DOI:
10.1039/D4TA03343D
(Paper)
J. Mater. Chem. A, 2024,
12, 19268-19276
Drag reduction and antifouling of a spontaneous fast moving air film†
Received
14th May 2024
, Accepted 20th June 2024
First published on 21st June 2024
Abstract
Minimizing frictional resistance and preventing the attachment of marine organisms are extremely important for global ship navigation to reduce energy consumption and carbon emissions. However, there is still a lack of an effective and environmentally-friendly drag reduction and antifouling method. Here, we propose a spontaneous fast-moving air film to resolve this problem. We first developed a serial brachistochrone-shaped superhydrophobic pattern to easily capture underwater air bubbles and realize the spontaneous and directional transportation of bubbles with an average velocity of 444 mm s−1 that is the fastest in the whole world. Then, the continuous supply of air bubbles and serial brachistochrone-shaped superhydrophobic pattern were combined to form a spontaneous fast-moving air film, which not only prevents the escape of supplied air bubbles but also replenishes the air film on a superhydrophobic surface. The spontaneous fast-moving air film showed an amazing 27% drag reduction rate and simultaneous 80% antifouling rate, which was an outstanding capability that had never been reported before. This effective and environmentally-friendly drag reduction and antifouling method will minimize energy consumption and carbon emissions and has strong implications for global sustainable development.
1 Introduction
To protect the future of mankind, 178 countries signed the Paris Agreement with reduce the emission of carbon dioxide.1,2 Ship navigation is a major energy consumer and carbon emitter, which consumed about 320 million tons of fuel and emitted more than 1 billion tons of CO2 in 2022.3 Reducing the drag of a ship is considered to be the most effective method to reduce its energy consumption and carbon emission.4 The frictional resistance originating from the friction between the surface of a ship's hull and surrounding water is the major component of the ship's water resistance.5,6 In addition, attached marine organisms originating from biofouling can further increase the frictional resistance of the ship.7–9 Therefore, minimizing frictional resistance and preventing the attachment of marine organisms are of great significance.10
Constructing microstructures inspired by shark skin is a popular method to reduce the frictional resistance of a subaqueous object.11 However, due to their lack of antifouling capability, marine organisms can easily attach to sharkskin-like microstructures, which in turn increases the frictional resistance.12,13 Injecting air bubbles onto the ship's bottom is a more practical method of drag-reduction and has been applied in many large ships, such as ships carrying ore or liquefied natural gas. However, air bubbles are difficult to anchor to the ship's bottom, and there is a heavy escape rate, resulting in a low drag reduction rate.14–16 In recent years, a superhydrophobic surface has been proposed to reduce frictional resistance because of its self-air film which is stored in surface microstructures.17–20 However, this air film is easily broken and flows away, leaving a disappeared drag reduction phenomenon.21–26 For marine antifouling, many chemical coatings are applied to kill the fouling organisms.27,28 However, those coatings have poor antifouling capability and show great toxicity to marine organisms, endangering the marine environment.29 Therefore, a more effective and environmentally-friendly drag reduction and antifouling method still needs to be explored.
In this work, we first optimally designed a serial cycloid-shaped pattern (SCP) which could spontaneously capture underwater air bubbles to realize the spontaneous and directional transportation of bubbles with an average velocity of 444 mm s−1. Theoretical analysis was conducted to analyze the reason for the fast transportation of air bubbles. We then combined the continuous supply of air bubbles and SCP to form a spontaneous fast-moving air film, which not only prevents the escape of supplied air bubbles but also replenishes the air film on a superhydrophobic surface, showing an amazing 27% drag reduction rate and simultaneous 80% antifouling rate. This effective and environmentally-friendly drag reduction and antifouling method will save a large amount of energy and promote the sustainable development of the whole world.
2 Experimental section
2.1 Materials
1060 aluminum sheets (Al, surface-free energy of 980 mJ m−2) were purchased from Shenzhen Hongwang Co., Ltd (China). Anhydrous ethanol (surface-free energy of 22 mJ m−2) was bought from Tianjin Kemio Chemical Reagent Co., Ltd (China). Fluoroalkylsilane [FAS, C8F13H4Si(OCH2CH3)3, containing a –CF3 group with 6 mJ m−2 surface-free energy and a –CF2 group with 18 mJ m−2 surface-free energy] was provided by Degussa Co. Ltd (Germany). Marine plants and clownfish were purchased from the local marine pet market. Seawater (surface-free energy of 72 mJ m−2) was obtained from the Bohai Sea near Dalian.
2.2 Fabrication of samples
Preparation of serial brachistochrone-shaped pattern (SBP).
A diagram of the preparation of the SBP is shown in Figure S1.† The Al sheet after cleaning was etched via a laser (SK-CX30, Shanghai Sanke Laser Technology Co. Ltd., China) to construct micro-rough structures. Next, the etched Al sheet was immersed in a 5 wt% FAS ethanol solution for 1.5 h. After drying, a superhydrophobic Al surface was obtained. Then, the exterior surrounding region of the superhydrophobic Al surface was processed again with the above laser to remove the FAS (Figure S2†). Finally, an SBP composed of an exterior surrounding a superhydrophilic region and interior superhydrophobic region was obtained.
Preparation of rectangular pattern (RP).
The fabrication processes were similar to the above, but the pattern was rectangular.
Preparation of entire superhydrophobic surface.
The Al sheet was etched by the aforementioned laser first, and then, a superhydrophobic Al surface was obtained by FAS modification.
2.3 Sample characterization
The microstructure of the surface was observed by a scanning electron microscope (SEM, JSM-6360LV, Japan). The corresponding elemental compositions were characterized by an energy dispersive spectroscope (EDS, JSM-6360LV, Japan). The water contact angle (WCA) in air and air contact angle (ACA) underwater were measured by a contact angle meter (DSA100, Krüss, Germany) using a water droplet of 4.1 μL and an air bubble of 3 μL at ambient temperature.
2.4 Underwater air bubble transportation
The equipment for an underwater air bubble transportation experiment was self-made to evaluate the transportation capability of the underwater air bubble on different samples (Figure S3†). The water depth of the underwater bubble transportation experiment was 30 mm. To ensure that the air bubbles were captured by the pattern, the bubble volume and distance between the bubble outlet and sample surface were 40 μL and 2 mm, respectively. All air bubble transportation experiments were pre-covered in an 80 μL air film. The release of air bubbles was controlled by a precision dispensing pump (LSP02-2A, Longer Pump). The underwater air bubble transportation behavior was recorded by a high-speed camera (1000 frames, DSC-RX10M3, Sony, Japan).
2.5 Underwater drag reduction
The underwater drag reduction experimental system was self-made to evaluate the drag reduction capability of the different samples. The sample platform could fix the different experiment samples, and one side of the sample platform was connected to a force sensor (DYX-306, Bengbu Sensing System Engineering Co. Ltd, China). The underwater free air bubbles which were generated by a supplier of continuous air bubbles (ES-3910, Anyuan Huaqun Technology Co. Ltd, China) on one side of the sample platform were captured by the SBP to form a constrained air film and were quickly transported to the other side of the SBP. The underwater drag reduction rate was the ratio of the underwater resistance of the common Al surface without bubble supply with the underwater resistance of the test sample with bubble supply subtracted, to the underwater resistance of the common Al surface without bubble supply, where the water flow rate was 0.5 m s−1, and the air bubble flux was 0.4 L min−1.
2.6 Marine antifouling
The marine antifouling test included an anti-diatom adhesion test and an antifouling test in a simulated marine environment.
For the anti-diatom adhesion test, we placed the common Al surface, superhydrophobic surface, SBP without bubble supply, and SBP with bubble supply in water containing diatoms and provided light for diatom growth. In the anti-diatom adhesion test, we recorded the images of the different samples every 2 days and calculated the corresponding diatom coverage rate which was the ratio of the area covered by diatoms to the test surface area. After 8 days, all the samples were taken out and observed with an optical microscope (MV-EM200C, Microvision Intelligent Manufacturing Technology Co. Ltd., China) to evaluate whether the sample surfaces were fouled by the diatoms.
For the antifouling test in the simulated marine environment, we placed the common Al surface, SBP without bubble supply, and SBP with bubble supply into seawater from the Bohai Sea near Dalian. To better simulate the marine environment, we also added marine plants and some clownfish to the seawater. After 4 days, all the samples were taken out and observed by SEM and the elemental compositions of these samples were characterized by EDS to evaluate their antifouling capability in the marine environment.
3 Results and discussion
3.1 Spontaneous high-speed transportation of a single air bubble
We proposed that a spontaneous fast-moving air film could realize underwater drag reduction and antifouling. Thus, the first problem to be solved here is how to obtain the spontaneous high-speed transportation of a single air bubble. Actually, there were several methods to realize the spontaneous transportation of an air bubble, such as using 3D structures,30–33 slippery grooves,34–37 rectangular patterns,38–40 and wedge-shaped patterns.41–44 However, the transportation distance and velocity for the air bubble on those surfaces were not long or fast enough. Nepenthes is a superstar in the field of bionic engineering.45–47 Its lip shows a serial arch-shaped pattern which can spontaneously transport a liquid over a long distance with considerable velocity. Inspired by the Nepenthes lip and famous brachistochrone curve (Figures 1(a) and S4),48 a serial brachistochrone-shaped pattern (SBP) with several single brachistochrone-shaped patterns connected end to end was proposed, where r, θ1, and θ2 were the rotating circle radius, angle by which the rotating circle rotated from its origin position to the starting position of a single pattern, and the angle by which the rotating circle rotated from its origin position to the end position of a single pattern, respectively. The SBP was composed of an exterior surrounding superhydrophilic region with a water contact angle of 0° and an interior superhydrophobic region with a water contact angle of 164°. The superhydrophobicity resulted from the combined function of the micro-rough structures fabricated by the laser and FAS with a low surface energy.49–51 After laser etching again to remove the FAS, the superhydrophobicity was successfully transformed into superhydrophilicity. Underwater, the exterior surrounding superhydrophilic region showed superaerophobicity with an air contact angle of 162°, while the interior superhydrophobic region showed superaerophilicity with an air contact angle of 0° (Fig. 1(b) and (c)). The underwater free air bubbles could be easily captured by the SBP to form a constrained air bubble and simultaneously be spontaneously and directionally transported along the SBP (Fig. 1(d), S5 and Video S1†). To obtain the critical parameters for the fastest transportation velocity, we conducted the single-factor experiment first and later Box–Behnken design optimization. For the parameters r, θ1, θ2, and k, when three of them were fixed, all the average transportation velocities of the air bubble increased first and later decreased with an increase in the unfixed parameter (Figures 1(e)–(h), S6–S8,† and †. A fast transportation velocity of the air bubble of 400 mm s−1 was obtained at r of 22 mm, θ1 of 160°, θ2 of 180°, and k of 0.8 in the single-factor experiment. Based on the aforementioned results, the SBP was further optimized by Box-Behnken design optimization. As shown in Figure S9, Tables S1–S3, and Video S3,† a faster transportation velocity of 415 mm s−1 was obtained at r of 22.1 mm, θ1 of 160.4°, θ2 of 180°, and k of 0.8.
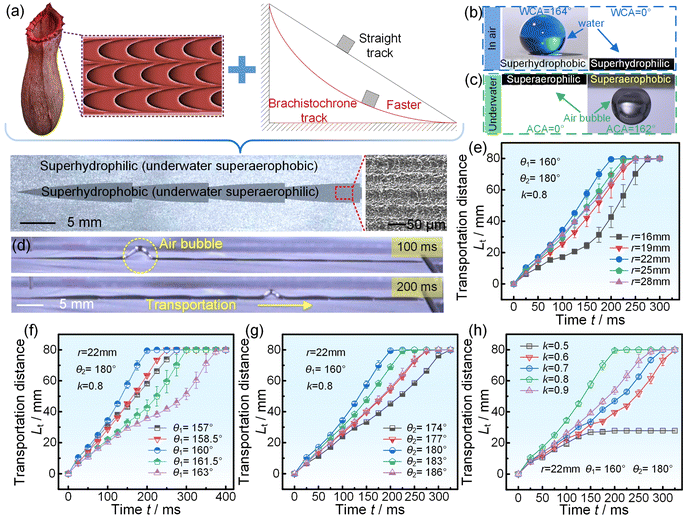 |
| Fig. 1 Proposal for the SBP, wettability of the SBP in air and underwater, and variation of the transportation distance with the SBP parameters. (a) This SBP which is inspired by the Nepenthes lip and famous brachistochrone curve was proposed. (b) The wettability of a 4.1 μL water droplet on the SBP in air. (c) The wettability of a 3 μL air bubble on the SBP underwater. (d) The underwater transportation behavior of an air bubble on the SBP. (e) The variation of the underwater air bubble transportation distance with r, where the θ1 of 160°, θ2 of 180°, and k of 0.8 are fixed. (f) The variation of the underwater air bubble transportation distance with θ1, where the r of 22 mm, θ2 of 180°, and k of 0.8 are fixed. (g) The variation of the underwater air bubble transportation distance with θ2, where the r of 22 mm, θ1 of 160°, and k of 0.8 are fixed. (h) The variation of the underwater air bubble transportation distance with k, where the r of 22 mm, θ1 of 160°, and θ2 of 180° are fixed. | |
We then studied the mechanism of influence of the SBP parameters (r, θ1, θ2, and k) on the transportation process of the air bubble. The force acting on the air bubble is shown in Fig. 2(a). Once the air bubble comes into contact with the SBP, it will be subjected to the Laplace force FL, capillary force FC, water resistance force FW, and pinning force FP, which can be expressed as follows:
where
F is the resultant force,
FL is the Laplace force,
FC is the capillary force,
FW is the resistance force from the water to the transported air bubble, and
FP is the resistance force from the junction to the transported air bubble. For
FC, the
FC of the air bubble on the SBP can be ignored. For
FL, it is derived from the unbalanced Laplace pressure between the air bubble of the front and rear sides, which is the main driving force and can be given by:
| FL ∼ γlf cos λf − γlb cos λb | (2) |
where
γ is the interfacial gas–water tension,
lf is the front contact line length of the air bubble,
λf is the front ACA of the air bubble,
lb is the rear-side contact line length of the air bubble,
λb is the rear-side ACA of the air bubble.
43 For the
FW, it can be described as:
| 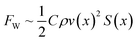 | (3) |
where
C,
ρ,
v(
x), and
S(
X) are the water drag coefficient, water density, instantaneous velocity of the air bubble at any transportation position
x, and the cross-sectional area of the air bubble at any transportation position
x.
FP can be demonstrated as follows:
| FP ∼ γ(ww − wn)(cos λa − cos λr) | (4) |
where
ww and
wn are the wide width and narrow width of the SBP, and
λa, and
λr are the advancing contact angle and receding contact angle of the air bubble at the junction. As shown in
Figure S6,
† decreasing
r, increasing
θ1, decreasing
θ2, and increasing
k led to an increase in the number of junctions, which meant that the air bubble was subjected to larger
FP, resulting in a decreasing average transportation velocity. Although increasing
r, decreasing
θ1, and increasing
θ2 could decrease the junction number, they would increase the SBP area, meaning that the air bubble would be more spread out on the SBP with a larger area than that on the SBP with a smaller area, showing the decreasing difference of the Laplace pressure between the front and rear sides of the air bubble, decreasing
FL, and decreasing average transportation velocity (
Figure S10†). In addition, according to
eqn (4), decreasing
k would increase
FP, resulting in decreasing average transportation velocity. If
k was further decreased, the air bubble could not even achieve long-distance transportation (
Figure S8†).
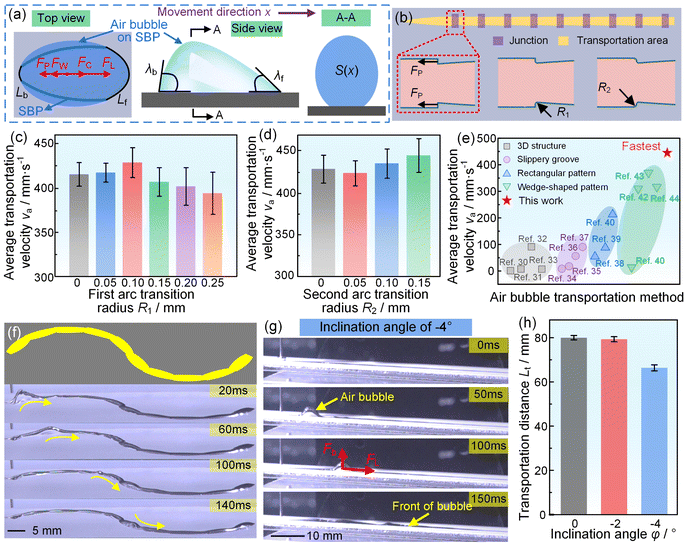 |
| Fig. 2 Force analysis of the air bubble on the SBP, junction optimization of the SBP, and SBP can transport the bubble in various complex conditions. (a) Force analysis of the air bubble on the SBP. (b) Schematic of junction optimization. (c) Average transportation velocity of the air bubble on the SBP with different first arc transition radii. (d) Average transportation velocity of the air bubble on the SBP with different second arc transition radii, where the first arc transition radius is 0.1 mm. (e) Average transportation velocities for different air bubble transportation methods. The details of the air bubble transportation velocity are included in Table S4.† (f) The air bubble transportation process on a curved SBP track. (g) The air bubble transportation process on the SBP with an inclination angle of −4°. (h) The transportation distance of a 40 μL air bubble on the SBP with inclination angles of 0°, −2°, and −4°. | |
From the above force analysis, it can be seen that the air bubble was subjected to FW and FP, where FW was difficult to decrease by optimal design. Therefore, we optimized the junction shape, using an arc to replace the wedge angle of the junction, which achieved decreasing FP and increasing average transportation velocity (Fig. 2(b)). We first optimized the first arc transition radius R1. The results showed that the average transportation velocity first increased and further decreased with increasing R1, as shown in Fig. 2(c). When R1 was 0.1 mm, the average transportation velocity increased from 415 mm s−1 to 428 mm s−1 (Video S4†). We then optimized the second arc transition radius R2, where R1 was 0.1 mm. The results showed that when R2 was 0.15 mm, the average transportation velocity was the fastest, reaching 444 mm s−1 (Fig. 2(d) and Video S4†). To confirm that our proposed SBP had faster transportation velocity than existing spontaneous air bubble transportation methods, we compared the SBP with these methods, as shown in Figures 2(e) and S11.30–44 It was surprising that the transportation velocity of the air bubble on the SBP was almost 1.4–296 times as high as those on 3D structures, slippery grooves, rectangular patterns, or wedge-shaped patterns, which meant that the transportation velocity of the air bubble on the SBP was the fastest among the existing spontaneous air bubble transportation methods. Specifically, the details of the above spontaneous air bubble transportation methods are shown in Table S4†. In addition, besides a straight SBP, the air bubble could realize fast transportation on a curved SBP, as shown in Fig. 2(f) and Video S5†. Moreover, buoyancy had a significant influence on underwater bubble transportation: when φ > 0°, the buoyancy would promote air bubble transportation; when φ < 0°, the buoyancy would hinder air bubble transportation (Figures S12 and S13†). It was amazing that the air bubble could even overcome the buoyancy and be spontaneously transported on the SBP with a transportation distance of 67 mm under a φ of −4°, (Fig. 2(g) and (h)). The aforementioned results showed that our proposed SBP is not only the fastest in the spontaneous bubble transportation field but can also transport the bubble in various complex conditions.
3.2 Underwater drag reduction
We then confirmed the importance of spontaneous high-speed transportation of air bubbles on improving the drag reduction rate. We designed an underwater drag reduction experiment system which included a supplier of continuous air bubbles and samples with different patterns (Fig. 3(a)). Every sample with 40 mm width and 200 mm length had 9 rectangular patterns (RPs) or 9 SBPs. For the common Al surface, we found that the supplied underwater free air bubbles were not captured by the surface and touched the surface only under the influence of buoyancy, but eventually escaped mostly without any spontaneous moving air film, which resulted in a low bubble capture rate of less than 5% (Fig. 3(b), (c) and Video S6†). For the sample with RPs, some underwater free air bubbles were captured by the RP to form constrained air bubbles and a spontaneous moving air film (Fig. 3(d)). Although the constrained air bubbles on the RP could harvest some surrounding air bubbles, it is hard for them to catch up and harvest the air bubbles in front of them, still leaving some escaping bubbles with a bubble capture rate of 74%. For the sample with SBPs, many underwater free air bubbles were captured by the SBP to form constrained air bubbles which were bigger and moved faster than those on the RP and not only harvested the surrounding air bubbles but also caught up and harvested the air bubbles in front of them with a bubble capture rate of 95%, leaving a spontaneous fast-moving air film (Fig. 3(e)). Therefore, compared with RP, the constrained air bubbles on the SBP moved faster and captured more air bubbles (Fig. 3(f)). In addition, a bigger bubble provided a greater contact area to capture the underwater free air bubbles (Fig. 3(g)). Eventually, a virtuous cycle of fast movement-capture more bubbles-get bigger-fast movement was created on the SBP to guarantee a spontaneous fast-moving air film. We next recorded the underwater resistance force curves of the common Al surface, common Al surface with bubble supply, 9 RPs with bubble supply, and 9 SBPs with bubble supply and calculated their drag reduction rates compared with the common Al surface using MATLAB software, as shown in Fig. 3(h), S14, and S15. The drag reduction rates for the common Al surface with bubble supply, 9 RPs with bubble supply, and 9 SBPs with bubble supply were 4.5%, 12.7%, and 17.5%, respectively. The higher drag reduction rate for the 9 SBPs with bubble supply resulted from the spontaneous fast-moving air film, which offered a heterogeneous slip boundary to reduce the drag experienced by water flowing on it.52 Moreover, increasing the number of SBPs can increase the coverage of spontaneous fast-moving air film over the whole substrate surface. Under the condition of maintaining the presence of a spontaneous fast-moving air film, the maximum number of SBPs for the sample with 40 mm width and 200 mm length was 13, and the corresponding maximum drag reduction rate was as high as 27% (Figure S16†).
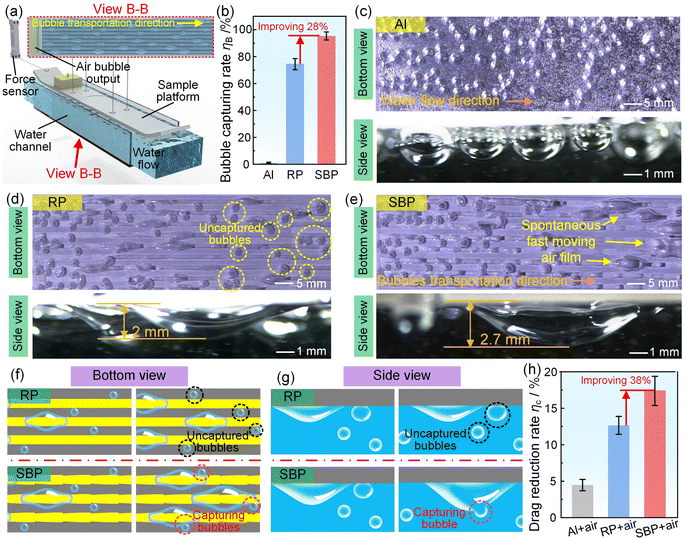 |
| Fig. 3 The SBP has superb underwater drag reduction capability. (a) Schematic of the underwater drag reduction experimental system. (b) Bubble capture rate of the common Al surface, RP, and SBP. (c) Images of the bubbles on the common Al surface with a bottom view and a side view. (d) Images of the bubbles on the RP with a bottom view and a side view. (e) Images of the bubbles on the SBP with a bottom view and a side view. (f) Schematic of bubbles on the RP and SBP with a bottom view. (g) Schematic of bubbles on the RP and SBP with a side view. (h) Drag reduction rate of the common Al surface with bubble supply, RP with bubble supply, and SBP with bubble supply. The water flow rate and air bubble flux were 0.5 m s−1 and 0.4 L min−1, respectively. | |
3.3 Marine antifouling
The marine fouling of the surface of a ship's hull first needs primary colonizers, such as diatoms or bacteria, to adhere on the surface, and then, other marine organisms use these primary colonizers as food to grow and breed. If the spontaneous fast-moving air film can prevent the attachment of primary colonizers, antifouling is expected to be realized. We first conducted an anti-diatom adhesion test using the experimental system shown in Fig. 4(a). We placed four samples, the common Al surface, superhydrophobic Al surface, the SBP without bubble supply, and the SBP with bubble supply in water containing diatoms. After 8 days of immersion, the common Al surface was thickly covered with many diatoms (Fig. 4(b), (b1)), and the superhydrophobic surface and SBP without bubble supply were thinly covered with some diatoms. However, the SBP with bubble supply remained clean without any diatom coverage (Fig. 4(b2)). In addition, we calculated the diatom coverage rate with immersion time for the different samples. The diatom coverage rate for the common Al surface, superhydrophobic Al surface, and SBP without bubble supply grew very fast and exceeded 90% for 4 immersion days and exceeded 97% for 8 immersion days (Fig. 4(c)). However, the diatom coverage rate for the SBP with bubble supply after 8 immersion days was almost 0, showing a superb anti-diatom adhesion capability. To further investigate the marine antifouling capability of the SBP with bubble supply, we then conducted an antifouling test in the simulated marine environment, including seawater, sea fish, and marine plants, as shown in Fig. 4(d). After 4 days of immersion, the whole common Al surface was corroded by the seawater and contaminated by the marine organisms (Fig. 4(e) and S17†). The SBP without bubble supply was also contaminated by the marine organisms. Chemical analysis indicated that the contaminant could be corrosion products, microorganisms, marine plant debris, and fish faeces (Figures S18 and S19†). However, the macroscopic and microscopic morphology of the SBP with bubble supply remained unchanged, indicating that the primary colonizers in the seawater could not adhere to the surface, indirectly preventing the adhesion of other marine organisms. It is worth noting that the exterior surrounding region of the SBP with bubble supply is still easily fouled. Similarly, to maintain the presence of a spontaneous fast-moving air film, for the sample with 40 mm width and 200 mm length, the maximum number of SBPs was 13, and the corresponding maximum marine antifouling rate was as high as 80% (Figure S16†).
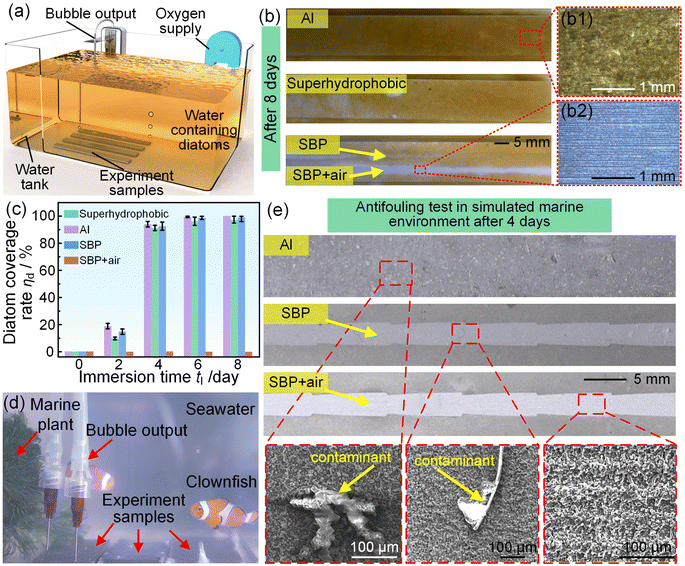 |
| Fig. 4 The SBP has excellent marine antifouling capability. (a) Schematic of an anti-diatom adhesion test device. (b) Images of the different samples after the anti-diatom adhesion test for 8 days. (b1) Enlarged view of the common Al surface. (b2) Enlarged view of the SBP with bubble supply. (c) Diatom coverage rate for the common Al surface, superhydrophobic surface, SBP without bubble supply, and SBP with bubble supply at different immersion times. (d) Image of the antifouling test in the simulated marine environment. (e) Images and SEM images of the common Al surface, SBP without bubble supply, and SBP with bubble supply after the antifouling test for 4 days. The air bubble flux for the SBP when the air supply was 0.4 L min−1. | |
4 Conclusions
In conclusion, a spontaneous fast-moving air film, which originated from the spontaneous and directional transportation of underwater air bubbles on a serial brachistochrone-shaped pattern (SBP), was proposed for drag reduction and antifouling. We first investigated the variation of the underwater air bubble transportation processes with the SBP parameters. The experimental results and theoretical analysis showed that the decreasing rolling circle radius r, increasing start rotation angle θ1, decreasing end rotation angle θ2, and increasing ratio of the narrow width to the wide width k led to an increase in the junction number. In addition, the increasing r, decreasing θ1, and increasing θ2 resulted in an increased SBP area. However, the increasing number of junctions and increasing SBP area would result in a decreased transportation velocity. Moreover, the decreasing k even meant that the air bubble could not be transported over a long distance. We then obtained the fastest transportation velocity of 444 mm s−1 after the Box–Behnken design optimization and junction optimization strategy, which was faster than the existing underwater spontaneous bubble transportation method. Since the air bubble could be quickly transported on the SBP, the supplied underwater air bubbles could form a spontaneous fast-moving air film, which offered a heterogeneous slip boundary to reduce the drag experienced by water flowing on it. In addition, the spontaneous fast-moving air film could prevent the attachment of primary colonizers and achieve antifouling. Eventually, under the condition of maintaining the presence of a spontaneous fast-moving air film, we simultaneously achieved 27% underwater drag reduction and 80% marine antifouling by setting a suitable number of SBPs. This finding could maximize energy saving for ships and facilitate sustainable development for humans.
Data availability
The data that supports the findings of this study are available in the ESI† of this article.
Author contributions
D. Yan and J. Song conceived the research. J. Lin, B. Zhang, S. Zhang and D. Yan performed the experiments. D. Yan and J. Song analysed the mechanism. D. Yan and J. Song wrote the original manuscript. S. Ling and J. Song revised it. All authors discussed the results and commented on the manuscript.
Conflicts of interest
There are no conflicts to declare.
Acknowledgements
This project was financially supported by the National Natural Science Foundation of China (NSFC, 52175380), Outstanding Youth Natural Science Foundation of Liaoning Province (2023JH3/10200013), Aviation Science Fund (2023Z045063001), Liao Ning Revitalization Talents Program (XLYC2203115), and Fundamental Research Funds for the Central Universities (DUT23YG118).
References
- A. Goldthau and S. Tagliapietra, Nature, 2022, 612, 627–630 CrossRef CAS PubMed.
- Z. Sun, Y. Liao, S. Zhao, X. Zhang, Q. Liu and X. Shi, J. Mater. Chem. A, 2022, 10, 5174–5211 RSC.
- X. Wang, Z. Li, X. Luo, S. Chang, H. Zhu, X. Guan and S. Wang, MRS Energy Sustain., 2023, 10, 247–260 CrossRef.
- K. W. Yanuar, S. Pratama, B. Candra and B. Rahmat, J. Mar. Sci. Appl., 2018, 17, 165–172 CrossRef.
- T. Wu, W. Chen, A. Zhao, P. He and H. Chen, Ocean Eng., 2020, 218, 107902 CrossRef.
- S. Amini, S. Kolle, L. Petrone, O. Ahanotu, S. Sunny, C. Sutanto, S. Hoon, L. Cohen, J. Weaver, J. Aizenberg, N. Vogel and A. Miserez, Science, 2017, 357, 668 CrossRef CAS PubMed.
- J. Tan, J. Xu, D. Wang, J. Yang and S. Zhou, J. Mater. Chem. A, 2020, 8, 24086–24097 RSC.
- W. Zhao, J. Yang, H. Guo, T. Xu, Q. Li, C. Wen, X. Sui, C. Lin, J. Zhang and L. Zhang, Chem. Eng. Sci., 2019, 207, 790–798 CrossRef CAS.
- H. Jin, L. Tian, W. Bing, J. Zhao and L. Ren, Prog. Mater. Sci., 2022, 124, 1008898 CrossRef.
- I. Vakarelski, E. Klaseboer, A. Jetly, M. Mansoor, A. Aguirre-Pablo, D. Chan and S. Thoroddsen, Sci. Adv., 2017, 3, e1701558 CrossRef PubMed.
- K. Choi, Nature, 2006, 440, 754 CrossRef CAS PubMed.
- W. Jo, H. Kang, J. Choi, J. Jung, J. Hyun, J. Kwon, I. Kim, H. Lee and H. Kim, Nano Lett., 2021, 21, 5500–5507 CrossRef CAS PubMed.
- G. Bixler and B. Bhushan, Adv. Funct. Mater., 2013, 23, 4507–4528 CrossRef CAS.
- S. Wu, C. Hsu and T. Lin, Ocean Eng., 2007, 83–93 CrossRef.
- S. Ceccio, Annu. Rev. Fluid. Mech., 2010, 42, 183–203 CrossRef.
- I. Kumagai, Y. Takahashi and Y. Murai, Ocean Eng., 2015, 95, 183–194 CrossRef.
- D. Saranadhi, D. Chen, J. Kleingartner, S. Srinivasan, R. Cohen and G. McKinley, Sci. Adv., 2016, 2, e1600686 CrossRef PubMed.
- I. Vakarelski, N. Patankar, J. Marston, D. Chan and S. Thoroddsen, Nature, 2012, 489, 274–277 CrossRef CAS PubMed.
- U. Gunatilake, Y. Alvarez-Brana, E. Ojeda, L. Basabe-Desmonts and F. Benito-Lopez, J. Mater. Chem. A, 2022, 10, 12832–12841 RSC.
- X. Bai, X. Gou, J. Zhang, J. Liang, L. Yang, S. Wang, X. Hou and F. Chen, Small, 2023, 19, 2206463 CrossRef CAS PubMed.
- C. Lee and C. Kim, Phys. Rev. Lett., 2011, 106, 14502 CrossRef PubMed.
- J. Wei, J. Zhang, X. Cao, J. Huo, X. Huang and J. Zhang, Nat. Commun., 2023, 14, 2862 CrossRef CAS PubMed.
- H. Hu, J. Wen, L. Bao, L. Jia, D. Song, B. Song, G. Pan, M. Scaraggi, D. Dini, Q. Xue and F. Zhou, Sci. Adv., 2017, 3, e1603288 CrossRef PubMed.
- W. Barthlott, T. Schimmel, S. Wiersch, K. Koch, M. Brede, M. Barczewski, S. Walheim, A. Wei, A. Kaltenmaier, A. Leder and H. Bohn, Adv. Mater., 2010, 22, 2325–2328 CrossRef CAS PubMed.
- L. Wang, D. Li, G. Jiang, X. Hu, R. Peng, Z. Song, H. Zhang, P. Fan and M. Zhong, ACS Nano, 2024, 18, 12489–12502 CrossRef CAS PubMed.
- J. Busch, W. Barthlott, M. Brede, W. Terlau and M. Mail, Philos. Trans. R. Soc. London, A, 2019, 377, 20180263 CrossRef CAS PubMed.
- O. Ferreira, P. Rijo, J. Gomes, R. Santos, S. Monteiro, c. Vilas-Boas, M. Correia, s. Almada, L. Alves, J. Bordado and E. Silva, ACS Sustainable Chem. Eng., 2020, 8, 12–17 CrossRef CAS.
- J. Choi, S. Lee, K. Ohkawa and D. Hwang, ACS Nano, 2021, 18566–18579 CrossRef CAS PubMed.
- I. Banerjee, R. Pangule and R. Kane, Adv. Mater., 2011, 23, 690–718 CrossRef CAS PubMed.
- Y. Wang, L. Zhang, W. Du, X. Zhou, Y. Guo, S. Zhao, D. Zhang and H. Chen, Adv. Funct. Mater., 2023, 33, 85–89 Search PubMed.
- X. Xue, C. Yu, J. Wang and L. Jiang, ACS Nano, 2016, 10, 10887–10893 CrossRef CAS PubMed.
- X. Gao, F. Zhang, Z. Zhang, Z. Wang, Y. Song, G. Cheng and J. Ding, ACS Appl. Mater. Interfaces, 2023, 15, 54119–54128 CrossRef CAS PubMed.
- C. Yu, M. Cao, Z. Dong, K. Li, C. Yu, J. Wang and L. Jiang, Adv. Funct. Mater., 2016, 26, 6830–6835 CrossRef CAS.
- X. Wang, H. Bai, J. Yang, Z. Li, Y. Wu, C. Yu, L. Jiang and M. Cao, Small, 2021, 17 Search PubMed 2007803.
- X. Wang, H. Bai, Z. Li, Y. Tian, T. Zhao and M. Cao, Mater. Horiz., 2023, 10, 3351–3359 RSC.
- C. Yu, X. Zhu, K. Li, M. Cao and L. Jiang, Adv. Funct. Mater., 2017, 27, 1701605 CrossRef.
- K. Zhuang, X. Yang, W. Huang, Q. Dai and X. Wang, ACS Appl. Mater. Interfaces, 2021, 13, 61780–61788 CrossRef CAS PubMed.
- Z. Wang, J. Xiong, Y. Liao, M. Xie, D. Yang, C. Zhang, Y. Chen and Z. Zou, Chem. Eng. J., 2023, 474, 145352 CrossRef CAS.
- J. Yong, Q. Yang, J. Huo, X. Hou and F. Chen, Int. J. Extrem. Manuf., 2022, 4, 15002 CrossRef.
- D. Xie, Y. Sun, Y. Wu, K. Wang, G. Wang, F. Zang and G. Ding, Adv. Mater., 2023, 35, e2208645 CrossRef PubMed.
- X. Xiao, S. Li, X. Zhu, C. Zhang, F. Jiang, C. Yu and L. Jiang, Nano Lett., 2021, 21, 2117–2123 CrossRef CAS PubMed.
- Z. Liu, H. Zhang, Y. Han, L. Huang, Y. Chen, J. Liu, X. Wang, X. Liu and S. Ling, ACS Appl. Mater. Interfaces, 2019, 11, 23808–23814 CrossRef CAS PubMed.
- H. Ma, M. Cao, C. Zhang, Z. Bei, K. Li, C. Yu and L. Jiang, Adv. Funct. Mater., 2018, 28, 1705091 CrossRef.
- J. Song, Z. Liu, X. Wang, H. Liu, Y. Lu, X. Deng, C. J. Carmalt and I. P. Parkin, J. Mater. Chem. A, 2019, 7, 13567–13576 RSC.
- H. Chen, T. Ran, Y. Gan, J. Zhou, Y. Zhang, L. Zhang, D. Zhang and L. Jiang, Nat. Mater., 2018, 17, 935–942 CrossRef CAS PubMed.
- J. Zhang, A. Wang and S. Seeger, Adv. Funct. Mater., 2013, 24, 1074–1080 CrossRef.
- H. Chen, P. Zhang, L. Zhang, H. L. Iu, Y. Jiang, D. Zhang, Z. Han and L. Jiang, Nature, 2016, 532, 85–89 CrossRef CAS PubMed.
- E. Rodgers, Am. J. Phys., 1946, 14, 249–252 CrossRef.
- D. Yan, Y. Chen, J. Liu and J. Song, Small, 2023, 19, 2301745 CrossRef CAS PubMed.
- Y. Wang, Y. Sun, Y. Sun, X. Sui, B. Yuan, Y. Wang and W. Liang, Prog. Org. Coat., 2024, 188, 108167 CrossRef CAS.
- D. Yan, Y. Lu, J. Liu, Y. Chen, J. Sun and J. Song, Nanoscale, 2023, 15, 11473–11481 RSC.
- R. A. Verschoof, R. C. A. van der Veen, C. Sun and D. Lohse, Phys. Rev. Lett., 2016, 117, 104502 CrossRef PubMed.
|
This journal is © The Royal Society of Chemistry 2024 |
Click here to see how this site uses Cookies. View our privacy policy here.