 = 5 mg L−1).17 Bai et al. prepared Bi4Ti3−2nCrnNbnO12/x-g-C3N4 heterojunctions which showed a piezo-photocatalytic degradation efficiency of RhB (C0 = 10 mg L−1) of 98.7% within 45 min.18 Nevertheless, the relatively large band gap (Eg = 3.29 eV) of BIT limits the spectrum utilization efficiency,19 and on the basis of the present research, the piezo-photocatalytic performance of BIT-based nanostructures is worth further optimization.
Metal ion doping is a popular and effective strategy to modulate the piezoelectric and photoelectric properties of BIT-based crystals. Rare earth metal ion doping in BIT, i.e., Bi3.25M0.75Ti3O12 (BMT, M = La, Sm, Nd, and Eu), has been proven to be effective to enhance the visible light absorption (narrowed band gap) and restrict the recombination of electron/hole pairs, leading to the improved photocatalytic activity.20 However, the underlying mechanisms related to the band structure or electronic configuration have not been clarified. On the other hand, in BIT-based bulk ceramics, it has been demonstrated that the substitution of Bi3+ by the lanthanide series Nd3+ ions with a smaller ionic radius [r(Bi3+) = 1.30 Å, r(Nd3+) = 1.27 Å] can apparently enhance the spontaneous polarization and thus piezoelectric response due to the increased structural distortion that is the rotation of the TiO6 octahedron in the a–b plane and the incline of the distorted TiO6 octahedron away from the c-axis.21 For example, Chon et al. concluded that the largest value of remanent polarization (2Pr = 103 μC cm−2) was achieved at x = 0.85 in the Bi4−xNdxTi3O12 system,22,23 and the piezoelectric coefficient increased from ∼7.3 pC N−1 (ref. 24) to ∼14 pC N−1.25 These results inspire us to explore the piezo-photocatalytic performance of Nd-doped BIT nanostructures, which are expected to possess higher piezoelectric potential output and enhanced spectral absorption compared to the widely-studied BIT piezo-phototronic nanocatalysts. Specifically, their piezo-photocatalytic activity in water splitting would be of great interest considering that no relevant research has been conducted to date.
Since the catalytic activity of piezo-photocatalysts is structure-dependent, their morphology design is of significance for efficient light and mechanical energy capture and transformation.26 One-dimensional nanostructures such as nanowires and nanorods, as well as two-dimensional nanostructures like nanosheets, are inherently inclined towards improving charge separation and reducing charge recombination.3 Among them, the large flat size of nanosheets provides them with a large capture area for light and mechanical energy, presenting great potential for highly efficient piezo-photocatalysis.27 In this work, Bi3.15Nd0.85Ti3O12 (BIT-Nd) hierarchical microspheres with assembled nanosheets are synthesized by a hydrothermal method, and we provided the first evaluation of the piezo-photocatalytic performance of the BIT-Nd hierarchical microspheres in the aspects of water splitting for H2 production and dye degradation at high/low frequencies. Using a combination of piezoresponse force microscopic measurements, UV-vis absorbance spectrum characterization, finite element analysis, and density functional theory calculations, an in-depth understanding of the excellent piezo-photocatalytic performance of the BIT-Nd hierarchical microspheres is presented in this work.
2. Results and discussion
2.1 Morphology and microstructure
Fig. 1(a) shows the XRD patterns of the Bi4Ti3O12 (BIT) and Bi3.15Nd0.85Ti3O12 (BIT-Nd) powders in the 2θ range of 10–60°. The XRD peaks are well matched with those of orthorhombic Bi4Ti3O12 (JCPDS #80-2143), demonstrating that Nd3+ has been successfully dispersed into the crystal lattice to form a solid solution. The Nd3+ ions replace and occupy the Bi3+ ion positions due to their similar ionic radii ((r(Nd3+) = 1.27 Å), r(Bi3+) = 1.30 Å). Fig. 1(b) shows that the peak (117) shifts to lower angles with the introduction of Nd, indicating a slight increase in both lattice parameters and volume of the unit cell.28 In addition, for the pure BIT powders, a typical orthorhombic symmetry was observed, as evidenced by the splitting of
and (202) characteristic peaks at 2θ values of 47.3° and 47.9°, respectively. For BIT-Nd powders, the peak splitting disappeared and only a single peak can be observed, indicating a phase transformation from the orthorhombic phase into the pseudo-cubic structure phase.29Fig. 1(c)–(f) show the SEM and AFM images of the BIT and BIT-Nd powders. BIT-Nd exhibits regular spherical structures composed of self-assembled nanosheets with a uniform size. This nanosheet-assembled hierarchical microsphere structure inherits the initial undoped BIT structure. The average edge length of BIT nanosheets is ∼470 nm, with an average thickness of ∼78 nm. In contrast, the BIT-Nd nanosheets show an edge length of ∼400 nm with an average thickness of ∼35 nm, which is lower than half of that of the BIT nanosheets. The thinner nanosheets in BIT-Nd reveal that Nd3+ doping as intercalation ions in BIT can reduce the crystallization rate of the nanosheets.30 The EDS mapping reveals a homogeneous distribution of Bi, Ti, Nd and O elements in both BIT and BIT-Nd, as shown in Fig. S1.† Fig. S2† shows that the dominant pore sizes of BIT-Nd and BIT both are ∼40 nm, and the surface areas of BET are 32.61 m2 g−1 and 27.67 m2 g−1 respectively.
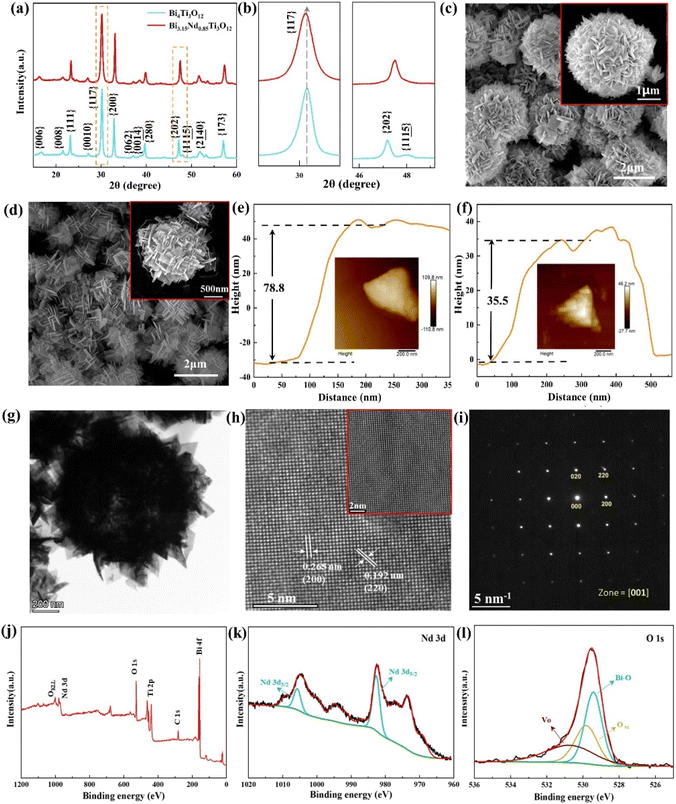 |
| Fig. 1 Morphology and microstructure characterization of the BIT and BIT-Nd hierarchical microspheres. (a) XRD patterns and (b) expanded XRD patterns of the BIT and BIT-Nd powders. SEM images of the (c) BIT and (d) BIT-Nd hierarchical microspheres. AFM images and the corresponding height curves of the (e) BIT nanosheets and (f) the BIT-Nd nanosheets. (g) Bright-field TEM image, (h) atomic-resolution TEM image, (i) SAED pattern, and (j) survey XPS spectrum of the BIT-Nd hierarchical microspheres. High-resolution XPS spectra of (k) Nd 3d and (l) O 1s of the BIT-Nd hierarchical microspheres. | |
Fig. 1(g)–(i) display the TEM observation results of the BIT-Nd hierarchical microspheres. Fig. 1(g) shows that the assembled nanosheets are well-defined with a clear rectangular outline. The atomic-resolution TEM image, as depicted in Fig. 1(h), exhibits uniform fringes across the entire nanosheet. The interplanar crystal spacings were measured to be 0.265 nm and 0.192 nm, which are in good agreement with the (200) and (220) atomic planes, respectively. The corresponding selected-area electron diffraction (SAED) pattern in Fig. 1(i) shows that BIT-Nd shows a single crystal characteristic. The survey XPS spectra in Fig. 1(j) reveal signals for Bi, Ti, O, Nd, and C elements, indicating the absence of impurities in BIT-Nd. Based on the value of binding energy, the Bi3+ and Ti4+ states can be demonstrated as shown in Fig. S3.† Moreover, the XPS spectrum of the Nd 3d peak can be deconvoluted into two peaks at 1005.8 and 982.5 eV by Gaussian processing, as shown in Fig. 1(k), corresponding to Nd 3d3/2 and Nd 3d5/2 of Nd3+. Notably, the binding energy located at 531 eV is observed, often attributed to the O2− ions in oxygen deficient (VO) regions (Fig. 1(l)). The presence of VO favors the adsorption and activation of O2 molecules on the surface of piezoelectric materials, which is conducive to accumulation of electrons from the localized electrons of oxygen vacancies, ultimately leading to the generation of ·O2−.31,32
2.2 Piezoelectric properties and optical properties
The optical responses of the BIT and BIT-Nd hierarchical microspheres were investigated by measuring the UV-vis absorbance spectra as shown in Fig. 2(a). The BIT-Nd powders demonstrated higher absorption intensity in the wavelength range of 450–800 nm, compared with BIT powders, which indicated that the effective doping improved the light absorption performance. Based on the equation of αhυ = B(hυ − Eg)1/2,33 the band gap (Eg) of BIT-Nd and BIT were calculated to be 2.89 eV and 3.11 eV, respectively. Enhanced light absorption and a narrowed band gap are both beneficial to the higher sunlight energy utilization and enhancement of photocatalytic activity. Both BIT and BIT-Nd are n-type semiconductors with positive slopes at different frequencies (1 kHz, 2 kHz, and 3 kHz), as shown in Fig. 2(b). The flat band potential of BIT and BIT-Nd is −0.51 V and −0.44 V, respectively. The transient photocurrent intensities were measured over several cycles of intermittent light irradiation to demonstrate the photo-excited carrier separation behavior of BIT and BIT-Nd, as shown in Fig. 2(c). The photocurrent density of BIT-Nd reaches 7.44 μA cm−2 and is 1.7 times higher than that of BIT, indicating that photogenerated carriers are efficiently separated in BIT-Nd. The EIS Nyquist plot of BIT-Nd presents a smaller radius compared to that of BIT, demonstrating the lower charge transport resistance, which contributes to the improvement of piezophotocatalytic activity, as shown in Fig. 2(d). As shown in Fig. 2(e) and (f), by applying a ±12 V DC bias field, both BIT and BIT-Nd exhibit classical amplitude-voltage butterfly loops and well-defined 180° phase reversal hysteresis loops, confirming the existence of ferroelectric switch behavior and piezoelectricity.34 Importantly, the maximum piezoelectric response amplitude of BIT-Nd is about 2.3 times larger than that of BIT, indicating the higher piezoelectric response of BIT-Nd.35 Therefore, the BIT-Nd hierarchical microspheres with high surface area, a smaller band gap, stronger photonic excitation, faster charge transfer and higher piezoelectricity could lead to a superior piezo-photocatalytic activity.
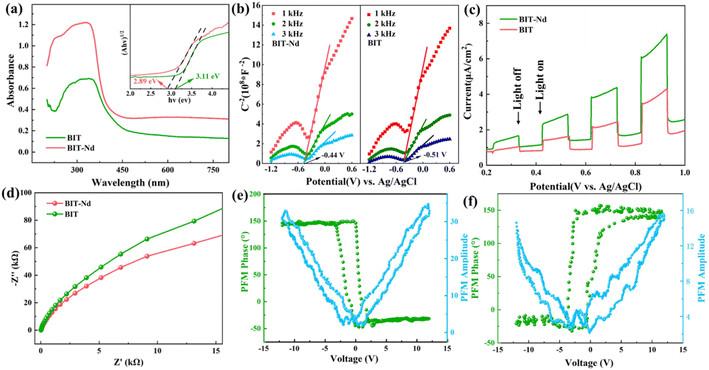 |
| Fig. 2 Piezoelectric and optical properties of the BIT and BIT-Nd hierarchical microspheres. (a) UV-vis diffuse reflectance spectra and the corresponding band gap of BIT and BIT-Nd. (b) Mott–Schottky plots of BIT-Nd and BIT at different frequencies of 1 kHz, 2 kHz, and 3 kHz. (c) Transient photocurrent responses and (d) EIS Nyquist plots of BIT and BIT-Nd. PFM amplitude-voltage butterfly loop and PFM phase hysteresis loop of (e) BIT-Nd and (f) BIT. | |
To better understand the change in Eg, the theoretical calculations were performed using DFT to investigate the band structures of BIT before and after Nd3+ doping. The calculations were based on the BIT unit cell, with consideration given to Nd doping by replacing two Bi atoms with two Nd atoms. As shown in Fig. 3(a) and (b), the calculated Eg of BIT-Nd (1.57 eV) is smaller than that of BIT (2.32 eV), which is consistent with the results of the experimental analysis (Fig. 2(a)). However, both of them are lower than the values of experimental Eg due to the ignored self-interaction correction and discontinuity in the exchange–correlation energy and potential.36,37 The bottom conduction band (CB) of BIT is mainly composed of Ti 3d and Bi 6p orbitals, whereas the top valence band (VB) is mainly composed of Ti 3d, Bi 6s and O 2p orbitals. The CB of BIT-Nd is mainly attributable to the Ti 3d, Bi 6p and Nd 4f orbitals, and the Ti 3d, Bi 6s and O 2p and Nd 4f orbitals are the main contributions to the VB. The reduction in Eg of BIT-Nd may be attributed to the presence of Nd 4f orbitals and the introduction of an additional spin polarization valence band (SPVB) in the original band gap of BIT.18
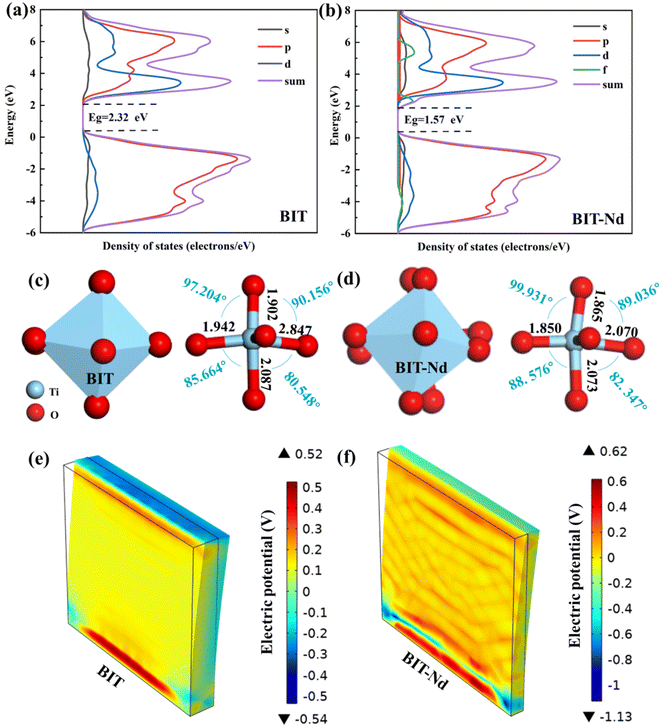 |
| Fig. 3 Theoretical calculations of the band structure and lattice structure of BIT and BIT-Nd. Density of states (DOS) of (a) BIT and (b) BIT-Nd calculated by DFT calculations. [TiO6] octahedron structures of (c) BIT and (d) BIT-Nd and the corresponding theoretical bond lengths (unit: Å) and bond angles. FEM simulation of the piezoelectric potential distribution in (e) BIT and (f) BIT-Nd nanosheets with lateral deformation. | |
The influence of Nd3+ doping on the ferroelectric polarization was calculated by using DFT, where BIT-Nd was constructed by replacing two Bi atoms in the pseudo-perovskite layer with Nd atoms.18Fig. 3(c) and (d) show the structural changes in the Ti–O octahedron in BIT before and after Nd3+ doping. Clearly, after substituting Bi with a Nd atom, the atomic framework of the Ti–O octahedron exhibits significant distortion, leading to a reduction in the symmetry of the crystal structure, as shown in Fig. S4.† The Ti–O bond lengths of BIT-Nd decrease, and the [TiO6] octahedron exhibits a greater rotation angle compared to that of BIT. Therefore, for BIT-Nd, the TiO6 octahedron possesses a larger distortion, which serves to enhance polarization and promote piezoelectric response. To investigate the generated piezoelectric potential distribution in BIT and BIT-Nd nanosheets, finite-element method (FEM) simulations were performed, as presented in Fig. 3(e) and (f). The geometrical parameters, including the edge length and thickness, were close to the actual sizes observed by SEM, as shown in Fig. 1(c) and (d). The size of the simulated BIT was 470 nm × 470 nm × 78 nm, while the size of the simulated BIT-Nd was 400 nm × 400 nm × 35 nm. In addition, the applied stress was set as 100 MPa considering the impact force caused by acoustic cavitation and acoustic pressure38 and the bottom surface was fixed and grounded.34 FEM simulation shows that the maximum piezoelectric potential generated by BIT and BIT-Nd nanosheets was 1.06 V and 1.75 V, respectively. The thinner nanosheet has a larger aspect ratio (length/diameter) and a smaller ground contact area than the thicker one, resulting in easier deformation and a higher piezoelectric potential, which may result in a more efficient catalytic process.
2.3 Catalytic activities for dye degradation and H2 evolution
Fig. 4(a) and (b) illustrate the degradation efficiency of RhB solution in the presence of the BIT-Nd hierarchical microspheres under different degradation conditions. As shown in Fig. 4(a), the degradation of RhB was negligible only under stirring at a speed of 200 rpm (referred to as “stir200 + dark” in Fig. 4), suggesting that low-frequency stirring had little effect on the photocatalytic reaction. However, RhB degradation rates of 91.9% and 97.3% were achieved within 15 minutes under the conditions of “Stir200 + light” and “ultrasonic + dark”, respectively. Moreover, combining the effect of light and ultrasonic excitations remarkably enhanced the degradation of RhB solution, reaching 97.5% within 8 minutes. The trend of the kinetic curve is consistent with linear fitting kinetic curve trends,39,40 and the resulting k and R2 values are summarized in Fig. 4(b). Compared to photocatalysis alone (k = 0.142 min−1) and piezocatalysis alone (k = 0.227 min−1), the k value achieved by the combined piezo-photocatalysis (k = 0.407 min−1) surpasses the sum of the k values for the above two individual catalytic process, as shown in Fig. 4(b). This enhancement is the result of the piezo-phototronic coupling effect, where the piezoelectric field facilitates the separation of carriers and enhances the piezo-photocatalytic performance.33 Further discussion on the insight mechanisms behind photocatalysis and piezo-photocatalysis will be provided later.
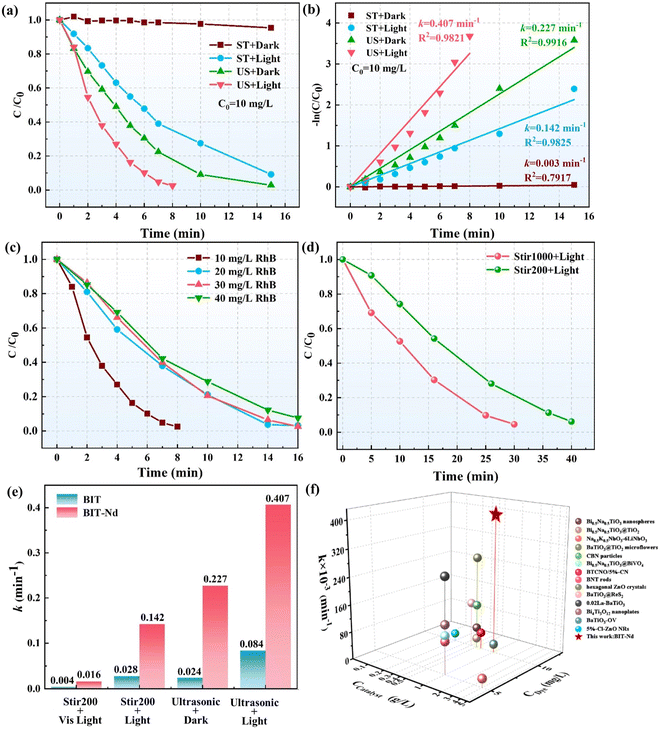 |
| Fig. 4 Piezo-photocatalytic performance of the BIT-Nd and BIT hierarchical microspheres for RhB degradation. (a) Relative variations of RhB concentration over time (C0 = 10 mg L−1) under different degradation conditions in the presence of BIT-Nd and (b) the corresponding ln(C/C0) − t plots with the calculated k and R2 values. (c) Piezo-photocatalytic activity of BIT-Nd for degrading the RhB solution with different initial concentrations. (d) Piezo-photocatalytic activity of BIT-Nd for degrading the RhB solution (C0 = 40 mg L−1) at different stirring speeds. (e) Comparison of the k values between BIT and BIT-Nd for the degradation of RhB solution (C0 = 10 mg L−1) under different conditions. (f) The k values achieved in this work and recently reported piezo-photocatalysis studies. | |
Fig. 4(c) gives the comparison of piezo-photocatalytic performance of BIT-Nd at different initial concentrations of the RhB solution. With an increase in the dye concentration, the degradation rate decreases due to the limited active sites on the surface of the catalyst, which are insufficient for degrading high-concentration dyes. Furthermore, ultrasonic waves are rarely found in natural water systems, and therefore, piezocatalysis driven by the low-frequency water flow has long been pursued. In order to evaluate the piezo-photocatalytic activity of BIT-Nd driven by the low-frequency water flow, the catalytic performance of BIT-Nd for degrading high-concentration RhB solutions (C0 = 40 mg L−1) under the conditions of light and stirring with different stirring speeds was measured, as shown in Fig. 4(d). Under light illumination, as the stirring speed increased from 200 to 1000 rpm, the time required for degrading 95% RhB decreased from 40 to 30 minutes. This degradation rate is comparable to the recently reported degradation rates of other piezo-photocatalysts under ultrasonication and illumination,18,39,41,42 indicating the great potential of the BIT-Nd hierarchical microspheres for future low-frequency piezocatalysis applications.
Furthermore, we compared the catalytic activity of BIT-Nd and BIT for the degradation of RhB solution (C0 = 10 mg L−1) under different conditions. The reduction of RhB concentration over time and the calculated k value are summarized in Fig. S5† and 4(e), respectively. BIT-Nd presents a higher piezocatalytic degradation efficiency with a k value of 0.227 min−1, 9.5 times higher than that of BIT, indicating the superior piezocatalytic capability of BIT-Nd. Furthermore, when exposed to light (190–1100 nm) or visible light (420–1100 nm) irradiation alone, better photocatalytic activity of BIT-Nd than that of BIT can be obtained, which is attributed to its narrower band gap, higher light absorption and faster charge transfer. These factors contributed to the generation of more photoinduced electron–hole pairs and a reduced recombination rate of carriers. Moreover, the degradation efficiency is apparently enhanced by combining ultrasonication and light irradiation for both the BIT and BIT-Nd powders. To assess the durability and stability of BIT and BIT-Nd powders, 5 cycles for repeatable piezo-photocatalysis are shown in Fig. S6.† The slight decrease in the degradation rate after 5 cycles exhibits a stable recycling ability of BIT-Nd and BIT powders. For the high-concentration RhB solution (C0 = 40 mg L−1), the piezo-photocatalytic degradation efficiency reached 92.5% within 16 min in the presence of BIT-Nd, which was faster than that of BIT, as shown in Fig. S7.†Fig. 4(f) shows a comprehensive comparison of k values from the recent reports and this work, and the catalytic conditions are summarized in Table S1,† where the catalytic performance of BIT-Nd is highly promising as a coupled piezo-photocatalyst.
In order to investigate the generated active species in the process of piezo-photocatalytic degradation of RhB solution (C0 = 10 mg L−1), phenylhydrazine (BQ), ethylenediamine tetraacetate dehydrate (EDTA), and tert-butyl alcohol (TBA) were used to remove the superoxide (·O2−), holes (h+), and hydroxyl ions (·OH), respectively. As shown in Fig. 5(a), under ultrasonic conditions and light irradiation, the addition of BQ, TBA, and EDTA greatly reduced the decomposition rate of RhB from 97.5% (no additive) to 59.9%, 59.7%, and 39.7%, respectively. The results imply that h+ acts as a major free radical during the degradation process and the active oxygen species ·O2− and ·OH play important roles during the catalytic oxidation process. In addition, the ·OH and ·O2− radical species produced during the photocatalysis, piezocatalysis, and piezo-photocatalysis processes were detected by the electron spin resonance (ESR) method,43 as shown in Fig. 5(b) and (c). The strongest radical signal is detected under the combined conditions of ultrasound and light irradiation, indicating that this state produces relatively higher amounts of active species. This is consistent with the result of the dye degradation mentioned above (Fig. 4(a) and (b)), and further confirms that piezoelectric polarization promotes the photocatalytic performance of BIT-Nd. Based on the above discussion, the possible reaction process can be explained by the following steps:
|  | (1) |
| ·OH + ·O2− + h+ + dye molecules → CO2 + H2O | (4) |
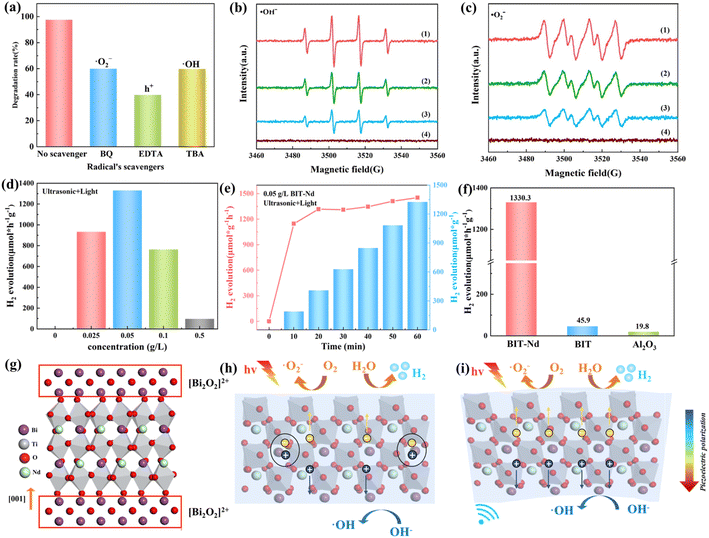 |
| Fig. 5 (a) Under ultrasonic conditions and light excitation, the degradation rate of RhB solution (C0 = 10 mg L−1) in the presence of BIT-Nd and different scavengers. ESR spectra of (b) DMPO-·OH and (c) DMPO/DMSO-·O2− measured during the piezo-photocatalysis (1, ultrasonic + light), piezocatalysis (2, ultrasonic + dark), and photocatalysis (3, Stir200 + light) processes in the presence of BIT-Nd, as well as the piezo-photocatalysis process (4, ultrasonic + light) in the absence of any catalyst. (d) Under ultrasonic conditions and light excitation, the H2 production rates at different contents of BIT-Nd. (e) The amount of H2 over time during the piezo-photocatalysis process (ultrasonic + light) in the presence of 0.05 g L−1 BIT-Nd. (f) The calculated H2 production rates of 0.05 g L−1 BIT-Nd, BIT, and Al2O3. (g) Crystal structure of BIT-Nd. Proposed mechanisms for the (h) photocatalysis and (i) piezo-photocatalysis processes. | |
Since the BIT-Nd hierarchical microspheres exhibited an excellent degradation rate for the RhB aqueous solution, the catalytic performance of BIT-Nd was further assessed by the water splitting for H2 production in pure water, without the addition of cocatalysts and scavengers. Under ultrasonic conditions and light excitation, the yield of H2 increased with increasing BIT-Nd content, when the content of the BIT-Nd is low (<0.05 g L−1). However, when the content of BIT-Nd exceeded 0.05 g L−1 and continued to increase, the production rate of H2 did not further improve due to catalyst aggregation.44 The highest H2 yield (1330.3 μmol g−1 h−1) was achieved when the BIT-Nd content was 0.05 g L−1, indicating an optimized content for high catalytic performance, as illustrated in Fig. 5(d). Fig. 5(e) shows that the production of H2 by BIT-Nd steadily increased over 60 minutes under ultrasonic conditions and light excitation. And the XRD pattern of BIT-Nd powders after six cycles of H2 production is shown in Fig. S8.† The characteristic peaks of BIT-Nd before and after the reaction are almost consistent, indicating the high stability of BIT-Nd powders. As shown in Fig. 5(f), the non-ferroelectric sample Al2O3 was tested to evaluate whether the H2 production was caused by sonocatalysis. Negligible H2 production was observed under the same conditions. For comparison, the piezo-photocatalytic activity of BIT for H2 production was also measured under the same conditions, where a H2 production rate (45.9 μmol g−1 h−1) was obtained. Table 1 summarizes the H2 production performance achieved in this work and previous reports with that of many other piezo-photocatalysts. The results highlight the promising catalytic activity of BIT-Nd as a coupled piezo-photocatalyst in H2 generation, even in pure water, demonstrating its comparable performance to these systems with the addition of sacrificial agents.
Table 1 Comprehensive comparison of previously reported piezo-photocatalysts and this work for H2 evolution
|
Catalyst |
Electrolyte |
Sacrificial agents |
Conditions |
H2 evolution (μmol g−1 h−1) |
Ref. |
1 |
Pt/Bi4NbO8Br nanoplates |
H2O |
Lactic acid |
Ultrasonic: 40 kHz, 280 W; light: Visible light 300 W |
380 |
46
|
2 |
Bi4Ti2.8Cr0.1 Nb0.1O12/5%-g-C3N4 |
H2O |
Na2S |
Ultrasonic: 45 kHz; light: 300 W |
696 |
18
|
3 |
Na0.5Bi0.5TiO3 |
H2O |
Triethanolamine |
Ultrasonic: 40 kHz, 110 W; light: 200 mW cm−2 |
158 |
49
|
4 |
(Na0.5Bi0.5)TiO3–Ba(Ti0.5Ni0.5)O3 |
H2O |
Triethanolamine |
Ultrasonic: 40 kHz, 200 W; light: 300 W |
450 |
50
|
5 |
PbTiO3/CdS |
H2O |
Na2S and Na2SO3 |
Ultrasonic: 40 kHz, 100 W; light: 300 W |
849 |
51
|
6 |
KNbO3/MoS2 |
H2O |
Triethanolamine |
Ultrasonic: 40 kHz, 100 W; light: 300 W |
96 |
52
|
7 |
Au/AgNbO3 |
H2O |
FeSO4 |
Ultrasonic: 40 kHz, 110 W; light: Visible light 300 W |
392 |
53
|
8 |
PtRu/C3N5 |
H2O |
Triethanolamine |
Ultrasonic: 40 kHz, 120 W; light: 100 W |
1010 |
54
|
10 |
d-FCNs |
H2O |
Na2SO3 |
Ultrasonic: 40 kHz; light: visible light |
638.74 |
55
|
11 |
BIT-Nd |
H2O |
— |
Ultrasonic: 45 kHz, 200 W; light: 300 W |
1330.3 |
This work |
Fig. 5(g) displays the layered perovskite structure of BIT-Nd, composed of the bismuth oxide and perovskite-like layers stacked alternately along the c axis. The schematic diagrams illustrating the charge transfer behaviors in the photocatalysis and piezo-photocatlysis processes are shown in Fig. 5(h) and (i), respectively. For the photocatalysis, a large number of photo-induced electron–hole pairs are generated through photo-emission. However, the high recombination rate of electron–hole pairs limits their participation in the redox reaction at the surface of BIT-Nd, leading to a low photocatalytic performance in this state,45–48 as shown in Fig. 5(h). When the system is subjected to periodic mechanical stress and light, as shown in Fig. 5(i), the [TiO6] octahedron deforms and forms an internal electric field to promote the electron and hole transport in opposite directions. The introduction of piezoelectric polarization enables efficient charge separation and inhibits the recombination of photoinduced charges. Compared to BIT, the enhanced spectral absorption, larger piezoelectric potential under pressure, and faster charge transfer enable the BIT-Nd hierarchical microspheres to show a more excellent piezo-photocatalytic activity for efficient pollutant degradation and H2 production.
3. Conclusions
This paper presents a detailed evaluation of the piezo-photocatalytic properties of the Bi3.15Nd0.85Ti3O12 (BIT-Nd) nanosheet-assembled microspheres for high-efficiency hydrogen production and dye degradation for the first time. Due to the built-in electric field promoting the electron–hole pair separation, the H2 evolution rate of BIT-Nd reached 1330.3 μmol g−1 h−1 under ultrasonic conditions and light excitation without any cocatalyst and scavenger. Furthermore, in the process of degrading a 100 mL RhB solution with an initial concentration of 10 mg L−1, the piezo-photocatalytic process achieved a remarkable removal efficiency of 97.5% within 8 minutes, leading to an ultrahigh k value of 0.407 min−1, which surpasses the sum of the k values for the photocatalysis and piezocatalysis alone. The evaluation combines theoretical calculations with experimental results, which collectively reveal the positive effects of Nd3+ doping on improving the piezoelectric properties of BIT through lattice distortion and the reduction of the band gap via band structure engineering. At the same time, the thinner BIT-Nd nanosheets were easier to deform under the external forces and then produced higher piezoelectric fields. The hierarchical microspheres, higher piezoelectric potential, and efficient spectral absorption of the BIT-Nd nanosheet-assembled microspheres consistently determine the ultra-high piezo-photocatalytic performance. The lattice distortion and band structure engineering provide new insights for designing excellent piezo-photocatalysts.
4. Experimental
4.1 Chemicals and reagents
All chemicals and reagents were obtained commercially and used in the study without further purification, including analytical grade Bi2O3 (99.9%, Macklin), Nd2O3 (99.99%, Aladdin), TiO2 (99.0%, Macklin), tetrabutyl titanate (99.0%, Adamas), Bi(NO3)3·5H2O (≥99.0%, Aladdin), Nd(NO3)3·6H2O (99.0%, Macklin), and NaOH (≥98%, pellets, Macklin).
4.2 Synthesis of BIT and BIT-Nd hierarchical microspheres
Bi3.15Nd0.85Ti3O12 (BIT-Nd) and Bi4Ti3O12 (BIT) hierarchical microspheres were synthesized by a hydrothermal synthesis method. A certain amount of Bi(NO3)3·5H2O, and Nd(NO3)3·6H2O was added to the 6 mL tetrabutyl titanate solution with a Bi
:
Nd
:
Ti molar ratio of 3.15
:
0.85
:
3. Subsequently, a 70 mL NaOH solution with a concentration of 3 M was added to the above prepared precursor solution. The mixture was then continuously stirred magnetically for 0.5 h followed by transferring it into a 100 mL Teflon-lined stainless autoclave and heating at 200 °C for 24 h. The collected powders were filtered and washed with deionized water and ethanol at least three times and dried at 80 °C in air for 6 h to obtain the BIT-Nd hierarchical microspheres. BIT hierarchical microspheres were synthesized with a Bi
:
Ti molar ratio of 4
:
3 under the same synthesis conditions.
4.3 Characterization
The phase structures of the BIT-Nd and BIT hierarchical microspheres were evaluated by the X-ray diffraction technique (XRD, PAN-analytical Empyrean, Netherlands). The morphology was observed by scanning electron microscopy (SEM, Ultim Max 40, Tescan mira4 LMH), and energy dispersive spectroscopy (EDS) was used to characterize the element distribution. The nanostructures were observed by high-resolution transmission electron microscopy (HR-TEM, Titan G2 60–300, USA). The specific surface area was analyzed by the Brunauer–Emmett–Teller (BET, ASAP 2460, USA) method. X-ray photoelectron spectroscopy (XPS, ESCALAB 250Xi, USA) was carried out with standard Al Kα 1 excitation. The piezoelectric properties were evaluated by the contact mode piezo-response force microscopy (PFM, NanoMan VS, USA) characterization. The UV-vis absorbance spectra were obtained via a UV-vis spectrometer (UV-2000i and UV-2450, Shimadzu, Japan).
Electrochemical impedance spectroscopy (EIS), photocurrent measurement and Mott–Schottky curve measurements were performed using a standard three-electrode electrochemical workstation (CHI604E, China). The experimental setup comprised a three-electrode system, with a 0.5 M Na2SO4 solution serving as the electrolyte. The counter and reference electrodes employed were the Pt electrode and Ag/AgCl electrode, respectively, both immersed in a 0.5 M Na2SO4 solution. To prepare the working electrode, 20 mg of the catalyst was dispersed in a mixture of 50 μL of Nafion aqueous solution and 2 mL of ethylene glycol. The homogenized suspension was then coated onto a conductive fluorine-doped tin oxide glass substrate. For EIS measurements, a frequency range of 0.05 to 100 kHz was used. Photocurrent measurements were conducted on the working electrode, illuminated by a 300 W xenon lamp. The Mott–Schottky curves for the working electrode were obtained at frequencies of 1 kHz, 2 kHz, and 3 kHz.
For the ESR test, take a 5 mL glass bottle and add 1 mL of powder aqueous solution (for ·OH) or DMSO solution (for ·O2−). Quickly extract 50 μL of solution and sonicate or illuminate for 15 minutes. Mix this extraction solution and 5 μL DMPO thoroughly in a centrifuge tube. Then, use a capillary tube to extract the mixed solution and seal the bottom with plasticine. Make sure the surface is clean and place it in a glass tube for ESR testing on a Bruker model A300 spectrometer (Bruker A300, Germany).
4.4 Catalytic activity evaluation for hydrogen production
The piezo-, photo-, and piezo-photocatalytic performances of the BIT-Nd hierarchical microspheres for hydrogen production were measured. Different doses of the BIT-Nd powders were added to 70 mL of deionized (DI) water. A xenon lamp (300 W, PLS-SXE300E, Beijing Perfect Light) and ultrasonic machine (200 W, 45/80/100 kHz, KD-200, China) were used to provide a periodic mechanical load and simulated solar source, respectively. The amounts of generated H2 were measured by gas chromatography (Fanwei GC-6600, China) using ultra-pure N2 (99.9995 vol%) as the carrier gas and a thermal conductivity detector.
4.5 Catalytic activity evaluation for pollutant degradation
100 mg of the BIT-Nd and BIT powders were placed in 100 mL of RhB aqueous solution with different initial concentrations (C0 = 10 mg L−1, 20 mg L−1, and 30 mg L−1). The mixture was stirred for 30 minutes in the dark to achieve adsorption–desorption equilibrium between the dye and catalyst. The relative variations of RhB concentration over time in the photocatalytic, piezocatalytic and piezo-photocatalytic processes were evaluated, respectively. Specifically, the process of photocatalysis required a low stirring speed of 200 rpm to ensure that the active sites of powders were exposed to light, referred to “Stir200 + light”. For comparison, and as a control, the degradation ratio (D%) and the first-order kinetic rate constant (k) were calculated according to the following eqn (5) and (6): | Degradation rate (D%) = [1 − (C/C0)] × 100% | (5) |
where t is the reaction time, C and C0 are the instantaneous and original dye concentrations, respectively, and k can be obtained from the slope of the ln(C0/C) − t curve to determine the kinetics of the degradation process.
4.6 Finite element analysis
COMSOL Multiphysics® (combinations of solid mechanics, electrostatics, and electrical circuit modules) was used to set up a 3D finite-element model to analyze the piezoelectric potential.
4.7 DFT calculations
The density functional theory (DFT) was based on the utilization of the Cambridge sequential total energy package (CASTEP) in Material Studio software. The Perdew–Burke–Ernzerh (PBE) generalized gradient approximation (GGA) was chosen for exchange–correlation potential. The convergence tolerances of the energy, the maximum force and displacement were set as 1 × 10−5 eV, 0.03 eV Å−1 and 0.001 Å, respectively. A k-point grid of 6 × 6 × 1 was adopted for structure optimizations.
Author contributions
Yan Zhao: conceptualization, methodology, investigation, writing – original draft. Yan Zhang: supervision, conceptualization, writing – review & editing. Qianqian Xu: conceptualization, investigation. Hanyu Gong: data curation. Mingyang Yan: formal analysis. Kaiyu Feng: investigation. Xiang Zhou: software. Xuefan Zhou: conceptualization, writing – review & editing. Dou Zhang: conceptualization, supervision.
Conflicts of interest
The authors declare that they have no known competing financial interests or personal relationships that could have appeared to influence the work reported in this paper.
Acknowledgements
This work was supported by the National Key Research and Development Program (2022YFB3807404), the National Natural Science Foundation of China (No. 52302158), the Overseas Talent Introduction Project of China,the Hundred Youth Talents Program of Hunan and the State Key Laboratory of Powder Metallurgy, Central South University, Changsha, China.
References
- L. Chen, X. Dai, X. Li, J. Wang, H. Chen, X. Hu, H. Lin, Y. He, Y. Wu and M. Fan, J. Mater. Chem. A, 2021, 9, 13344–13354 RSC.
- Q. Liu, Q. Hu, D. Zhai, Q. Sun, H. Luo and D. Zhang, J. Mater. Chem. A, 2021, 9, 17841–17854 RSC.
- Q. Liu, F. Zhan, H. Luo, D. Zhai, Z. Xiao, Q. Sun, Q. Yi, Y. Yang and D. Zhang, Appl. Catal., B, 2022, 318, 121817–121831 CrossRef CAS.
- W. Feng, J. Yuan, F. Gao, B. Weng, W. Hu, Y. Lei, X. Huang, L. Yang, J. Shen, D. Xu, X. Zhang, P. Liu and S. Zhang, Nano Energy, 2020, 75, 104990–105001 CrossRef CAS.
- Y. Luo, L. Tang, U. Khan, Q. Yu, H.-M. Cheng, X. Zou and B. Liu, Nat. Commun., 2019, 10, 269–278 CrossRef PubMed.
- Q. Liu, F. Zhan, H. Luo, X. Luo, Q. Yi, Q. Sun, Z. Xiao, Y. Zhang, D. Zhang and C. R. R. Bowen, Adv. Funct. Mater., 2023, 230376–230391 Search PubMed.
- W. Liu, P. Wang, Y. Ao, J. Chen, X. Gao, B. Jia and T. Ma, Adv. Mater., 2022, 34, 2202508–2202516 CrossRef CAS PubMed.
- L. Pan, S. Sun, Y. Chen, P. Wang, J. Wang, X. Zhang, J. J. Zou and Z. L. Wang, Adv. Energy Mater., 2020, 10, 2000214–2000239 CrossRef CAS.
- Z. Liu, B. Wang, D. Chu and C. Cazorla, J. Mater. Chem. A, 2022, 10, 18132–18146 RSC.
- K. Wang, D. Shao, L. Zhang, Y. Zhou, H. Wang and W. Wang, J. Mater. Chem. A, 2019, 7, 20383–20389 RSC.
- X. Xue, W. Zang, P. Deng, Q. Wang, L. Xing, Y. Zhang and Z. L. Wang, Nano Energy, 2015, 13, 414–422 CrossRef CAS.
- X. Liu, L. Xiao, Y. Zhang and H. Sun, J. Materiomics, 2020, 6, 256–262 CrossRef.
- R. Tang, D. Gong, Y. Zhou, Y. Deng, C. Feng, S. Xiong, Y. Huang, G. Peng and L. Li, Appl. Catal., B, 2022, 303, 120929–120945 CrossRef CAS.
- Z. Chen, H. Jiang, W. Jin and C. Shi, Appl. Catal., B, 2016, 180, 698–706 CrossRef CAS.
- H. Irie, M. Miyayama and T. Kudo, J. Appl. Phys., 2001, 90, 4089–4094 CrossRef CAS.
- X. Liu, T. Wang, G. Li, G. Liu, J. Qiu, Z. Guo, H. Hao, J. Dong, H. Liu and J. Xing, J. Alloys Compd., 2023, 936, 168367–168377 CrossRef CAS.
- Q. Tang, J. Wu, X.-Z. Chen, R. Sanchis-Gual, A. Veciana, C. Franco, D. Kim, I. Surin, J. Pérez-Ramírez and M. Mattera, Nano Energy, 2023, 108202–108212 CrossRef CAS.
- J. Bai, J. Xiang, C. Chen and C. Guo, Chem. Eng. J., 2023, 456, 141095–141102 CrossRef CAS.
- S. Tu, H. Huang, T. Zhang and Y. Zhang, Appl. Catal., B, 2017, 219, 550–562 CrossRef CAS.
- X. Lin, Q. Guan, C. Zou, T. Liu, Y. Zhang, C. Liu and H. Zhai, Mater. Sci. Eng. B, 2013, 178, 520–526 CrossRef CAS.
- R. Nie, J. Yuan, Q. Chen, J. Xing, J. Zhu and W. Zhang, J. Am. Ceram. Soc., 2019, 102, 5432–5442 CrossRef CAS.
- U. Chon, J. S. Shim and H. M. Jang, Solid State Commun., 2004, 129, 465–468 CrossRef CAS.
- U. Chon, H. M. Jang, M. G. Kim and C. H. Chang, Phys. Rev. Lett., 2002, 89, 087601–087603 CrossRef PubMed.
- X. Xie, Z. Zhou, R. Liang and X. Dong, J. Materiomics, 2021, 7, 59–68 CrossRef.
- C. Gi Ppeum, C. H. O. Sam Yeon, J. Do Hyun, B. U. Sang Don, L. E. E. Gyoung Ja and L. E. E. Min Ku, New Phys.: Sae Mulli, 2015, 65, 657–663 Search PubMed.
- S. Tu, Y. Guo, Y. Zhang, C. Hu, T. Zhang, T. Ma and H. Huang, Adv. Funct. Mater., 2020, 30, 2005158–2005189 CrossRef CAS.
- W. Feng, J. Yuan, L. Zhang, W. Hu, Z. Wu, X. Wang, X. Huang, P. Liu and S. Zhang, Appl. Catal., B, 2020, 277, 119250–119260 CrossRef CAS.
- T. Patri, T. Durga Rao, K. S. K. R. Chandra Sekhar, S. Raghupathi Rao, S. Rayaprol and P. D. Babu, Phys. Status Solidi B, 2022, 259, 2200223–2200235 CrossRef CAS.
- X. Ruan, Y. Li, X. Wang and X. Yao, Ferroelectrics, 2010, 404, 119–126 CrossRef CAS.
- Z. Zhang, Q. Dong, P. Li, S. L. Fereja, J. Guo, Z. Fang, X. Zhang, K. Liu, Z. Chen and W. Chen, J. Phys. Chem. C, 2021, 125, 24814–24822 CrossRef CAS.
- H. Yu, F. Chen, X. Li, H. Huang, Q. Zhang, S. Su, K. Wang, E. Mao, B. Mei, G. Mul, T. Ma and Y. Zhang, Nat. Commun., 2021, 12, 4594–4604 CrossRef CAS PubMed.
- X. Zhou, Q. Sun, D. Zhai, G. Xue, H. Luo and D. Zhang, Nano Energy, 2021, 84, 1–12 Search PubMed.
- Z. Xie, X. Tang, J. Shi, Y. Wang, G. Yuan and J.-M. Liu, Nano Energy, 2022, 98, 107247–107258 CrossRef CAS.
- Q. Liu, F. Zhan, X. Luo, Q. Yi, Z. Xiao, D. Zhai, J. Huang, Y. Zhang, H. Luo, D. Zhang and C. R. Bowen, Nano Energy, 2023, 108, 108252–108266 CrossRef CAS.
- R. Xu, J. Karthik, A. R. Damodaran and L. W. Martin, Nat. Commun., 2014, 5, 1–7 Search PubMed.
- P. Mori-Sánchez, A. J. Cohen and W. Yang, Phys. Rev. Lett., 2008, 100, 146401–146404 CrossRef PubMed.
- H. Xiao, J. Tahir-Kheli and W. A. Goddard III, J. Phys. Chem. Lett., 2011, 2, 212–217 CrossRef CAS.
- J. Wu, N. Qin and D. Bao, Nano Energy, 2018, 45, 44–51 CrossRef CAS.
- H. Zheng, X. Li, K. Zhu, P. Liang, M. Wu, Y. Rao, R. Jian, F. Shi, J. Wang, K. Yan and J. Liu, Nano Energy, 2022, 93, 1–13 CrossRef.
- Y. Zhao, Q. Xu, X. Zhou, M. Yan, H. Gong, X. Yuan, H. Luo, K. Zhou, D. Zhang and C. Bowen, Ceram. Int., 2023, 49, 8259–8270 CrossRef CAS.
- Q. Liu, D. Zhai, Z. Xiao, C. Tang, Q. Sun, C. R. Bowen, H. Luo and D. Zhang, Nano Energy, 2022, 92, 1–14 Search PubMed.
- J. P. Ma, J. Ren, Y. M. Jia, Z. Wu, L. Chen, N. O. Haugen, H. T. Huang and Y. S. Liu, Nano Energy, 2019, 62, 376–383 CrossRef CAS.
- Q. Liu, F. Zhan, H. Luo, X. Luo, Q. Yi, Q. Sun, Z. Xiao, Y. Zhang, D. Zhang and C. R. Bowen, Adv. Funct. Mater., 2023, 33, 2303736 CrossRef CAS.
- Y. Zhang, P. T. T. Phuong, N. P. H. Duy, E. Roake, H. Khanbareh, M. Hopkins, X. Zhou, D. Zhang, K. Zhou and C. Bowen, Nanoscale Adv., 2021, 3, 1362–1374 RSC.
- S. Y. Lan, C. Yu, F. Sun, Y. X. Chen, D. Y. Chen, W. J. Mai and M. S. Zhu, Nano Energy, 2022, 93, 106792–106803 CrossRef CAS.
- C. Hu, H. Huang, F. Chen, Y. Zhang, H. Yu and T. Ma, Adv. Funct. Mater., 2020, 30, 1908168–1908178 CrossRef CAS.
- X. Zhou, F. Yan, S. Wu, B. Shen, H. Zeng and J. Zhai, Small, 2020, 16, 2001573–2001588 CrossRef CAS PubMed.
- H. You, Z. Wu, L. Zhang, Y. Ying, Y. Liu, L. Fei, X. Chen, Y. Jia, Y. Wang and F. Wang, Angew. Chem., 2019, 131, 11905–11910 CrossRef.
- Z. Zhao, L. Wei, S. Li, L. Zhu, Y. Su, Y. Liu, Y. Bu, Y. Lin, W. Liu and Z. Zhang, J. Mater. Chem. A, 2020, 8, 16238–16245 RSC.
- H. Xiao, W. Dong, Q. Zhao, F. Wang and Y. Guo, J. Hazard. Mater., 2021, 416, 125808–125816 CrossRef CAS PubMed.
- X. Huang, R. Lei, J. Yuan, F. Gao, C. Jiang, W. Feng, J. Zhuang and P. Liu, Appl. Catal., B, 2021, 282, 119586–119596 CrossRef CAS.
- S. Jia, Y. Su, B. Zhang, Z. Zhao, S. Li, Y. Zhang, P. Li, M. Xu and R. Ren, Nanoscale, 2019, 11, 7690–7700 RSC.
- S. Li, Z. Zhao, M. Liu, X. Liu, W. Huang, S. Sun, Y. Jiang, Y. Liu, J. Zhang and Z. Zhang, Nano Energy, 2022, 95, 107031–107043 CrossRef CAS.
- S. Shi, Y. Jiang, Y. Yu, M. Liang, Q. Bai, L. Wang, D. Yang, N. Sui and Z. Zhu, Adv. Funct. Mater., 2023, 33, 2210850–2210866 CrossRef CAS.
- Y. Wang, Y. Guo, C. Zeng, D. Yang, Y. Zhang, L. Wu, Y. Wu, J. Hao, J. Wang and R. Yang, Nano Energy, 2022, 104, 107983–110792 CrossRef CAS.
|
This journal is © The Royal Society of Chemistry 2024 |
Click here to see how this site uses Cookies. View our privacy policy here.