DOI:
10.1039/D3TA06755F
(Paper)
J. Mater. Chem. A, 2024,
12, 2359-2372
Two-dimensional trilayer heterostructures with cascade dual Z-schemes to achieve efficient hydrogen evolution reaction†
Received
5th November 2023
, Accepted 15th December 2023
First published on 15th December 2023
Abstract
Cascade dual Z-schemes for photocatalytic overall water splitting for hydrogen production are constructed for trilayer Bi/HfSeTe/ZrSe2, Bi/HfSeTe/ZrSe2, and InAs3/HfSeTe/ZrSe2 heterostructures. Electronic properties are studied by first-principles calculations, and the photoexcited carrier pathway is explored by nonadiabatic molecular dynamics simulations. The arrow-up, arrow-down, and cascade arrangement of band alignments for the Bi/HfSeTe/ZrSe2 and InAs3/HfSeTe/ZrSe2 heterostructures are explored, and those for the HfSe2/ZrSe2/HfSe2 and ZrSe2/HfSe2/ZrSe2 sandwich heterostructures are also considered for comparison. The solar-to-hydrogen efficiency of 22.08% for the bilayer HfSeTe/ZrSe2 heterostructure can be raised to 40.52%, 39.47%, and 41.04% by the cascade dual Z-schemes with Bi/HfSeTe/ZrSe2, Bi/HfSeTe/ZrSe2, and InAs3/HfSeTe/ZrSe2. They can further be boosted to 41.53%, 41.12%, and 43.57% under 1%, 1%, and −2% biaxial strains, respectively. The nonadiabatic molecular dynamics simulations reveal that the activity of the photogenerated carriers can be well protected. Moreover, the Gibbs free energy changes demonstrated that the hydrogen and oxygen evolution reactions driven by Bi/HfSeTe/ZrSe2 can spontaneously proceed. Therefore, the cascade dual Z-scheme provides an effective way to develop highly efficient photocatalysts for hydrogen generation from overall water splitting.
1. Introduction
Serious environmental and energy problems have arisen with the rapid economic development of countries around the world.1 The method of photocatalytic water splitting to produce hydrogen is gaining considerable attention due to its advantages of being inexpensive and non-polluting.2 Photocatalyst performance is promoted by a high carrier separation efficiency, wide light absorption range, and strong redox ability.3 Extensive efforts have been devoted to improving the photocatalytic ability of materials by enhancing the above factors. For example, constructing Janus monolayers with electrostatic potential difference (Δφ) on two surfaces is one of the most effective methods of facilitating internal carrier separation,4–6 such as group III chalcogenides,7 III2–VI3 groups,8 and group VI chalcogenides.9 Establishing heterostructures also has the above advantages and can further improve the overpotential of the hydrogen evolution reaction (HER) and oxygen evolution reaction (OER) compared with the monolayers composing them.10,11 Bilayer heterostructures can be classified into three types according to their different band structures. Type-I heterostructures have the straddling band structure characteristic, accumulating electrons and holes into the same components under light irradiation.12 For type-III heterostructures, the bandgap of one monolayer is broken by the other component.13 However, type-II heterostructures with staggered band structures are beneficial for efficient photocatalyst performance.14 Based on the different carrier migration pathways, type-II heterostructures can be divided into conventional type-II and direct Z-schemes. The conventional type-II scheme has excellent photogenerated carrier utilization capacity, but diminished redox capacity.15 Conversely, the direct Z-scheme can improve the redox capacity and have excellent light absorption performance that can drive HERs in the higher conduction band minimum (CBM) of two components and the OERs in the lower valence band maximum (VBM).16 Thus, constructing direct Z-scheme heterostructures is a smart choice to obtain efficient photocatalyst materials. For example, ZnIn2S4/MoSe2
17 with a direct Z-scheme fabricated through an experiment exhibits an optimized apparent quantum yield and a high hydrogen-production rate. In2O3/ZnInSe4,18 TiO2/ZnIn2S4,19 and Cd0.5Zn0.5S/BiVO4F
20 heterostructures display a more excellent photocatalyst performance than the monolayers composing them. The theoretically proposed PtTe2/Sb2S3,21 C7N6/Sc2CCl2,22 and C7N6/GaSnPS
23 with direct Z-schemes further demonstrate outstanding light absorption and carrier separation abilities. Dual Z-scheme heterostructures constructed from three monolayers with a narrow bandgap can achieve a wide light absorption range, which is one of the crucial factors for enhancing the photocatalyst performance. Generally, the dual Z-scheme of trilayer heterostructures is divided into arrow-up,24 arrow-down,25 and cascade26,27 according to the characteristics of band alignments. Considering that the HER and OER can occur in nonadjacent monolayers in dual Z-scheme heterostructures, the lifetime of photogenerated carriers in a trilayer heterostructure is prolonged, which is conducive to suppressing the recombination of carriers.28,29 Meanwhile, the shape and size of different materials can be controlled, resulting in the large specific surface area and the high photocatalytic activity of a trilayer heterostructure. Carrier migration mechanisms with different types of band alignments have been suggested in some experimental reports, such as the V2O5/CdS/CoS2,30 BiSi/Bi2WO6/g-C3N4,31 and AgI/g-C3N4/Bi2WO6
32 trilayer heterostructures. However, they have not been confirmed theoretically. Therefore, intensive theoretical studies of the carrier migration mechanism of trilayer heterostructures are urgently needed.
Two-dimensional (2D) transition metal dichalcogenide HfSe2 and ZrSe2 monolayers reportedly have attractive electronic and optical properties.33–35 For example, the HfSe2/ZrSe2 heterostructure has been reported to have a type-I band alignment, and the potential of CBMs cannot straddle the hydrogen reduction potential (−4.44 eV).36 We have first constructed sandwich heterostructures by stacking37,38 and doping11,39 methods, which have turned out to be effective measures to regulate band alignment. However, the working CBM of HfSe2/ZrSe2/HfSe2 and ZrSe2/HfSe2/ZrSe2 configurations cannot match the HER condition. The band alignment of the HfSe2/ZrSe2 heterostructure can be changed to type II by doping Te atoms into the HfSe2 monolayer. We have also considered two different configurations of the HfSeTe/ZrSe2 heterostructures. However, we have found that only the HfSeTe/ZrSe2-I heterostructure can straddle the potentials of the HER and OER, whereas the overpotential for the HER is only 0.03 eV even though the η′STH is 22.08%. Subsequently, four sandwich configurations constructed from the HfSeTe and ZrSe2 monolayers have also been explored, whereas their band alignment types are arrow-up or arrow-down. The CBMs and VBMs cannot fully match the potential conditions of HERs or OERs. In the present study, we constructed eight different configurations of 2D trilayer heterostructures by adding the Bi or InAs3 monolayer on the bilayer HfSeTe/ZrSe2 heterostructure. As expected, the Bi/HfSeTe/ZrSe2-I, Bi/HfSeTe/ZrSe2-II, and InAs3/HfSeTe/ZrSe2-I configurations had cascade band alignment, by which we constructed cascade dual Z-schemes. The obtained schemes also satisfied the potential requirements of the HER and OER. The η′STH's on Bi/HfSeTe/ZrSe2-I, Bi/HfSeTe/ZrSe2-II, and InAs3/HfSeTe/ZrSe2-I can reach 40.52%, 39.47%, and 41.04%, respectively. The η′STH was further boosted to 41.53%, 41.12%, and 43.57% under 1%, 1%, and −2% biaxial strain, respectively. Additionally, the protection of the redox ability in cascade dual Z-scheme heterostructures is demonstrated using the carrier transfer speeds through non-adiabatic molecular dynamics (NAMD)40 simulations. The Gibbs free energy changes (ΔG) indicated that HERs and OERs were thermodynamically feasible. Remarkably, the HER and OER with Bi/HfSeTe/ZrSe2-II can proceed spontaneously in a neutral environment because the potential energies of the photogenerated electrons and holes were larger than the ΔG's in the HER and OER, respectively. Therefore, these trilayer heterostructures had competitive potential to achieve high STH efficiency through the cascade dual Z-scheme.
2. Computational methods
The HfSeTe monolayer was constructed by replacing all Se atoms on the same side of the HfSe2 monolayer with Te atoms. The present HfSeTe, ZrSe2, and InAs3 monolayers were with the symmetry of the p3m1 space group, and the Bi monolayer belonged to the p6m2 space group, as shown in Fig. S1.† Trilayer heterostructures were constructed by adding the third monolayer to the top or bottom of HfSe2/ZrSe2 or HfSeTe/ZrSe2 bilayer heterostructures. For all heterostructures containing the HfSeTe monolayer, we also considered two different configurations (HfSeTe monolayer rotating 180° along the y direction), which were represented by HfSeTe/ZrSe2-I, HfSeTe/ZrSe2-II, ZrSe2/HfSeTe/ZrSe2-I, HfSeTe/ZrSe2/HfSeTe-II, HfSeTe/ZrSe2/HfSeTe-III, ZrSe2/HfSeTe/ZrSe2, Bi/HfSeTe/ZrSe2-I, Bi/HfSeTe/ZrSe2-II, HfSeTe/ZrSe2/Bi-I, HfSeTe/ZrSe2/Bi-II, InAs3/HfSeTe/ZrSe2-I, InAs3/HfSeTe/ZrSe2-II, HfSeTe/ZrSe2/InAs3-I, and HfSeTe/ZrSe2/InAs3-II. The interlayer distances (d) and formation energies (Ef) for 16 configurations are listed in Table S1.†
All calculations for the geometrical optimization and electronic and optical properties were realized in the Vienna ab intitio simulation package (VASP).41 The projector augmented wave42 pseudopotentials in combination with the generalized gradient approximation with Perdew–Burke–Ernzerhof43 and Heyd–Scuseria–Ernzerhof (HSE06)44 hybrid functionals were used. The dipole correction was added along the z direction in all calculations to correct the potential and force because of the asymmetric monolayers in the heterostructures. The cutoff energy was used at 550 eV for the plane wave. The density functional dispersion correction (DFT-D4)45,46 was used to describe the van der Waals interactions. The energy and force convergence standards were selected as 10−6 eV and 0.01 eV Å−1, respectively. Geometric structures were optimized and static calculations of electronic properties were performed using HSE06 with a 3 × 3 × 1 and 5 × 5 × 1 k-mesh, respectively. Ab initio molecular dynamics (AIMD) simulations for the screened structures lasting 10 ps at 300 K based on the Nose–Hoover thermostat and a canonical ensemble (NVT)47,48 were conducted to confirm the thermodynamic stability of the heterostructures. NAMD simulations combined with decoherence-induced surface hopping (DISH)49 methods implemented in the Hefei-NAMD code40 were used to study the migration pathway and lifetimes of the photogenerated carriers. For the DISH simulation, we selected 100 random initial configurations and sampled 2000 trajectories, expanding the simulation time to 10 ps.
The calculation methods and details for the η′STH's, optical absorption, carrier mobility, ΔG's in the HER and OER, and NAMD simulation are presented in the ESI.†
3. Results and discussion
3.1 Bilayer heterostructures composed of HfSe2 and ZrSe2 monolayers
HfSe2 and ZrSe2 monolayers were prepared in the experiment and showed excellent thermal and optical properties,33,50 whereas the HfSe2/ZrSe2 heterostructure36 was found to have a type-I band alignment. The CBM and VBM contributed by the ZrSe2 monolayer did not match the overall water splitting conditions. To manipulate the photocatalyst performance, we constructed HfSe2/ZrSe2/HfSe2 and ZrSe2/HfSe2/ZrSe2 sandwich configurations, as shown in Fig. 1a and d. The negative formation energies (Ef's) of −35.74 and −37.82 meV Å−2 indicated that they were stable in energy. The band structures and projected density of states (PDOS) are presented in Fig. 1b and e. They showed that the band of the sandwich configurations changed into a dual type-I arrangement. The charge transfer between the adjacent monolayers was decided by the direction of the built-in electron field (Ein)51 through Bader charge analysis52 and plane-integrated charge density difference (Δρ). As shown in Fig. S1a,† the charge transfer of 0.0012 and 0.0016 |e| occurs from the bottom and top HfSe2 to the ZrSe2 monolayer, together with the accumulated electrons on both sides of the ZrSe2 monolayers, given by Δρs, indicating that the Ein directions between the two interfaces of the HfSe2/ZrSe2/HfSe2 heterostructure were from the HfSe2 to the ZrSe2 monolayers. As shown in Fig. 1c, the photogenerated electrons in the CBM of ZrSe2 transferred into the CBM of HfSe2 monolayers, whereas the holes in the VBM of HfSe2 transferred into the VBM of the ZrSe2 monolayer. Therefore, the HER occurred in the CBMs of the two HfSe2 monolayers, and the OER was driven by the middle ZrSe2 monolayer.
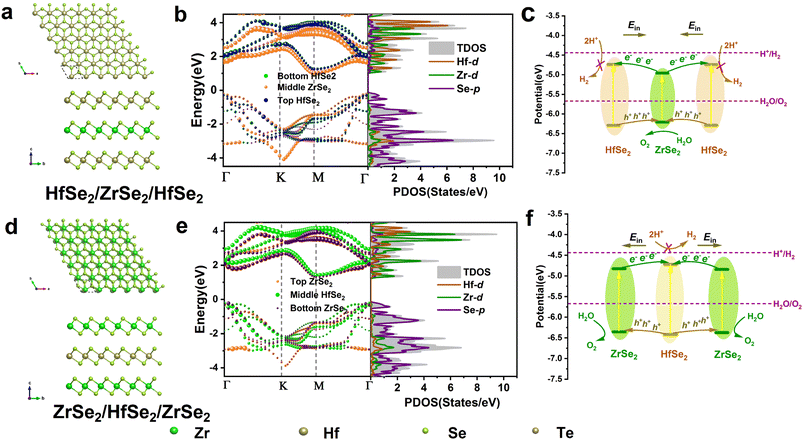 |
| Fig. 1 The geometric structures, band alignments, projected density of states, and the schemes for HERs and OERs. (a)–(c) HfSe2/ZrSe2/HfSe2, and (d)–(f) ZrSe2/HfSe2/ZrSe2. | |
For ZrSe2/HfSe2/ZrSe2, Fig. S1b† demonstrates that 0.0015 and 0.0014|e| transferred from the two ZrSe2 monolayers into the HfSe2 one. Combining the band alignment and Ein at the two interfaces, the photogenerated electrons in the CBMs of the two ZrSe2 monolayers were found to spontaneously transfer into the CBM of the HfSe2 monolayer, whereas the holes in the VBM of the HfSe2 monolayer transferred into the VBMs of the two ZrSe2 monolayers (Fig. 1f). Therefore, the electrons in the CBM of the HfSe2 and the holes in the VBM of the ZrSe2 monolayers proceeded to undergo the HER and OER, respectively.
The absolute band edges can be evaluated by the following equations:
| EAbsCBM = EHSE06CBM − Vvac | (1) |
| EAbsVBM = EHSE06VBM − Vvac | (2) |
where
EHSE06CBM/
EHSE06CBM represent the energies of the CBM and VBM calculated by the HSE06 functional, respectively, and
Vvac represents the values obtained from the electrostatic potential plots, as shown in Fig. S2 and S3.
† Unfortunately, the
EAbsCBM's cannot match the HER condition, although the
EAbsVBM's of the HfSe
2/ZrSe
2/HfSe
2 and ZrSe
2/HfSe
2/ZrSe
2 configurations can satisfy the OER requirement.
3.2 Bilayer HfSeTe/ZrSe2 and trilayer HfSeTe/ZrSe2/HfSeTe and ZrSe2/HfSeTe/ZrSe2 sandwich heterostructures
The electronic properties of monolayers and heterostructures can be significantly controlled by doped atoms.39,53 Thus, the heterostructure comprising the Te-doped HfSe2 monolayer, i.e., HfSeTe, was explored. The geometric structure, band alignment, and PDOS of the HfSeTe monolayer are shown in Fig. S4a and b.† The present direct bandgap of 0.39 eV of the HfSeTe monolayer was close to the previous ones of 0.44
54 and 0.47 eV,55 confirming that the present theoretical level was reliable. The HfSeTe monolayer can also be synthesized by chemical vapor deposition or conventional flux methods experimentally, such as the fabrication of MoSSe
56 and MoSeTe.57 Accordingly, we constructed bilayer and trilayer heterostructures by using the HfSeTe and ZrSe2 monolayers. Nowadays, the MoSSe/MoS2,58 MoS2/WSe2,59 and ZrSe2/MoSe2
60 heterostructures are synthesized by mechanical exfoliation, chemical vapor deposition, and dry transfer methods, so the bilayer heterostructure of HfSeTe/ZrSe2 may be synthesized in the same ways because of the similar structures. We considered two configurations of the HfSeTe/ZrSe2 heterostructure, as shown in Fig. 2a and d. The negative Ef's of −21.65 and −19.48 meV Å−2 meant that the HfSeTe/ZrSe2 heterostructures can be synthesized through an exothermic process. The insignificant deformation of the geometric structures in the AIMD simulations in Fig. S5a and b† indicated that they were thermodynamically stable. Staggered band alignments were observed for the HfSeTe/ZrSe2-I and HfSeTe/ZrSe2-II configurations, as shown in Fig. 2b and e. The Ein directions from HfSeTe to the ZrSe2 monolayer in the heterostructures can propel the electrons transferring from the ZrSe2 into the HfSeTe monolayer, as shown in Fig. S1c and d.† Therefore, the photogenerated electrons in the CBM of the ZrSe2 monolayer recombined with the holes in the VBM@HfSeTe. As a result, direct Z-schemes formed with the HER proceeding on the CBM@HfSeTe and the OER on the VBM@ZrSe2, respectively. However, as presented in Fig. 2c and f, the CBM of HfSeTe/ZrSe2-II cannot satisfy the HER condition, whereas the HER overpotential of 0.03 eV for HfSeTe/ZrSe2-I implied a weak reduction ability.
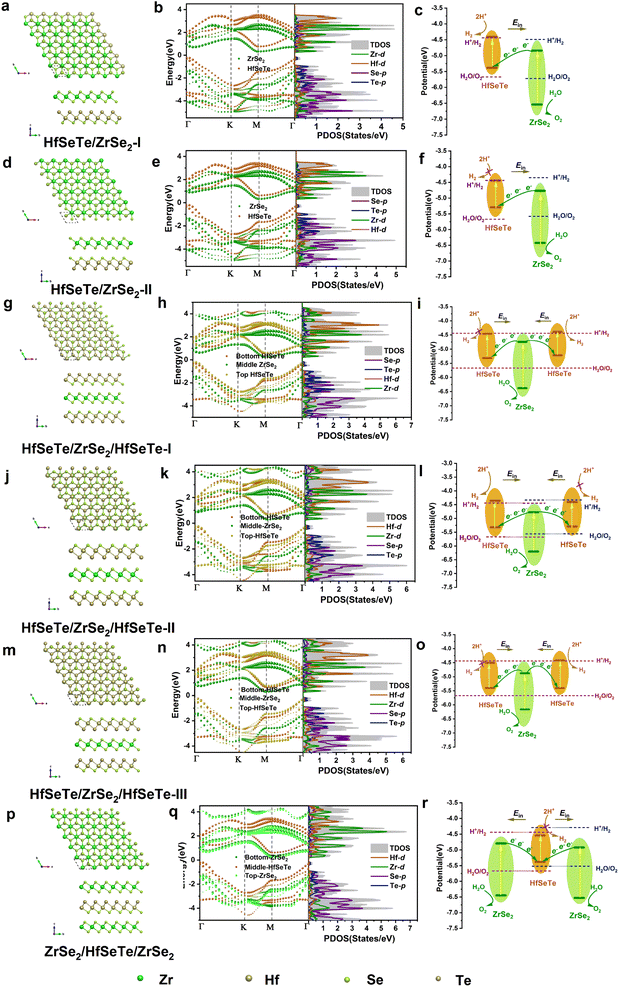 |
| Fig. 2 The geometric structures, band alignments, project density of states, and the schemes for HERs and OERs. (a)–(c) HfSeTe/ZrSe2-I, (d)–(f) HfSeTe/ZrSe2-II, (g)–(i) HfSeTe/ZrSe2/HfSeTe-I, (j)–(l) HfSeTe/ZrSe2/HfSeTe-II, (m)–(o) HfSeTe/ZrSe2/HfSeTe-III, and (p)–(r) ZrSe2/HfSeTe/ZrSe2. | |
Furthermore, three sandwich configurations of HfSeTe/ZrSe2/HfSeTe-I, HfSeTe/ZrSe2/HfSeTe-II, and HfSeTe/ZrSe2/HfSeTe-III were considered, as shown in Fig. 2g, j and m. The band alignments in Fig. 2h, k and n showed a staggered and arrow-down feature, whereas the directions of Ein between the adjacent monolayers were from the HfSeTe to the ZrSe2 monolayers. As shown in Fig. 2i, l and o, all three configurations were suitable for constructing a dual Z-scheme with HERs proceeding on the HfSeTe monolayers and OERs on the middle ZrSe2 monolayer. Unlike the single Vvac's for HfSeTe/ZrSe2/HfSeTe-I and HfSeTe/ZrSe2/HfSeTe-III, the HfSeTe/ZrSe2/HfSeTe-II had different vacuum energy levels on the two surfaces. Thus, we evaluated EAbsCBM and EAbsVBM with different Vvac's according to eqn (1) and (2). Results demonstrated that only one of the two CBMs relative to the two HfSeTe monolayers can straddle the hydrogen reduction potential, although all VBMs of the three configurations can drive the OER. Consequently, these heterostructures were not considered in the following discussion because they were similar to the bilayer heterostructures discussed in the above sections.
The trilayer sandwich configuration ZrSe2/HfSeTe/ZrSe2 had a staggered arrow-up type band alignment, and the directions of Ein were also from the HfSeTe into the ZrSe2 monolayer, as shown in Fig. 2q and S1h.† The photocatalytic dual Z-scheme with the HER on CBM@HfSeTe and the OER on both ZrSe2 monolayers is shown in Fig. 2r. However, CBMs@HfSeTe in Table S1,† regardless of any one of the two Vvac's, failed to match the HER condition of −4.44 eV. Therefore, these sandwich configurations cannot drive the HER. The sandwich configurations, which were relatively more easily prepared than the trilayer heterostructures with three different monolayers, conferred difficulty in achieving an efficient photocatalytic overall water-splitting performance, although the dual Z-scheme can be constructed for some of them.
3.3 Dual Z-scheme heterostructures comprising Bi/InAs3, HfSeTe, and ZrSe2 monolayers
Trilayer Bi/HfSeTe/ZrSe2 and InAs3/HfSeTe/ZrSe2 heterostructures were constructed by adding the InAs3 or Bi monolayer onto the bilayer HfSeTe/ZrSe2 heterostructure, respectively. The synthesis procedure similar to the bilayer heterostructures can also be used to prepare trilayer heterostructures, as demonstrated by the preparations of the BiFeO3/g-C3N4/WO3,24 Ag3PO4/AgBr/g-C3N4,28 and V2O5/CdS/CoS2 heterostructures.30 Thus, the present Bi/HfSeTe/ZrSe2 and InAs3/HfSeTe/ZrSe2 heterostructures may be fabricated in a similar way. The InAs3 and Bi monolayers optimized and calculated by HSE06 are shown in Fig. S4e–h.† The lattice constant and bandgap of 4.22 Å and 1.02 eV for the Bi monolayer approached the theoretical 4.24 Å and 1.16 eV obtained by Singh et al.,61 whereas those for the InAs3 monolayer were 7.85 Å and 1.19 eV, respectively. The CBMs of the two monolayers can match the HER condition, but the VBMs cannot satisfy the OER one, as demonstrated in Table S2.† Considering that the primary cells of the two monolayers substantially differed from the bilayer HfSeTe/ZrSe2 heterostructures, we constructed trilayer heterostructures by using the 2 × 2 supercell of the HfSeTe/ZrSe2 heterostructures and the √3 × √3 supercell of Bi or the primary cell of the InAs3 monolayer. As a result, the lattice mismatch ratios of the Bi/HfSeTe/ZrSe2 and InAs3/HfSeTe/ZrSe2 heterostructures were <1.5%.
Eight different configurations were considered and represented by Bi/HfSeTe/ZrSe2-I, Bi/HfSeTe/ZrSe2-II, HfSeTe/ZrSe2/Bi-I, HfSeTe/ZrSe2/Bi-II, InAs3/HfSeTe/ZrSe2-I, InAs3/HfSeTe/ZrSe2-II, HfSeTe/ZrSe2/InAs3-I, and HfSeTe/ZrSe2/InAs3-II. Their geometric structures, band structures, and carrier migration pathways are shown in Fig. S7 and S3.† The negative Ef's in Table S1† demonstrated that the heterostructures are stable in energy. The band alignments of HfSeTe/ZrSe2/Bi-I, HfSeTe/ZrSe2/Bi-II, and HfSeTe/ZrSe2/InAs3-I were arrow-down, whereas those of HfSeTe/ZrSe2/InAs3-II were arrow-up (Fig. S7b, e, k, n†). The InAs3/HfSeTe/ZrSe2-II band alignment was the type-I and type-II on the two interfaces, respectively (Fig. S7h†), thereby losing the conditions necessary to form a dual Z-scheme. The Ein directions were from the bottom and top layers to the middle layer in the above five configurations, as shown in Fig. S1k, l, n, o and p.† The photocatalytic dual Z-schemes for HfSeTe/ZrSe2/Bi-I, HfSeTe/ZrSe2/Bi-II, and HfSeTe/ZrSe2/InAs3-I are shown in Fig. S7c, f and l.† Unfortunately, CBMs@HfSeTe cannot match the HER condition for the three trilayer heterostructures, although the VBMs in the middle layer satisfied the OER requirement. As shown in Fig. S7i,† CBM@ZrSe2 in InAs3/HfSeTe/ZrSe2-II cannot meet the HER condition. As for the photocatalytic scheme of HfSeTe/ZrSe2/InAs3-II, the electrons in CBM@ZrSe2 transferred into the CBMs of the InAs3 and HfSeTe monolayers, and the holes in the VBM of the InAs3 and HfSeTe transferred into VBMs@ZrSe2, as presented in Fig. S7o.† Thus, the carrier migration in HfSeTe/ZrSe2/InAs3-II was a dual conventional type-II scheme rather than a dual Z-scheme. However, CBM@HfSeTe and VBM@ZrSe2 cannot straddle the H+/H2 and H2O/O2 potentials, respectively. As a result, HfSeTe/ZrSe2/Bi-I, HfSeTe/ZrSe2/Bi-II, InAs3/HfSeTe/ZrSe2-II, HfSeTe/ZrSe2/InAs3-I, and HfSeTe/ZrSe2/InAs3-II cannot drive overall photocatalytic water splitting.
Notably, cascade band alignments were observed for Bi/HfSeTe/ZrSe2-I, Bi/HfSeTe/ZrSe2-II, and InAs3/HfSeTe/ZrSe2-I, as shown in Fig. 3b, e and h. Results of Bader charge analysis and Δρ in Fig. S1i, j and m† demonstrated that the Ein directions at the two interfaces of the heterostructures were arranged as bottom → middle → top monolayers. Accordingly, the electrons in CBM@ZrSe2 recombined with the holes in VBM@HfSeTe, and the electrons in CBM@HfSeTe recombined with the holes in VBM@Bi or VBM@InAs3. The outcome was the formation of a cascade dual Z-scheme, as shown in Fig. 3c, f and i. Therefore, the HER and OER were driven by CBM@Bi or CBM@InAs3 and VBM@ZrSe2, and all band edges of Bi/HfSeTe/ZrSe2-I, Bi/HfSeTe/ZrSe2-II, and InAs3/HfSeTe/ZrSe2-I can meet the conditions of the HER and OER, respectively. The working CBMs for HERs on Bi/HfSeTe/ZrSe2-I and Bi/HfSeTe/ZrSe2-II had larger overpotentials than those of InAs3/HfSeTe/ZrSe2-I, whereas their overpotentials for the OER were smaller than those of InAs3/HfSeTe/ZrSe2-II. As shown in Fig. S5c–e,† AIMD simulations at 300 K demonstrated that the three trilayer heterostructures were thermodynamically stable. Moreover, the stability of the considered heterostructures in the solution was explored by optimizing the geometric structures of the Bi/HfSeTe/ZrSe2-I, Bi/HfSeTe/ZrSe2-II, and InAs3/HfSeTe/ZrSe2-II trilayer heterostructures in the solution environments of water, formic acid, and acetone solvents using VASPsol,62 respectively. As shown in Fig. S6,† the structures of the three configurations were obviously unchanged in the three neutral, acidic, and alkaline solutions, respectively. The solvation energy (ΔEsol) of the three trilayer heterostructures, summarized in Table S3,† was defined by ΔEsol = Esol − Evac. In this equation, Esol and Evac represent the total energy of the heterostructures with and without solution, respectively. The negative ΔEsol's of the three trilayer heterostructures indicated that they were also stable in the solutions. Accordingly, we further explored the photocatalyst performance of Bi/HfSeTe/ZrSe2-I, Bi/HfSeTe/ZrSe2-II, and InAs3/HfSeTe/ZrSe2-I.
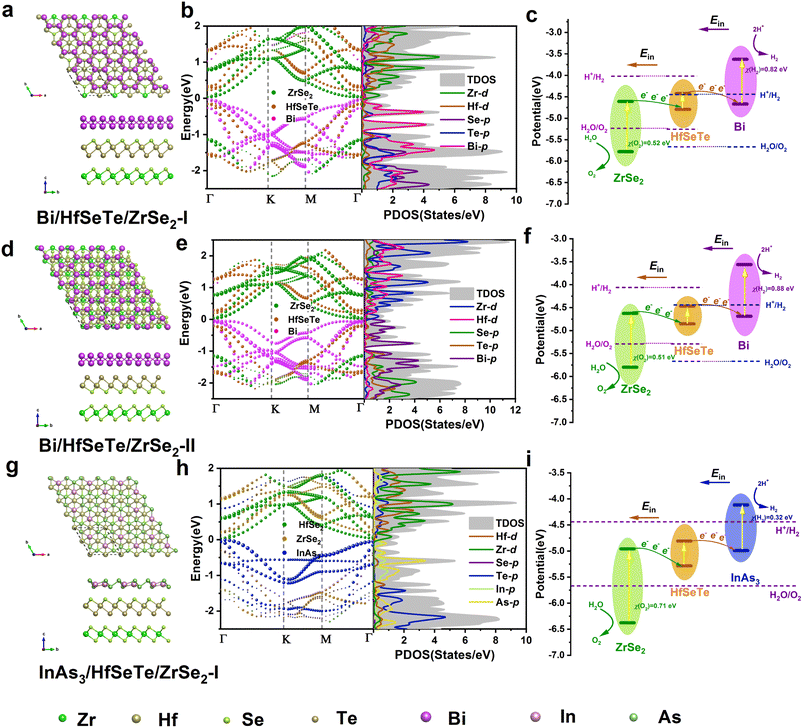 |
| Fig. 3 The geometric structures, band alignments, project density of states, and the schemes for HERs and OERs. (a)–(c) Bi/HfSeTe/ZrSe2-I, (d)–(f) Bi/HfSeTe/ZrSe2-II, and (g)–(i) InAs3/HfSeTe/ZrSe2-I. | |
3.4 STH conversion efficiencies and effects of strain engineering
The STH conversion efficiencies η′STH's of the three trilayer heterostructures can be calculated by63 | 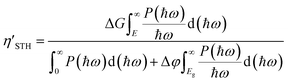 | (3) |
where P (ℏω) is the AM1.5 G solar-energy flux at the photoenergy hω, Eg is the larger one of the bandgaps projected on the monolayers, and ΔG is the potential difference of 1.23 eV for water splitting. Δφ is the electrostatic potential difference between the two outer surfaces, and E is the energy of photos that can actually be utilized for water splitting and evaluated by | 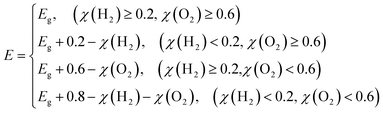 | (4) |
The η′STH's for the three trilayer heterostructures Bi/HfSeTe/ZrSe2-II, Bi/HfSeTe/ZrSe2-II, and InAs3/HfSeTe/ZrSe2-I were 40.52%, 39.47%, and 41.04%, respectively. They were much higher than the 22.08% of the corresponding bilayer HfSeTe/ZrSe2-I. According to eqn (S4)–(S7),† the smaller value of the maximum one of the bandgaps of monolayers and the larger overpotentials for the HER and OER were responsible for the high η′STH. For the present trilayer heterostructures, the narrower bandgaps were the dominant factor causing the higher η′STH than that of the bilayer one because the overpotentials of the working CBMs and VBMs of the trilayer heterostructures did not substantially increase. Notably, the η′STH's of Bi/HfSeTe/ZrSe2-I, Bi/HfSeTe/ZrSe2-II, and InAs3/HfSeTe/ZrSe2-II were much larger than those of ZrS2/MoSSe (6.4%),64 HfSe2/InSe (25.24%),15 and AlP3/GaP3 (16.89%).65 Moreover, the η′STH can also be compared with the experimental data if the AQE and experimental STH conversion efficiency (STHexp) are available in the literature,66 although the ideal quantum efficiency was used in eqn (3). For example, the GaN:Mg/InGaN:Mg nanowires67 and Rh/Cr2O3/Co3O4-loaded InGaN/GaN nanowires68 had STHexp's of 3.3% and 9% corresponding to the η′STH's of 7.5% and 17.7%, respectively. They were apparently lower than those of the present trilayer heterostructures.
As shown in Fig. 4a, the Bi/HfSeTe/ZrSe2-II and InAs3/HfSeTe/ZrSe2-I heterostructures can maintain stability within the range of −3% to +4% biaxial strain because their strain energy Es showed a quadratic character. The deformations of Bi/HfSeTe/ZrSe2-I cannot maintain elasticity under −3% compressed strain, and only the range of −2% to +4% strains was considered for this heterostructure. As shown in Fig. 4b–d and listed in Tables S4–S6,† the bandgaps and overpotentials changed under the strains. As a result, the η′STH increased to 41.53% and 41.12% for Bi/HfSeTe/ZrSe2-I and Bi/HfSeTe/ZrSe2-II under 1% biaxial strain, respectively, whereas the other strains reduced the η′STH's because of the increased bandgap of the monolayers and the decreased overpotentials of HERs and OERs. For the same reasons, the η′STH of InAs3/HfSeTe/ZrSe-I was boosted under −3% to −1% compressed strains but decreased under the tensile strains. The maximum η′STH of 43.57% for InAs3/HfSeTe/ZrSe-I can be achieved under −2% compressed strains. Overall, most of the considered strains resulted in obviously decreased η′STH, although smaller lifts were also observed for the light strains. Therefore, heavy strains, regardless of being compressive or tensile, should be avoided to maintain the high η′STH's of the present trilayer heterostructures.
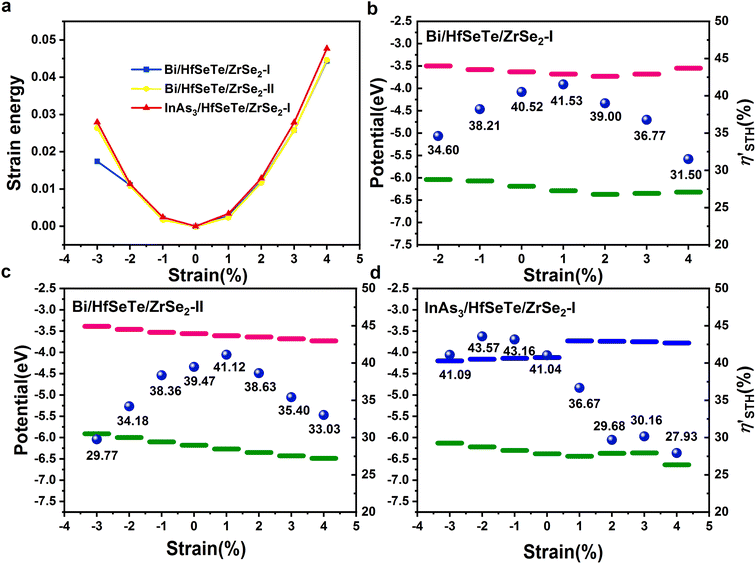 |
| Fig. 4 The strain engineering for heterostructures. (a) The strain energies for Bi/HfSeTe/ZrSe2-I, Bi/HfSeTe/ZrSe2-II, and InAs3/HfSeTe/ZrSe2-I. (b)–(d) The potentials of band edges and STH conversion efficiency η′STH of Bi/HfSeTe/ZrSe2-I, Bi/HfSeTe/ZrSe2-II, and InAs3/HfSeTe/ZrSe2-I under biaxial strains. The blue balls represent η′STH. | |
3.5 Dynamics of photogenerated carriers for cascade dual Z-scheme heterostructures
To confirm the photocatalyst performance of Bi/HfSeTe/ZrSe2-I, Bi/HfSeTe/ZrSe2-II, and InAs3/HfSeTe/ZrSe2-I, we performed NAMD simulations by using the Hefei-NAMD40 code (the details in ESI†) which has been used to analyze the carrier dynamics theoretically for the experimental observations of MoS2/MoSe2,69,70 ReSe2/MoS2,71,72 and MoS2/WS2.73,74 As shown in Fig. 5a–c, nine different processes occurred in the trilayer heterostructure, including the electron transfer (①②), hole transfer (③④), interlayer e–h recombination (⑤⑥), and intralayer e–h recombination (⑦⑧⑨). Fig. 6 demonstrates that carriers tended to transfer into the adjacent states according to the evolution of the spatial distributions and the average energies of the carriers in each CBM and VBM of the three monolayers at 300 K for 10 ps based on the DISH.49 Moreover, the carrier energy relaxation processes in the three trilayer heterostructures had different time scales, which can be understood through the nonadiabatic coupling (NAC) elements between different states.75,76 In general, a large NAC corresponds to a higher hopping probability. According to eqn (S9),† a larger electron–phonon (e–p) coupling, a smaller energy gap, and a faster velocity of nuclei lead to a larger NAC matrix element. As shown in Fig. 5d–f, the Fourier transforms of the normalized autocorrelation function (ACF) for the fluctuations of the energy difference between the initial and final states were calculated and used to estimate the contribution of e–p coupling and nuclear velocity.70 The low frequency and weak intensity peaks in the Fourier energy spectrum dominated the slow dephasing process,77,78 and the long pure-dephasing time was also beneficial for ultrafast carrier transfer. As shown in Fig. S8 and S9,† the low-frequency modes were similar among the six processes in Bi/HfSeTe/ZrSe2-I and Bi/HfSeTe/ZrSe2-II. The peaks at 66 and 103 cm−1 correspond to the out-of-plane vibrations of the Hf–Se and Hf–Te bonds; the peaks at 140 and 202 cm−1 correspond to the out-of-plane vibration of Se, Te, Hf, and Zr atoms; and the side peaks near 262 cm−1 correspond to the vibration of Se and Zr atoms in the ZrSe2 monolayer. This finding demonstrated their approximately equal nuclear velocities. As shown in Fig. S10,† all low-frequency vibration modes observed for the six processes in InAs3/HfSeTe/ZrSe2-I were contributed by the out-of-plane vibrations of all bonds, which increased the e–p coupling, causing fast carrier transfer.
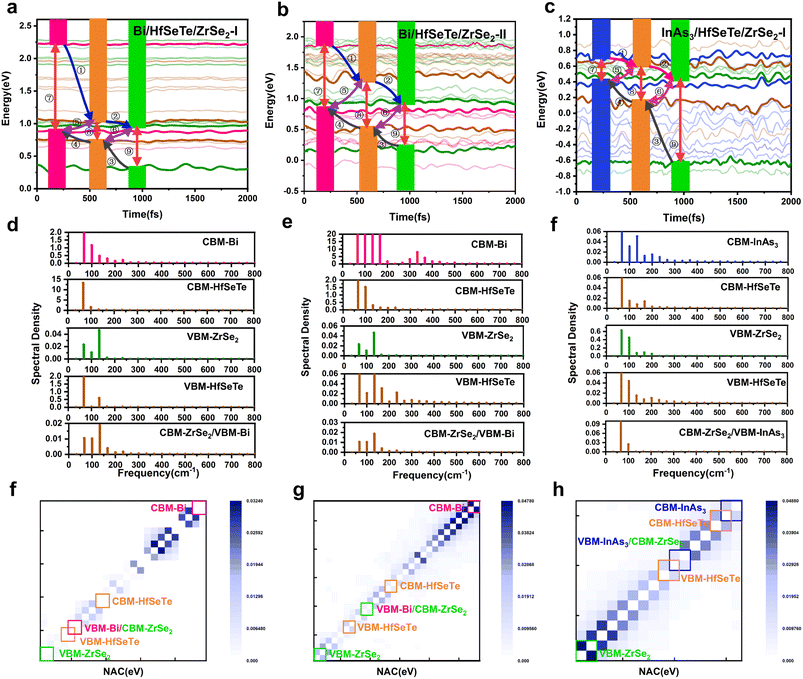 |
| Fig. 5 The time-dependent evolution of the Kohn–Sham orbital, Fourier transforms of normalized ACF of the energy difference fluctuation between the initial and final states, and averaged values of NAC between different states for (a), (d) and (f) Bi/HfSeTe/ZrSe2-I, (b), (e) and (g) Bi/HfSeTe/ZrSe2-II, and (c), (f) and (h) InAs3/HfSeTe/ZrSe2-I. The purplish red, orange, and green lines in (a), (b), and (c) represent the states dominated by Bi, HfSeTe, and ZrSe2 monolayers, respectively. The inset diagram shows nine photogenerated carrier dynamics pathways: the electron transfer (➀➁), the hole transfer (➂➃), the interlayer electron–hole recombination (➄➅), and the intralayer electron–hole recombination (➆➇➈). | |
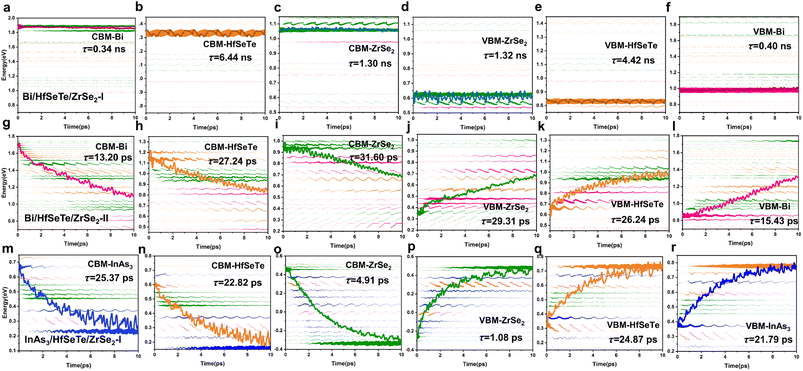 |
| Fig. 6 The time-dependent evolution of the electron/hole spatial localization and distribution in each state in Bi/HfSeTe/ZrSe2-I, Bi/HfSeTe/ZrSe2-II, and InAs3/HfSeTe/ZrSe2-I. The purplish red, blue, orange, and green lines represent the energy states dominated by Bi, InAs3, HfSeTe, and ZrSe2 monolayers, respectively. The descent or ascent lines represent the evolution of the averaged energy of the carriers in the considered states. (a), (g) and (m) CBM@Bi/InAs3, (b), (h) and (n) CBM@HfSeTe, (c), (i) and (o) CBM@ZrSe2, (d), (j) and (p) VBM@ZrSe2, (e), (k) and (q) VBM@HfSeTe, and (f), (l) and (r) VBM@Bi/InAs3. | |
As presented in Fig. 5f–h and listed in Tables S7–S9,† the strong NAC originating from the narrow bandgaps between the adjacent states accelerated the carrier transfer into the involved states. A few peaks existed in the spectral density of the six states of Bi/HfSeTe/ZrSe2-I due to the gentle fluctuations of Kohn–Sham orbitals, demonstrating that the slight vibration of atoms contributed minimally to the NAC, as shown in Fig. 5f. Therefore, all energy relaxation processes in Bi/HfSeTe/ZrSe2-I were slow, and the time scale difference in the six energy relaxation processes was nearly 10 times, which was averse to the transfer of carriers in the heterostructure and promoted the photocatalytic process, as shown in Fig. 6a–f. The numerous strong peaks, as well as the small bandgaps between CBM@Bi and the adjacent state in the Bi/HfSeTe/ZrSe2-II, further led to the strongest NAC (47.17 meV) in the six energy states, as shown in Fig. 5g. Hence, the electron relaxation in the highest CBM was the quickest one among the six processes of Bi/HfSeTe/ZrSe2-II, as shown in Fig. 6g–l. Similarly, the NAC between VBM@ZrSe2 and the adjacent states was the strongest one (48.63 meV), as shown in Fig. 5h, so entire hole relaxation on this energy level was the quickest one among the six processes of InAs3/HfSeTe/ZrSe2-I, as shown in Fig. 6m–r. Moreover, all energy relaxation processes in Bi/HfSeTe/ZrSe2-II were in the same time scale, which benefited the carrier cycling and were utilized in the photocatalysis.
The carrier population evolution relative to time in Fig. 7 demonstrated a characteristic of exponential function among the six transfer processes of the interlayer carriers. As in a previous analysis method,69,79 we fit the time population evolutions with the function f(t) = exp(−t/τ) to understand the evolution tendency. The τ corresponding to the carrier population of 1/e was defined as the carrier lifetime because the transfer process corresponding to this carrier population of an electronic state was considered irreversible and over. Generally, the slow carrier transfer in CBM@Bi/CBM@InAs3 and VBM@ZrSe2 was beneficial for the carriers to participate in the redox reaction of the Z-schemes. As shown in Fig. 7, the longest carrier lifetimes of 14.64 and 126.77 ps were attributed to Bi/HfSeTe/ZrSe2-I among the three heterostructures, indicating that the redox abilities of the two energy levels can be effectively protected. The Z-schemes with Bi/HfSeTe/ZrSe2-II and InAs3/HfSeTe/ZrSe2-I were more efficient than that with Bi/HfSeTe/ZrSe2-I owing to the quick carrier transfer migrations during the interlayer e–h recombination processes in CBM@HfSeTe, VBM@HfSeTe, CBM@ZrSe2, and VBM@Bi/InAs3 in the former two heterostructures. When the carrier migrations and recombination were involved, InAs3/HfSeTe/ZrSe2-I was superior to the others.
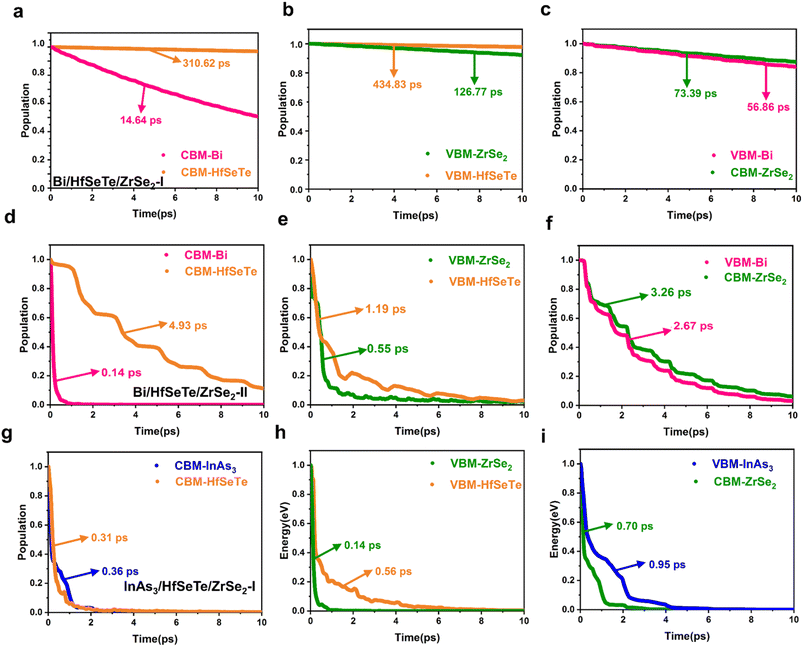 |
| Fig. 7 The carrier transfer dynamics in Bi/HfSeTe/ZrSe2-I, Bi/HfSeTe/ZrSe2-II, and InAs3/HfSeTe/ZrSe2-I. (a), (d) and (g) CBM@Bi/InAs3 and CBM@HfSeTe, (b), (e) and (h) VBM@ZrSe2 and VBM@HfSeTe, and (c), (f) and (i) CBM@ZrSe2 and VBM@Bi/InAs3. | |
3.6 Gibbs free energies of the HER and OER
According to the Sabatier principle,80 the closer the Gibbs free energy ΔG is to zero, the easier the reaction proceeds. As shown in Table S10,† the absorbed energy of the H atom on every unequal position demonstrated that the H atom preferred to adsorb onto the Bi1 atom in Bi/HfSeTe/ZrSe2-I and Bi/HfSeTe/ZrSe2-II and on the In atom in InAs3/HfSeTe/ZrSe2-I. The adsorption sites with the lowest energy were selected to calculate the ΔGs for the HER and OER. The results are presented in Fig. S11.† The potential of the photogenerated electrons (Ue) for the HER was measured from the CBM to the H+/H2 potential, whereas that of the photogenerated holes (Uh) for the OER was determined by the difference between the VBM and the potential of H+/H2.81,82 When the ΔGH*'s were less than eUes, the HER proceeded spontaneously. Similarly, the OER spontaneously proceeded when all ΔG's of the intermediates in the OER (ΔGOER's) were less than the eUh's. Considering that pH can impact the redox potentials of overall water splitting,83 we calculated the ΔG's for the HER and OER with pH 0–14 according to the method described in the ESI.†
As shown in Fig. 8, the eUe's with Bi/HfSeTe/ZrSe2-I, Bi/HfSeTe/ZrSe2-II, and InAs3/HfSeTe/ZrSe2-I were 0.81, 0.88, and 0.32 eV, respectively. The HERs at pH 0 on Bi/HfSeTe/ZrSe2-I and Bi/HfSeTe/ZrSe2-II can spontaneously proceed because the ΔG*H's of 0.68 and 0.41 eV were smaller than the corresponding eUe's. However, the ΔG*H of 0.34 eV with InAs3/HfSeTe/ZrSe2-I was 0.02 eV larger than the eUe, indicating that the HER cannot spontaneously proceed. pH can affect the redox potentials for the HER and OER. As shown in Fig. S12,† the HERs on Bi/HfSeTe/ZrSe2-I and Bi/HfSeTe/ZrSe2-II spontaneously proceeded within the pH ranges of 0.0–2.37 and 0.0–7.97, respectively, although the HER on InAs3/HfSeTe/ZrSe2-I failed in all pH values. However, the ΔG*H's with Bi/HfSeTe/ZrSe2-I were smaller than the reported values that have been experimentally determined on some materials (e.g., 1.5 eV for Mo2CO2
84) for HERs, indicating that all of them were feasible if additional energy was provided. During the calculations of the Gibbs free energies of (ΔGmax's), the reactions with the *OH intermediates were the rate-limiting steps for all considered OERs. The OERs with the three heterostructures had 2.13, 2.14, and 2.13 eV of ΔGmax's, and spontaneously proceed within the pH ranges of 6.44–14, 6.78–14, and 3.22–14, as shown in Fig. S12.† If the HER and OER were involved, only Bi/HfSeTe/ZrSe2-II can drive them to proceed spontaneously within pH 6.78–7.97.
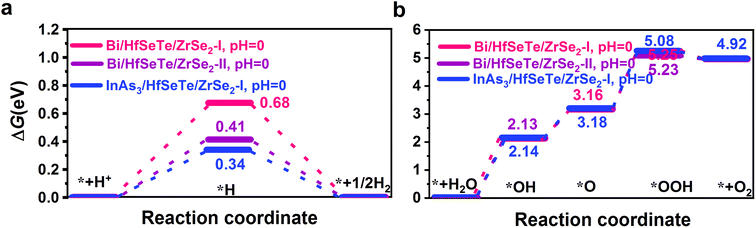 |
| Fig. 8 The Gibbs free energy changes for (a) HERs and (b) OERs at pH = 0 of Bi/HfSeTe/ZrSe2-I, Bi/HfSeTe/ZrSe2-II, and InAs3/HfSeTe/ZrSe2-I, respectively. | |
4. Conclusions
We constructed cascade dual Z-schemes with very high STH efficiency by using the trilayer Bi/HfSeTe/ZrSe2-I, Bi/HfSeTe/ZrSe2-II, and InAs3/HfSeTe/ZrSe2-I heterostructures after examining eight trilayer heterostructures. We found that the bilayer or trilayer heterostructures comprising HfSe2 and ZrSe2 monolayers were type-I or dual type-I band alignments and cannot drive the HERs. Meanwhile, the HfSeTe/ZrSe2-I heterostructure met the overall water splitting requirements but had low overpotentials for the HER. For the four sandwich configurations formed with the HfSeTe and ZrSe2 monolayers, the CBMs and VBMs cannot simultaneously match the potential conditions of the HER and OER. The cascade dual Z-schemes with η′STH's of 40.52%, 39.47%, and 41.04% were constructed for Bi/HfSeTe/ZrSe2-I, Bi/HfSeTe/ZrSe2-II, and InAs3/HfSeTe/ZrSe2-I after examining eight configurations obtained by adding the Bi or InAs3 monolayer into the bilayer HfSeTe/ZrSe2 heterostructures. Results of NAMD simulations demonstrated that the redox abilities of the three cascade dual Z-scheme heterostructures, especially for Bi/HfSeTe/ZrSe2-I, were protected because of the slow carrier migrations in CBM@Bi(InAs3) and VBM@ZrSe2. The ΔG*H's and ΔGOER's demonstrated the thermodynamic feasibility of the HERs and OERs driven by the three trilayer heterostructures. Remarkably, Bi/HfSeTe/ZrSe2-II can drive the HER and OER to proceed spontaneously at pH 6.78–7.97. Therefore, Bi/HfSeTe/ZrSe2-II was a candidate for developing an efficient photocatalyst for hydrogen production from overall water splitting. The cascade dual Z-scheme was a promising mechanism to achieve high STH efficiency and worth extending to develop more photocatalysts.
Author contributions
X. Q. Wan: data curation, investigation, writing-original draft preparation. C. L. Yang: conceptualization, methodology, supervision, writing – reviewing and editing. X. H. Li: methodology. Y. L. Liu: visualization. W. K. Zhao: software.
Conflicts of interest
The authors declare no competing financial interest.
Acknowledgements
This work is supported by the National Natural Science Foundation of China (Grant No. 12374232). C. L. Yang acknowledges the Xinjiang Tianchi Talent Program (2023).
References
- T. Hisatomi and K. Domen, Reaction systems for solar hydrogen production via water splitting with particulate semiconductor photocatalysts, Nat. Catal., 2019, 2, 387–399 CrossRef CAS.
- J. Liu, Y. Liu, N. Liu, Y. Han, X. Zhang, H. Huang and Z. Kang, Metal-free efficient photocatalyst for stable visible water splitting via a two-electron pathway, Science, 2015, 347, 970–974 CrossRef CAS PubMed.
- J. Kou, C. Lu, J. Wang, Y. Chen, Z. Xu and R. S. Varma, Selectivity enhancement in heterogeneous photocatalytic transformations, Chem. Rev., 2017, 117, 1445–1514 CrossRef CAS.
- X. X. Li, J. Zhao and J. L. Yang, Semi-hydrogenated BN sheet: a promising visible-light-driven photocatalyst for water splitting, Sci. Rep., 2013, 3, 1858 CrossRef.
- Y. Ji, M. Yang, H. Dong, T. Hou, L. Wang and Y. Li, Two-dimensional germanium monochalcogenide photocatalyst for water splitting under ultraviolet, visible to near-infrared light, Nanoscale, 2017, 9, 8608–8615 RSC.
- V. Kiran, D. Mukherjee, R. N. Jenjeti and S. Sampath, Active guests in the MoS2/MoSe2 host lattice: efficient hydrogen evolution using few-layer alloys of MoS2(1−x)Se2x, Nanoscale, 2014, 6, 12856–12863 RSC.
- Y. Fan, X. Ma, J. Wang, X. Song, A. Wang, H. Liu and M. Zhao, Highly-efficient overall water splitting in 2D Janus group-III chalcogenide multilayers: the roles of intrinsic electric filed and vacancy defects, Chin. Sci. Bull., 2020, 65, 27–34 CrossRef CAS.
- X. Liu, P. Cheng, X. Zhang, T. Shen and J. Liu,
et al., Enhanced solar-to-hydrogen efficiency for photocatalytic water splitting based on a polarized heterostructure: the role of intrinsic dipoles in heterostructures, J. Mater. Chem. A, 2021, 9, 14515–14523 RSC.
- T. Zhao, J. Chen, X. Wang and M. Yao,
et al., Ab initio insights into electronic structures, optical and photocatalytic properties of Janus WXY (X/Y = O, S, Se, and Te), Appl. Surf. Sci., 2021, 545, 148968 CrossRef CAS.
- Y. Fan, X. Song, S. Qi, X. Ma and M. Zhao, Li-III–VI bilayers for efficient photocatalytic overall water splitting: the role of the intrinsic electric field, J. Mater. Chem. A, 2019, 7, 26123–26130 RSC.
- Y. Fan, S. Qi, W. Li and M. Zhao, Direct Z-scheme photocatalytic CO2 conversion to solar fuels in a two-dimensional C2N/aza-CMP heterostructure, Appl. Surf. Sci., 2021, 541, 148630 CrossRef CAS.
- V. O. Özçelik, J. G. Azadani, C. Yang, S. J. Koester and T. Low, Band alignment of two-dimensional semiconductors for designing heterostructures with momentum space matching, Phys. Rev. B, 2016, 94, 035125 CrossRef.
- C. Lei, Y. Ma, X. Xu, T. Zhang and B. Huang,
et al., Broken-gap type-III and alignment in WTe2/HfS2 van der Waals heterostructure, J. Phys. Chem. C, 2019, 123, 23089–23095 CrossRef CAS.
- Q. K. Yin, C. L. Yang, M. S. Wang and X. G. Ma, Two-dimensional heterostructures of AuSe/SnS for the photocatalytic hydrogen evolution reaction with a Z-scheme, J. Mater. Chem. C, 2021, 9, 12231–12238 RSC.
- R. Sun, C. L. Yang, M. S. Wang and X. G. Ma, High solar-to-hydrogen efficiency photocatalytic hydrogen evolution reaction with the HfSe2/InSe heterostructure, J. Power Sources, 2022, 547, 232008 CrossRef CAS.
- Q. K. Yin, C. L. Yang, M. S. Wang and X. G. Ma, Two-dimensional AuSe/SnSe heterostructure for solar photocatalytic hydrogen evolution reaction with Z-scheme, Sol. Energy Mater. Sol. Cells, 2022, 247, 111940 CrossRef CAS.
- X. Wang, X. Wang, J. Huang, S. Li, A. Meng and Z. Li, Interfacial chemical bond and
internal electric field modulated Z-scheme Sv-ZnIn2S4/MoSe2 photocatalyst for efficient hydrogen evolution, Nat. Commun., 2021, 12, 4112 CrossRef CAS.
- Y. Chao, P. Zhou, N. Li, J. Lai, Y. Yang and Y. Zhang,
et al., Ultrathin visible-light-driven Mo Incorporating In2O3–ZnIn2Se4 Z-Scheme Nanosheet Photocatalysts, Adv. Mater., 2019, 31, 1807226 CrossRef PubMed.
- G. Zuo, Y. Wang, W. L. Teo, Q. Xian and Y. Zhao, Direct Z-scheme TiO2–ZnIn2S4 nanoflowers for cocatalyst-free photocatalytic water splitting, Appl. Catal., B, 2021, 291, 120126 CrossRef CAS.
- C. Zeng, Y. Hu, T. Zhang, F. Dong and Y. Zhang,
et al., Core-Satellite structured Z-scheme catalyst Cd0.5Zn0.5S/BiVO4 for highly efficient and stable photocatalytic water splitting, J. Mater. Chem. A, 2018, 6, 16932–16942 RSC.
- F. Wang, C. L. Yang, M. S. Wang and X. G. Ma, PtTe2/Sb2S3 nanoscale heterostructures for the photocatalytic direct Z-scheme with high solar-to-hydrogen efficiency: a theoretical investigation, ACS Appl. Nano Mater., 2023, 6, 5591–5601 CrossRef CAS.
- J. Meng, J. Wang, J. Wang and Q. Li,
et al., C7N6/Sc2CCl2 Weak van der Waals heterostructure: A promising visible-lLight-driven Z-scheme water splitting photocatalyst with interface ultrafast carrier recombination, J. Phys. Chem. Lett., 2022, 13, 1473–1479 CrossRef CAS.
- J. Wang, X. Zhang, X. Song and Y. Fan,
et al., Insights into photoinduced carrier dynamics and overall water splitting of Z-scheme van der Waals heterostructures with intrinsic electric polarization, J. Phys. Chem. Lett., 2023, 14, 798–808 CrossRef CAS.
- S. Ali, M. Humayun, W. Pi, Y. Yuan and M. Wang, Fabrication of BiFeO3-g-C3N4-WO3 Z-scheme heterojunction as highly efficient visible-light photocatalyst for water reduction and 2,4-chlorophenol degradation: insight mechanism, J. Hazard Mater., 2021, 397, 22708 Search PubMed.
- X. Wang, G. Hai, B. Li, Q. Luan and W. Dong,
et, al., Construction of dual-Z-scheme WS2–WO3·H2O/g-C3N4 catalyst for photocatalytic H2 evolution under visible light, Chem. Eng. J., 2021, 426, 130822 CrossRef CAS.
- X. Li, H. Jiang, C. Ma, Z. Zhu, X. Song and H. Wang,
et al., Local surface plasma resonance effect enhanced Z-scheme ZnO/Au/g-C3N4 film photocatalyst for reduction of CO2 to CO, Appl. Catal., B, 2021, 283, 119638 CrossRef CAS.
- J. Jia, Q. Zhang, K. Li, Y. Zhang, E. Liu and X. Li, Recent advances on g–C3N4–based Z-scheme photocatalysts: Structural design and photocatalytic applications, Int. J. Hydrogen Energy, 2023, 48, 196e231 Search PubMed.
- H. Yu, D. Wang, B. Zhao, Y. Lu, X. Wang and S. Zhu,
et al., Enhanced photocatalytic degradation of tetracycline under visible light by using a ternary photocatalyst of Ag3PO4/AgBr/g-C3N4 with dual Z-scheme heterojunction, Sep. Purif. Technol., 2021, 237, 16365 Search PubMed.
- M. Wang, G. Tan, H. Ren, A. Xia and Y. Liu, Direct double Z-scheme g-C3N4/Zn2SnO4N/ZnO ternary heterojunction photocatalyst with enhanced visible photocatalytic activity, Appl. Surf. Sci., 2019, 492, 690–702 CrossRef CAS.
- S. Sun, H. Cheng, K. Cao, A. Song, C. Xu and J. Ba,
et al., Ultrafast charge separation in ternary V2O5/CdS/CoS2 Z-scheme heterojunction enables efficient visible-light-driven hydrogen generation, Energy Fuels, 2022, 36, 2034–2043 CrossRef CAS.
- R. Zhang and K. Zeng, A novel flower-like dual Z-scheme BiSi/Bi2WO6/g-C3N4 photocatalyst has excellent photocatalytic activity for the degradation of organic pollutants under visible light, Diamond Relat. Mater., 2021, 115, 108343 CrossRef CAS.
- W. Xue, D. Huang, J. Li, G. Zeng, R. Deng and Y. Yang,
et al., Assembly of AgI nanoparticles and ultrathin g-C3N4 nanosheets codecorated Bi2WO6 direct dual Z-scheme photocatalyst: an efficient, sustainable and heterogeneous catalyst with enhanced photocatalytic performance, Chem. Eng. J., 2019, 373, 1144–1157 CrossRef CAS.
- K. E. Aretouli, P. Tsipas and D. Tsoutsou,
et al., Two-dimensional semiconductor HfSe2 and MoSe2/HfSe2 van der Waals heterostructures by molecular beam epitaxy, Appl. Phys. Lett., 2015, 106, 143105 CrossRef.
- P. Tsipas, D. Tsoutsou and J. Marquez-Velasco,
et al., Epitaxial ZrSe2/MoSe2 semiconductor van der Waals heterostructures on wide band gap AlN substrates, Microelectron. Eng., 2015, 147, 269–272 CrossRef CAS.
- M. J. Mleczko, C. Zhang, H. R. Lee and H. H. Kuo,
et, al., HfSe2 and ZrSe2: two-dimensional semiconductors with native high-κ oxides, Sci. Adv., 2017, 3, e1700481 CrossRef PubMed.
- X. Q. Wan, C. L. Yang, M. S. Wang and X. G. Ma, Efficient photocatalytic hydrogen evolution and CO2 reduction by HfSe2/GaAs3 and ZrSe2/GaAs3 heterostructures with direct Z-schemes, Phys. Chem. Chem. Phys., 2023, 25, 8861–8870 RSC.
- R. Sivasamy, K. Paredes-Gil and J. V. Ramaclus, Sandwich-like GaN/MoSe2/GaN heterostructure nanosheet: a first-principle study of the structure, electronic, optical, and thermodynamical properties, Surf. Interfaces, 2022, 34, 102298 CrossRef CAS.
- D. S. Gavali, Y. Kawazoe and R. Thapa, First-principles identification of interface effect on Li storage capacity of C3N/graphene multilayer heterostructure, J. Colloid Interface Sci., 2022, 610, 80–88 CrossRef CAS.
- X. Q. Wan, C. L. Yang, M. S. Wang and X. G. Ma, Boost solar-to-hydrogen efficiency by constructing heterostructures with the pristine and Se/Te-doped AgInP2S6 monolayers, Appl. Surf. Sci., 2023, 614, 156254 CrossRef CAS.
- Q. Zheng, W. Chu, C. Zhao and L. Zhang,
Ab initio nonadiabatic molecular dynamics investigations on the excited carriers in condensed matter systems, Wiley Interdiscip. Rev.: Comput. Mol. Sci., 2019, 9, e1411 CAS.
- G. Kresse and J. Furthmüller, Efficient iterative schemes for ab initio total-energy calculations using a plane-wave basis set, Comput. Mater., 1996, 6, 15–50 CrossRef CAS.
- P. E. Blöchl, Projector augmented-wave method, Phys. Rev. B, 1994, 50, 17953–17979 CrossRef.
- J. P. Perdew, K. Burke and M. Ernzerhof, Generalized gradient approximation made simple, Phys. Rev. Lett., 1996, 77, 3865 CrossRef CAS.
- S. Grimme, S. Ehrlich and L. Goerigk, Effect of the damping function in dispersion corrected density functional theory, J. Comput. Chem., 2011, 32, 1456–1465 CrossRef CAS.
- S. Grimme, S. Ehrlich and L. Goerigk, A consistent and accurate ab initio parametrization of density functional dispersion correction (DFT-D) for the 94 elements H-Pu, J. Chem. Phys., 2010, 132, 154104 CrossRef.
- S. Nose, An extension of the canonical ensemble molecular dynamics method, J. Phys. Chem. Lett., 1984, 81, 511 CrossRef CAS.
- W. G. Hoover, Canonical dynamics: equilibrium phase-space distributions, Phys. Rev. A, 1985, 31, 1695 CrossRef PubMed.
- J. Heyd, G. E. Scuseria and M. Ernzerhof, Hybrid functionals based on a screened Coulomb potential, J. Chem. Phys., 2003, 118, 8207 CrossRef CAS.
- H. M. Jaeger, S. Fischer and O. V. Prezhdo, Decoherence-induced surface hopping, J. Chem. Phys., 2012, 137, 22a545 CrossRef PubMed.
- Y. Tian, M. Zheng and Y. Cheng,
et al., Epitaxial growth of ZrSe2 nanosheets on sapphire via chemical vapor deposition for optoelectronic application, J. Mater. Chem. C, 2021, 9(39), 13954–13962 RSC.
- K. Li, B. Peng and T. Peng, Recent advances in heterogeneous photocatalytic CO2 conversion to solar fuels, ACS Catal., 2016, 6, 7485–7527 CrossRef CAS.
- R. F. W. Bader, Atoms in molecules: a quantum theory, Adv. Quantum Chem., 1981, 14, 63–124 CrossRef CAS.
- Y. Ji, M. Yang, H. Lin, T. Hou, L. Wang and Y. Li,
et al., Janus structures of transition metal dichalcogenides as the heterojunction photocatalysts for water splitting, J. Phys. Chem. C, 2018, 122, 3123–3129 CrossRef CAS.
- N. Jena, A. Rawat, R. Ahammed and M. K. Mohanta,
et al., Emergence of high piezoelectricity along with robust electron mobility in Janus structures in semiconducting Group IVB dichalcogenide monolayers, J. Mater. Chem. A, 2018, 6, 24885–24898 RSC.
- L. Wan, D. Chen, W. Zeng, J. Li and S. Xiao, Hazardous gas adsorption of Janus HfSeTe monolayer adjusted by surface vacancy defect: A DFT study, Surf. Interfaces, 2022, 34, 102316 CrossRef CAS.
- A. Y. Lu, H. Zhu and J. Xiao,
et al., Janus monolayers of transition metal dichalcogenides, Nat. Nanotechnol., 2017, 12(8), 744–749 CrossRef CAS PubMed.
- P. Mudgal, H. Arora and J. Pati,
et al., Electrochemical investigation of MoSeTe as an anode for sodium-ion batteries, Proc. Indian Natl. Sci. Acad., 2022, 88(3), 430–438 Search PubMed.
- K. Zhang, Y. Guo and D. T. Larson,
et al., Spectroscopic signatures of interlayer coupling in Janus MoSSe/MoS2 heterostructures, ACS Nano, 2021, 15(9), 14394–14403 CrossRef CAS PubMed.
- K. Si, J. Ma and C. Lu,
et al., A two-dimensional MoS2/WSe2 van der Waals heterostructure for enhanced photoelectric performance, Appl. Surf. Sci., 2020, 507, 145082 CrossRef CAS.
- P. Tsipas, D. Tsoutsou and J. Marquez-Velasco,
et al., Epitaxial ZrSe2/MoSe2 semiconductor vd Waals heterostructures on wide band gap AlN substrates, Microelectron. Eng., 2015, 147, 269–272 CrossRef CAS.
- S. Singh, Z. Zanolli, M. Amsler and B. Belhadji,
et al., Low-energy phases of Bi monolayer predicted by structure searching two dimensions, J. Phys. Chem. Lett., 2019, 10, 7324–7332 CrossRef CAS PubMed.
- K. Mathew, R. Sundararaman and K. Letchworth-Weaver,
et al., Implicit solvation model for density-functional study of nanocrystal surfaces and reaction pathways, J. Chem. Phys., 2014, 140(8), 084106 CrossRef.
- C. F. Fu, J. Sun and Q. Luo,
et al., Intrinsic electric fields in two-dimensional materials boost the solar-to-hydrogen efficiency for photocatalytic water splitting, Nano Lett., 2018, 18(10), 6312–6317 CrossRef CAS PubMed.
- G. Wang, J. Chang and S. D. Guo,
et al., MoSSe/Hf (Zr) S2 heterostructures used for efficient Z-scheme photocatalytic water-splitting, Phys. Chem. Chem. Phys., 2022, 24(41), 25287–25297 RSC.
- B. Lu, X. Zheng and Z. Li, Two-dimensional lateral heterostructures of triphosphides: AlP3–GaP3 as a promising photocatalyst for water splitting, ACS Appl. Mater. Interfaces, 2020, 12(48), 53731–53738 CrossRef CAS.
- X. Q. Wan, C. L. Yang and X. H. Li,
et al., Insights into photogenerated carrier dynamics and overall water splitting of the CrS3/GeSe heterostructure, J. Phys. Chem. Lett., 2023, 14(40), 9126–9135 CrossRef CAS PubMed.
- J. C. Lai, L. Li and D. P. Wang,
et al., A rigid and healable polymer cross-linked by weak but abundant Zn (II)-carboxylate interactions, Nat. Commun., 2018, 9(1), 2725 CrossRef PubMed.
- P. Zhou, I. A. Navid and Y. Ma,
et al., Solar-to-hydrogen efficiency of more than 9% in photocatalytic water splitting, Nature, 2023, 613(7942), 66–70 CrossRef CAS PubMed.
- R. Long and O. V. Prezhdo, Quantum coherence facilitates efficient charge separation at a MoS2/MoSe2 van der Waals junction, Nano Lett., 2016, 16, 1996–2003 CrossRef CAS PubMed.
- F. Ceballos, M. Z. Bellus, H. Y. Chiu and H. Zhao, Ultrafast charge separation and indirect exciton formation in a MoS2–MoSe2 van der Waals heterostructure, ACS Nano, 2014, 8, 12717–12724 CrossRef CAS.
- W. Dou, Y. Jia, X. Hao, Q. Meng and J. Wu,
et al., Time-domain ab initio insights into the reduced nonradiative electron-hole recombination in ReSe2/MoS2 van der Waals heterostructure, J. Phys. Chem. Lett., 2021, 12, 2682–2690 CrossRef CAS PubMed.
- J. He, L. Zhang, D. He, Y. Wang, Z. He and H. Zhao, Ultrafast transient absorption measurements of photocarrier dynamics in monolayer and bulk ReSe2, Opt. Express, 2018, 26, 21501–21509 CrossRef CAS PubMed.
- X. Hong, J. Kim, S. F. Shi, Y. Zhang, C. Jin and Y. Sun,
et al., Ultrafast charge transfer in atomically thin MoS2/WS2 heterostructures, Nat. Nanotechnol., 2014, 9, 682–686 CrossRef CAS.
- Q. Zheng, W. A. Saidi, Y. Xie, Z. Lan and O. V. Prezhdo,
et al., Phonon-assisted ultrafast charge transfer at van der Waals heterostructure interface, Nano Lett., 2017, 17, 6435–6442 CrossRef CAS.
- Z. Zhang, W. H. Fang and R. Long,
et, al., Exciton dissociation and suppressed charge recombination at 2D perovskite edges: key roles of unsaturated halide bonds and thermal disorder, J. Am. Chem. Soc., 2019, 141, 15557–15566 CrossRef CAS.
- Y. Wei, W. H. Fang and R. Long, Covalent functionalized black phosphorus greatly inhibits nonradiative charge recombination: a time domain ab initio study, J. Phys. Chem. Lett., 2020, 11, 478–484 CrossRef CAS.
- J. He, D. Casanova, W. H. Fang and R. Long,
et al., MAI termination favors efficient hole extraction and slow charge recombination at the MAPbI3/CuSCN heterojunction, J. Phys. Chem. Lett., 2020, 11, 4481–4489 CrossRef CAS.
- S. V. Kilina, A. J. Neukirch, B. F. Habenicht and D. S. Kilin,
et al., Quantum zeno effect rationalizes the phonon bottleneck in semiconductor quantum dots, Phys. Rev. Lett., 2013, 110, 180404 CrossRef.
- Y. Wang and R. Long, Rapid decoherence induced by light expansion suppresses charge recombination in mixed cation perovskites: time-domain ab initio analysis, J. Phys. Chem. Lett., 2020, 11, 1601–1608 CrossRef CAS.
- P. Sabatier,
et, al., Hydrogénations et déshydrogénations par catalyse, Chem. Ges., 1911, 44, 1984–2001 CrossRef CAS.
- J. Rossmeisl, Z. W. Qu, H. Zhu and G. J. Kroes,
et al., Electrolysis of water on oxide surfaces, J. Electroanal. Chem., 2007, 607, 83–89 CrossRef CAS.
- J. K. Nørskov, J. Rossmeisl and A. Logadottir,
et al., Origin of the overpotential for oxygen reduction at a fuel-cell cathode, J. Phys. Chem. B, 2004, 108, 17886–17892 CrossRef.
- Y. Fan, X. Song, X. Ma, W. Li and M. Zhao, Rational design of black phosphorus-based direct Z-scheme photocatalysts for overall water splitting: The role of defects, J. Phys. Chem. Lett., 2022, 13, 9363–9371 CrossRef CAS.
- D. A. Kuznetsov, Z. Chen and P. V. Kumar,
et al., Single site cobalt substitution in 2D molybdenum carbide (MXene) enhances catalytic activity in the hydrogen evolution reaction, J. Am. Chem. Soc., 2019, 141, 17809–17816 CrossRef CAS PubMed.
|
This journal is © The Royal Society of Chemistry 2024 |
Click here to see how this site uses Cookies. View our privacy policy here.